DOI:
10.1039/D3QI02107F
(Research Article)
Inorg. Chem. Front., 2024,
11, 107-113
Na10Zn(NO3)4(SO3S)4: a nonlinear optical crystal combining inorganic π-conjugated and non-π-conjugated heteroanion groups†
Received
14th October 2023
, Accepted 7th November 2023
First published on 7th November 2023
Abstract
The research on nonlinear optical (NLO) crystals is mainly focused on two relatively separate systems, π-conjugated or non-π-conjugated systems, respectively. In this work, we report a new NLO crystal Na10Zn(NO3)4(SO3S)4 combining inorganic π-conjugated [NO3] and non-π-conjugated heteroanion [S2O3] groups, which was synthesized by the aqueous solution method. In the structure, the similar coexistence of planar triangular anionic groups (π-conjugated) and heteroanion tetrahedra (non-π-conjugated) is rarely mentioned, which provides a new approach for designing NLO crystals. Notably, this compound exhibits a phase-matching second-harmonic generation (SHG) response 1.2 times that of KH2PO4. In addition, birefringence, absorption edge and theoretical calculations are also reported in this article. This work provides a new designing route to expand NLO systems via combining planar π-conjugated and non-π-conjugated heteroanion groups.
Introduction
Nonlinear optical (NLO) crystals have emerged as the core materials of all-solid-state lasers and are widely applied in diverse areas such as high-resolution photoelectron spectroscopy, laser communication, laser prototyping and more.1–5 Over the past few decades, researchers have displayed persistent research interest in exploring ultraviolet (UV) and deep-UV NLO crystals that exhibit excellent comprehensive properties. Generally, these properties including the second-harmonic generation (SHG) effect, birefringence and band gap are closely related to the structure of crystals.6 Traditionally, inorganic π-conjugated groups, such as [BO3],7 [CO3],8 and [NO3],9 have been the primary sources for designing and synthesizing high-performance NLO crystals. Some examples include borates (KBe2BO3F2,10 Li4Sr(BO3)2,11 and Sr2Be2B2O7
12), carbonates (Ca2Na3(CO3)3F),13 and nitrates (Ba2NO3(OH)3).14 These groups possess delocalized π-electron structures, enabling NLO crystals to exhibit strong SHG effects and birefringence.
The inorganic non-π-conjugated groups (such as [SO4],15 [PO4],16 and [SiO4]
17) have received limited attention as NLO materials due to their isotropic tetrahedral structure resulting in compounds with relatively weak birefringence and SHG effects. Until 2014, Ba3P3O10Cl,3 as a significant example, confirmed the superior UV transparency properties of NLO crystals constructed with non-π-conjugated groups. This discovery has triggered widespread attention and investigation into non-π-conjugated systems. Subsequently, a series of phosphates, including Ba5P6O20,18 Cs2LiPO4,19,20 and Ba2NaClP2O7,21 as well as sulphates NH4NaLi2(SO4)2
22 and silicates Rb6Si10O23,23 have been reported for deep-UV NLO crystals. Furthermore, new crystals with significant potential in the field of nonlinear optics have been successfully developed, such as K2Sb(P2O7)F,24 LiHgPO4,25 [Ag(NH3)2]2SO4,26 CeF2SO4
27 and K2Mn3(SO4)3F2·4H2O.28 Recently, researchers have proposed design strategies to improve the birefringence and NLO properties of non-π-conjugated systems.29 One of these strategies involves the partial substitution of anions in tetrahedral units to form heteroanion groups like BOxF4−x (x = 1, 2, 3),30 POxF4−x (x = 1, 2, 3),31 SOxF4−x (x = 1, 2, 3),32 and SiOxF6−x (x = 1, 2, 3, 4, 5).33 Indeed, these non-π-conjugated heteroanion groups result in large birefringence and NLO coefficients of compounds like NH4B4O6F,34 (NH4)2PO3F,31,35 NaNH4PO3F·H2O,35,36 C(NH2)3SO3F
37 and so on. Recently, several novel compounds with partial sulfur-substituted groups have been discovered, such as Na3PO3S,38 Na10Cd(NO3)4(SO3S)4,39 (C(NH2)3)SO3S,40 and [C(NH2)3]2S2O8.41 These new partially substituted non-π-conjugated groups exhibit enhanced microscopic properties compared to normal oxygen tetrahedral units (MO4, where M = B, P, S, Si, etc.).
Therefore, both π-conjugated and non-π-conjugated groups have their own structural advantages and have the potential to construct NLO crystals with excellent performance. However, inorganic π-conjugated and non-π-conjugated systems were considered as two relatively separate research subjects in previous studies. The research on NLO crystals combining both inorganic π-conjugated and non-π-conjugated groups has been relatively limited. Recently, Chen's team proposed the π-conjugated confinement principle, which states that separating the non-π-conjugated [PO4] groups successfully decreases the π-conjugation interaction between [CO3] units, thereby establishing a connection between π-conjugated and non-π-conjugated groups. Under this guidance, we propose a rational design strategy that combines π-conjugated and non-π conjugated groups to obtain NLO crystals. Thus, we successfully synthesized Na10Zn(NO3)4(SO3S)4 (1) which combining the π-conjugated [NO3] and non-π-conjugated heteroanion [SO3S] groups. It exhibits a significant SHG effect 1.2 times that of KDP and a short UV absorption edge of 240 nm. The synthesis, elemental analysis, crystal structure, optical properties and theoretical calculations of this compound are also reported in this work.
Experimental section
Reagents and synthesis
All the reagents including Na2S2O3 (Tansoole, 99.0%) and Zn(NO3)2·6H2O (Aladdin, 99.0%) are of analytical grade obtained from commercial sources without further purification. In this experiment, we obtained the crystals of 1 by a facile aqueous solution method. A mixture of Na2S2O3 (0.632 g, 4 mmol) and Zn(NO3)2·6H2O (0.297 g, 1 mmol) was dissolved in 10 ml deionized water, and this solution needed heating and stirring for about 30 min until it became clear. Then this solution was allowed to evaporate slowly at room temperature. After several days, colourless single crystals of 1 were obtained.
Single crystal X-ray diffraction
A transparent crystal of compound 1, measuring 0.08 × 0.03 × 0.03 mm3, was carefully chosen under an optical microscope for single-crystal X-ray diffraction (XRD) analysis. The diffraction data were acquired at a temperature of 293(2) K using graphite-monochromatized Cu Kα radiation (λ = 1.54178 Å) on a Rigaku XtaLAB Synergy diffractometer equipped with a HyPix detector. The crystal structure of 1 was successfully determined using the OLEX2
42 program. The comprehensive details regarding the crystallographic data and the results of the structural refinement for compound 1 can be found in Table S1.† In addition, Tables S2–S5† present the atomic coordinates and corresponding equivalent isotropic displacement parameters, selected bond lengths and angles, as well as the anisotropic displacement parameters (Å2 × 103) for compound 1.
Powder X-ray diffraction
Powder X-ray diffraction (PXRD) measurements were performed for pure materials using a Miniflex600 powder X-ray diffractometer equipped with Cu Kα radiation (λ = 1.5418 Å). The diffraction angle was scanned within the range of 5° to 75° with a step width of 0.02°. As depicted in Fig. 1, the experimental X-ray powder patterns of 1 exhibit a high degree of agreement with the values simulated from single-crystal XRD analysis, confirming the purity of 1.
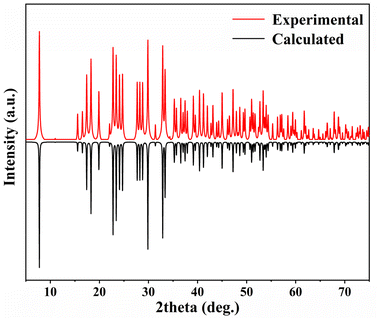 |
| Fig. 1 Experimental and calculated XRD patterns of 1. | |
Elemental analysis
For the elemental analysis of compound 1, a semiquantitative microprobe was employed in conjunction with a field emission scanning electron microscope (FESEM, SU-80100) that was equipped with an energy dispersive X-ray spectroscope. The energy dispersive spectra were obtained by carefully selecting clean surfaces of the sample for measurement. The presence of Na, Zn, N, O, and S elements of compound 1 was effectively confirmed by energy dispersive X-ray spectra (EDS), as depicted in Fig. S1.† Furthermore, the EDS mapping presented in Fig. 2 illustrates the spatial distribution of these elements.
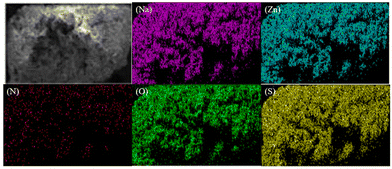 |
| Fig. 2 EDS mapping of sodium (purple), zinc (blue), nitrogen (red), oxygen (green) and sulfur (yellow) on the 1 crystal (top left). The scale bar is 100 μm. | |
UV-vis-NIR diffuse reflectance spectroscopy
A PerkinElmer Lambda-950 UV/vis/NIR spectrophotometer was used to obtain UV-vis-near-infrared (NIR) diffuse reflection data. The measurements were performed at room temperature within a scan range of 200–800 nm. During the measurement process, BaSO4 powder was used as the standard with 100% reflectance. The recorded data provide information on the diffuse reflection properties of the sample across the specified wavelength range.
SHG measurements
The investigation of the polycrystalline SHG signals of sample 1 was performed using the Kurtz–Perry method.43 Measurements were conducted using a Q-switched Nd:YAG solid-state laser with a fundamental wavelength of 1064 nm. The crystals 1 were ground and screened into different particle size ranges, namely 45–53 μm, 53–75 μm, 75–105 μm, 105–150 μm, 150–210 μm, and 210–300 μm. Similarly, KH2PO4 (KDP) crystals were also processed following the same procedures as that for a reference material. Subsequently, both the samples were placed inside a black box and exposed to pulsed laser irradiation with a wavelength of 1064 nm, allowing further analysis.
Birefringence measurements
To measure the birefringence of the crystal, the interference colour method was employed using a polarizing microscope (ZEISS Axio Scope.A1) equipped with a Berek compensator at a wavelength of 550 nm. The birefringence index (Δn) was calculated using the formula R = Δn × d, where R represents the optical path difference obtained using the polarizing microscope, and d represents the crystal thickness measured using the same microscope.
Theoretical calculations
Theoretical calculations based on density functional theory (DFT)44,45 were performed using the CASTEP package. The exchange–correlation energy was described using the functional developed by Perdew–Burke–Ernzerhof within the generalized gradient approximation (GGA) form. A plane wave cut-off energy of 800 eV was set as the basic cut-off energy. For the numerical integration of the Brillouin zone, the Monkhorst–Pack scheme with a grid density of 3 × 3 × 1 at the k-point was employed for sample 1.
Results and discussion
Crystal structure
Compound 1 crystallizes in a non-centrosymmetric tetragonal space group of P
(no. 81) with unit cell parameters of a = 11.279(2), b = 11.279(2), c = 5.392(2) Å, α = β = γ = 90°, and Z = 1. The unit cell contains one Zn atom, one N atom, two S atoms, three Na atoms and six O atoms. As shown in Fig. 3a, each N atom coordinates with three O atoms to form a π-conjugated [NO3] group. The distance of the N–O bond ranges from 1.248(3) to 1.253(3) Å. Two sulfur atoms are present in different oxidation states: −2 and +6, respectively. Each S6+ atom, serving as the central atom in a tetrahedron, coordinates with three oxygen atoms and one S2− atom to form a non-π-conjugated heteroanion [SO3S] group (Fig. 3b). Meanwhile, the S2− atom forms an S
S double bond with a bond length of 2.027(7) Å. Based on the
symmetry operation, four heteroanion [SO3S] groups further connect with the central Zn atom which is located at the face-centered (the center of the ab plane) position of the unit cell through their S2− atoms, forming an isolated Zn(SO3S)4 moiety (Fig. 3c). The π-conjugated [NO3] groups dispersed between each [SO3S] moiety through four-fold rotational reflection symmetry operations. Meanwhile, the Na+ cation serves as a charge-balancing cation filled in gaps. To further identify the S
S bond in 1, the Raman spectrum is shown in Fig. S2.† The peak at 476 cm−1 presents the symmetrical stretching mode of the S
S bond.46 In addition, for the growth of large-sized single crystals, we made preliminary attempts and provided a photograph of the crystal (refer to Fig. S3†).
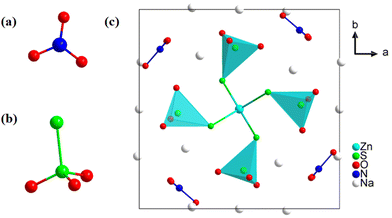 |
| Fig. 3 (a) π-Conjugated [NO3] group. (b) Non-π-conjugated heteroanion [SO3S] group; (c) the crystal structure of 1 viewed along the c axis. | |
UV-vis-NIR diffuse reflectance spectra
As illustrated in Fig. 4, the UV-vis-NIR diffuse reflection spectrum of 1 shows that its reflectance is about 10% at a wavelength of 240 nm. Based on Kubelka–Munk function,47 the reflectance can be used to calculate the experimental energy gap. By applying this approach, the experimental energy gap of 1 was deduced to be 4.02 eV, thus indicating its potential applications in the UV spectral range.
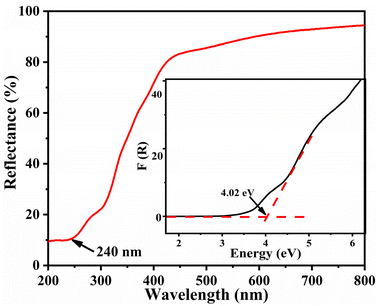 |
| Fig. 4 UV-visible-NIR diffuse reflectance spectrum of 1. | |
NLO properties
The powder SHG response of crystal 1 was measured under 1064 nm laser irradiation using the Kurtz–Perry method,48 and KDP crystals used as a reference. As indicated in Fig. 5a, the SHG intensity will increase simultaneously with the increasing particle size until it reaches a stable value, which suggests that compound 1 is phase matchable. Specifically, with the particle size of the powder in the range of 210–300 μm, the intensity of the SHG signal for compound 1 is approximately 1.2 times that of the KDP (Fig. 5b). The SHG effect of 1 was superior to those of some sulphates such as Cs2Zn2(SO4)3 (0.15 × KDP),15 Cs2Ca2(SO4)3 (0.6 × KDP),49 K2Zn3(SO4)(HSO4)2F4 (0.3 × KDP),50 (NH4)2Na3Li9(SO4)7 (0.5 × KDP),22 KTb(SO4)2 (0.3 × KDP),51 and Li8NaRb3(SO4)6·2H2O (0.3 × KDP).52 In addition, compared to other NLO materials containing inorganic π-conjugated and non-π-conjugated groups, compound 1 exhibits comparable or even better SHG effects. Examples of such compounds include Ba4(BO3)3(SiO4)Cl·Ba3X (X = Cl, Br) (1 × KDP),17 Ba3Ca4(BO3)3(SiO4)Cl (0.6 × KDP),53 (NH4)2B4SO10 (1.1 × KDP)54 and so on. Therefore, we can draw a conclusion that compound 1 exhibits a significant SHG effect. It is worth noting that such NLO crystals combining inorganic π-conjugated and non-π-conjugated groups are still in their infancy; there is much unexplored space, indicating the need for further research in this field.
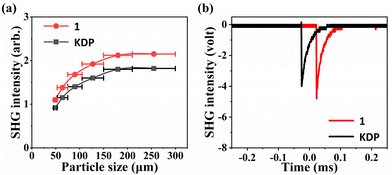 |
| Fig. 5 (a) SHG intensity vs. particle sizes at λ = 1064 nm for 1. (b) SHG signals of 1 as compared with the KDP reference. | |
Birefringence
The experimental birefringence of crystal 1 was determined by the method of detecting interference colours under a polarizing microscope. It was observed that crystal 1 exhibited interference effects under linearly polarized light (refer to Fig. 6a). Subsequently, by inserting a compensator and adjusting appropriately, complete compensation of the phase difference of light is achieved, resulting in the complete extinction of interference effects. In this case, crystal 1 achieved complete extinction, appearing black, which indicates its birefringent properties. Additionally, the optical path difference of the crystal was 306.3 nm according to the reading of the compensator. Using the polarizing microscope, further measurements determined that the thickness of crystal 1 is 23.02 μm (Fig. S5†). By plugging the above numerical value into the formula, the birefringence of crystal 1 is determined to be approximately 0.013 at a wavelength of 550 nm.
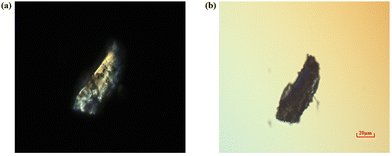 |
| Fig. 6 (a) Single crystal of 1 under the polarizing microscope. (b) Crystal achieves complete extinction under the Beret compensator. | |
In addition, the birefringence of crystal 1 at a wavelength of 550 nm, calculated using the first-principles method, is determined to be 0.017 (see Fig. 7). The consistency between the theoretical value and the experimental measurement indicates that the birefringence we obtained is reliable.
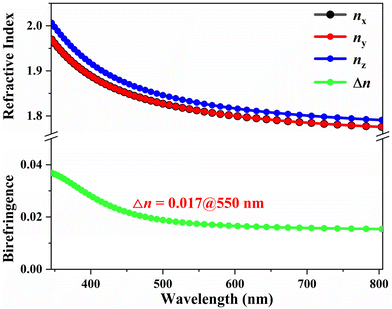 |
| Fig. 7 Calculated refractive index and birefringence of 1. | |
Theoretical calculations
To further gain a comprehensive understanding of the relationship between the structures and properties, we carried out theoretical calculations based on density functional theory. The electronic band structure analysis suggests that 1 has an indirect band gap of 2.59 eV (Fig. 8a), which is consistent with the experimental result based on the single crystal sample. In the investigation of the optical properties of materials, the focus is on analysing electron transitions that occur between the top of the valence band and the bottom of the conduction band. Therefore, Fig. 8b depicts the total density of states (DOS) and the partial DOS of 1, providing a visual representation of the distribution of electronic states for that specific element. At the top of the valence band, ranging from −5 to 0 eV, it is primarily occupied by S 3p (especially for S2−) and O 2p orbitals, where the S 3p orbitals originate from the non-π-conjugated [SO3S] groups. Conversely, the bottom of the conduction band is predominantly composed of N 2p and O 2p orbitals, where the N 2p orbitals originate from the π-conjugated [NO3] groups. It can be concluded that these optical properties of 1 are mainly determined by the π-conjugated [NO3] groups and the non-π-conjugated heteroanionic [SO3S] groups, and the contribution of Na and Zn cations can be neglected. It is necessary to point out that combining non-π-conjugated heteroanionic groups with π-conjugated groups is an effective strategy for obtaining compounds with excellent optical properties and its potential needs further exploration.
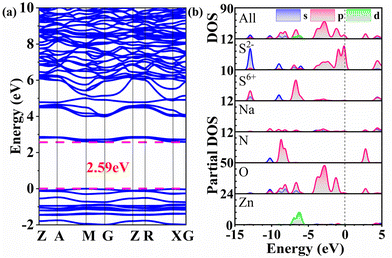 |
| Fig. 8 (a) Electronic band structure of 1. (b) DOS and partial DOS of 1. | |
Conclusions
In conclusion, we successfully synthesized a NLO crystal Na10Zn(NO3)4(SO3S)4via a facile water solution method. The single crystal structure analysis confirmed the presence of π-conjugated [NO3] groups and non-π-conjugated [SO3S] groups in this material, as further confirmed through EDS and Raman measurements. Experimental and calculation results demonstrate its excellent optical properties, including diffuse reflectance, SHG and birefringence. It is particularly noteworthy that this compound exhibits a significant SHG response, 1.2 times that of KDP. Theoretical calculations indicate that the synergistic interaction between the π-conjugated [NO3] groups and the non-π-conjugated heteroanionic [SO3S] groups makes the primary contribution. This finding provides new possibilities for designing novel NLO materials by combining different conjugated groups such as π-conjugated and non-π-conjugated heteroanionic groups.
Author contributions
Junhua Luo provided research ideas and supervised the entire research. Sangen Zhao was responsible for theoretical calculations. Zihao Yu completed the entire experimental work. Yuhang Jiang and Weiqi Huang assisted in the experiments. Changsheng Yang assisted in the PXRD measurements. Qingran Ding wrote the initial draft and revised the manuscript. All authors participated in the discussion.
Conflicts of interest
There are no conflicts to declare.
Acknowledgements
This work was financially supported by the National Natural Science Foundation of China (22201110, 22193042, 21833010, 22125110, 22122507, 21921001 and U21A2069), the Key Research Program of Frontier Sciences of the Chinese Academy of Sciences (ZDBS-LY-SLH024), the Natural Science Foundation of Fujian Province (2021J01523 and 2020J01112), the Youth Innovation Promotion of CAS (2019301, Y202069 and 2020307), Young Talent Supporting Project of Fujian Association of Science and Technology (2021000008), the National Key Research and Development Program of China (2019YFA0210402), and the Jiangxi Provincial Natural Science Foundation (20232BAB213023).
Notes and references
- D. F. Eaton, Nonlinear optical materials, Science, 1991, 253, 281–287 CrossRef CAS.
- D. Cyranoski, China's crystal cache: a Chinese laboratory is the only source of a valuable crystal. David Cyranoski investigates why it won't share its supplies, Nature, 2009, 457, 953–956 CrossRef CAS.
- P. Yu, L. M. Wu, L. J. Zhou and L. Chen, Deep-Ultraviolet Nonlinear Optical Crystals: Ba3P3O10X (X = Cl, Br), J. Am. Chem. Soc., 2014, 136, 480–487 CrossRef CAS.
- Y. N. Xia, C. T. Chen, D. Y. Tang and B. C. Wu, New nonlinear optical crystals for UV and VUV harmonic generation, Adv. Mater., 1995, 7, 79–81 CrossRef CAS.
- H. W. Huang, L. J. Liu, S. F. Jin, W. J. Yao, Y. H. Zhang and C. T. Chen, Deep-Ultraviolet Nonlinear Optical Materials: Na2Be4B4O11 and LiNa5Be12B12O33, J. Am. Chem. Soc., 2013, 135, 18319–18322 CrossRef CAS.
- J. Chen, Q. Q. Chen, F. F. Mao, Z. Liu, B. X. Li, X. H. Wu and K. Z. Du, Ba4Ag5(IO3)6(I3O8)3(I4O11)2:A Nonlinear Optical Crystal Containing Two Types of Polyiodate Anions, Inorg. Chem. Front., 2022, 9, 5917–5925 RSC.
- M. Mutailipu, M. Zhang, H. P. Wu, Z. H. Yang, Y. H. Shen, J. L. Sun and S. L. Pan, Ba3Mg3(BO3)3F3 polymorphs with reversible phase transition and high performances as ultraviolet nonlinear optical materials, Nat. Commun., 2018, 9, 3089 CrossRef.
- X. M. Liu, L. Kang, P. F. Gong and Z. S. Lin, LiZn(OH)CO3: A Deep-Ultraviolet Nonlinear Optical Hydroxycarbonate Designed from a Diamond-like Structure, Angew. Chem., Int. Ed., 2021, 60, 13574–13578 CrossRef CAS.
- S. G. Zhao, Y. Yang, Y. G. Shen, B. Q. Zhao, L. N. Li, C. M. Ji, Z. Y. Wu, D. Q. Yuan, Z. S. Lin, M. C. Hong and J. H. Luo, Cooperation of Three Chromophores Generates the Water-Resistant Nitrate Nonlinear Optical Material Bi3TeO6OH(NO3)2, Angew. Chem., Int. Ed., 2017, 56, 540–544 CrossRef CAS.
- B. C. Wu, D. Y. Tang, N. Ye and C. T. Chen, Linear and nonlinear optical properties of the KBe2BO3F2 (KBBF) crystal, Opt. Mater., 1996, 5, 105–109 CrossRef CAS.
- S. G. Zhao, P. F. Gong, L. Bai, X. Xu, S. Q. Zhang, Z. H. Sun, Z. S. Lin, M. C. Hong, C. T. Chen and J. H. Luo, Beryllium-free Li4Sr(BO3)2 for deep-ultraviolet nonlinear optical applications, Nat. Commun., 2014, 5, 4019 CrossRef CAS.
- C. T. Chen, Y. B. Wang, B. C. Wu, K. C. Wu, W. L. Zeng and L. H. Yu, Design and synthesis of an ultraviolet-transparent nonlinear optical crystal Sr2Be2B2O7, Nature, 1995, 373, 322–324 CrossRef CAS.
- M. Luo, Y. X. Song, C. S. Lin, N. Ye, W. D. Cheng and X. F. Long, Molecular Engineering as an Approach To Design a New Beryllium-Free Fluoride Carbonate as a Deep-Ultraviolet Nonlinear Optical Material, Chem. Mater., 2016, 28, 2301–2307 CrossRef CAS.
- X. H. Dong, L. Huang, Q. Y. Liu, H. M. Zeng, Z. E. Lin, D. G. Xu and G. H. Zou, Perfect balance harmony in Ba2NO3(OH)3: a beryllium-free nitrate as a UV nonlinear optical material, Chem. Commun., 2018, 54, 5792–5795 RSC.
- Y. C. Liu, Y. Q. Li, Y. Zhou, Q. R. Ding, Y. X. Chen, S. G. Zhao and J. H. Luo, A new nonlinear optical sulfate of layered structure: Cs2Zn2(SO4)3, Inorg. Chem. Commun., 2021, 124, 108390 CrossRef CAS.
- L. Xiong, L. M. Wu and L. Chen, A General Principle for DUV NLO Materials: π-Conjugated Confinement Enlarges Band Gap, Angew. Chem., Int. Ed., 2021, 60, 25063–25067 CrossRef CAS.
- X. X. Lin, F. F. Zhang, S. L. Pan, H. W. Yu, F. Y. Zhang, X. Y. Dong, S. J. Han, L. Y. Dong, C. Y. Bai and Z. Wang, Ba4(BO3)3(SiO4)·Ba3X (X = Cl, Br): new salt-inclusion borosilicate halides as potential deep UV nonlinear optical materials, J. Mater. Chem. C, 2014, 2, 4257–4264 RSC.
- S. G. Zhao, P. F. Gong, S. Y. Luo, L. Bai, Z. S. Lin, Y. Y. Tang, Y. L. Zhou, M. C. Hong and J. H. Luo, Tailored Synthesis of a Nonlinear Optical Phosphate with a Short Absorption Edge, Angew. Chem., Int. Ed., 2015, 54, 4217–4221 CrossRef CAS.
- Y. G. Shen, Y. Yang, S. G. Zhao, B. Q. Zhao, Z. S. Lin, C. M. Ji, L. N. Li, P. Fu, M. C. Hong and J. H. Luo, Deep-Ultraviolet Transparent Cs2LiPO4 Exhibits an Unprecedented Second Harmonic Generation, Chem. Mater., 2016, 28, 7110–7116 CrossRef CAS.
- L. Li, Y. Wang, B. H. Lei, S. J. Han, Z. H. Yang, K. R. Poeppelmeier and S. L. Pan, A New Deep-Ultraviolet Transparent Orthophosphate LiCs2PO4 with Large Second Harmonic Generation Response, J. Am. Chem. Soc., 2016, 138, 9101–9104 CrossRef CAS.
- J. Chen, L. Xiong, L. Chen and L. M. Wu, Ba2NaClP2O7: Unprecedented Phase Matchability Induced by Symmetry Breaking and Its Unique Fresnoite-Type Structure, J. Am. Chem. Soc., 2018, 140, 14082–14086 CrossRef CAS.
- Y. Q. Li, F. Liang, S. G. Zhao, L. N. Li, Z. Y. Wu, Q. R. Ding, S. Liu, Z. S. Lin, M. C. Hong and J. H. Luo, Two Non-π-Conjugated Deep-UV Nonlinear Optical Sulfates, J. Am. Chem. Soc., 2019, 141, 3833–3837 CrossRef CAS.
- S. Z. Huang, Y. Yang, J. B. Chen, W. Q. Jin, S. C. Cheng, Z. H. Yang and S. L. Pan, “Removing Center”—An Effective Structure Design Strategy for Nonlinear Optical Crystals, Chem. Mater., 2022, 34, 2429–2438 CrossRef CAS.
- Y. L. Deng, L. Huang, X. H. Dong, L. Wang, K. M. Ok, H. M. Zeng, Z. E. Lin and G. H. Zou, K2Sb(P2O7)F: Cairo Pentagonal Layer with Bifunctional Genes Reveal Optical Performance, Angew. Chem., Int. Ed., 2020, 59, 21151–21156 CrossRef CAS.
- B. L. Wu, C. L. Hu, F. F. Mao, R. L. Tang and J. G. Mao, Highly Polarizable Hg2+ Induced a Strong Second Harmonic Generation Signal and Large Birefringence in LiHgPO4, J. Am. Chem. Soc., 2019, 141, 10188–10192 CrossRef CAS.
- Y. C. Yang, X. Liu, J. Lu, L. M. Wu and L. Chen, [Ag(NH3)2]2SO4: A Strategy for the Coordination of Cationic Moieties to Design Nonlinear Optical Materials, Angew. Chem., Int. Ed., 2021, 60, 21216–21220 CrossRef CAS.
- C. Wu, T. H. Wu, X. X. Jiang, Z. J. Wang, H. Y. Sha, L. Lin, Z. S. Lin, Z. P. Huang, X. F. Long, M. G. Humphrey and C. Zhang, Large Second-Harmonic Response and Giant Birefringence of CeF2(SO4) Induced by Highly Polarizable Polyhedra, J. Am. Chem. Soc., 2021, 143, 4138–4142 CrossRef CAS.
- Y. Zhou, Y. Q. Li, Q. R. Ding, Y. C. Liu, Y. X. Chen, X. T. Liu, X. Y. Huang, L. N. Li, S. G. Zhao and J. H. Luo, Noncentrosymmetric K2Mn3(SO4)3F2·4H2O and Rb2Mn3(SO4)3F2·2H2O with pseudo-KTP structures, Chin. Chem. Lett., 2021, 32, 263–265 CrossRef CAS.
- H. Y. Wu, C. L. Hu, M. B. Xu, Q. Q. Chen, N. Ma, X. Y. Huang, K. Z. Du and J. Chen, From H12C3N2CdI4 to H11C4N2CdI3: a highly polarizable CdNI3 tetrahedron induced a sharp enhancement of second harmonic generation response and birefringence, Chem. Sci., 2023, 14, 9533–9542 RSC.
- B. B. Zhang, G. Q. Shi, Z. H. Yang, F. F. Zhang and S. L. Pan, Fluorooxoborates: Beryllium-Free Deep-Ultraviolet Nonlinear Optical Materials without Layered Growth, Angew. Chem., Int. Ed., 2017, 56, 3916–3919 CrossRef CAS.
- B. B. Zhang, G. P. Han, Y. Wang, X. L. Chen, Z. H. Yang and S. L. Pan, Expanding Frontiers of Ultraviolet Nonlinear Optical Materials with Fluorophosphates, Chem. Mater., 2018, 30, 5397–5403 CrossRef CAS.
- W. Q. Jin, W. Y. Zhang, A. Tudi, L. Y. Wang, X. Zhou, Z. H. Yang and S. L. Pan, Fluorine-Driven Enhancement of Birefringence in the Fluorooxosulfate: A Deep Evaluation from a Joint Experimental and Computational Study, Adv. Sci., 2021, 8, 2003594 CrossRef CAS.
- Q. R. Ding, X. M. Liu, S. G. Zhao, Y. S. Wang, Y. Q. Li, L. N. Li, S. Liu, Z. S. Lin, M. C. Hong and J. H. Luo, Designing a Deep-UV Nonlinear Optical Fluorooxosilicophosphate, J. Am. Chem. Soc., 2020, 142, 6472–6476 CrossRef CAS.
- G. Q. Shi, Y. Wang, F. F. Zhang, B. B. Zhang, Z. H. Yang, X. L. Hou, S. L. Pan and K. R. Poeppelmeier, Finding the Next Deep-Ultraviolet Nonlinear Optical Material: NH4B4O6F, J. Am. Chem. Soc., 2017, 139, 10645–10648 CrossRef CAS.
- L. Xiong, J. Chen, J. Lu, C. Y. Pan and L. M. Wu, Monofluorophosphates: A New Source of Deep-Ultraviolet Nonlinear Optical Materials, Chem. Mater., 2018, 30, 7823–7830 CrossRef CAS.
- J. Lu, J. N. Yue, L. Xiong, W. K. Zhang, L. Chen and L. M. Wu, Uniform Alignment of Non-π-Conjugated Species Enhances Deep Ultraviolet Optical Nonlinearity, J. Am. Chem. Soc., 2019, 141, 8093–8097 CrossRef CAS.
- M. Luo, C. S. Lin, D. H. Lin and N. Ye, Rational Design of the Metal-Free KBe2BO3F2·(KBBF) Family Member C(NH2)3SO3F with Ultraviolet Optical Nonlinearity, Angew. Chem., Int. Ed., 2020, 59, 15978–15981 CrossRef CAS.
- X. Y. Zhang, L. Kang, P. F. Gong, Z. S. Lin and Y. C. Wu, Nonlinear Optical Oxythiophosphate Approaching the Good Balance with Wide Ultraviolet Transparency, Strong Second Harmonic Effect, and Large Birefringence, Angew. Chem., Int. Ed., 2021, 60, 6386–6390 CrossRef CAS.
- Y. C. Liu, Y. Q. Liu, Z. S. Lin, Y. Q. Li, Q. R. Ding, X. Chen, L. N. Li, S. G. Zhao, M. C. Hong and J. H. Luo, Nonpolar Na10Cd(NO3)4(SO3S)4 Exhibits a Large Second-Harmonic Generation, CCS Chem., 2022, 4, 526–531 CrossRef CAS.
- Y. C. Liu, X. M. Liu, Z. Y. Xiong, B. W. Liu, J. L. Xu, L. N. Li, S. G. Zhao, Z. S. Lin, M. C. Hong and J. H. Luo, 2D van der Waals Layered [C(NH2)3]2SO3S Exhibits Desirable UV Nonlinear-Optical Trade-Off, Inorg. Chem., 2021, 60, 14544–14549 CrossRef CAS.
- M. Zhang, B. B. Zhang, D. Q. Yang and Y. Wang, [C(NH2)3]2S2O8: combining delocalized and localized π-conjugate units to achieve strong optical anisotropy, Inorg. Chem. Front., 2022, 9, 6067–6074 RSC.
- O. V. Dolomanov, L. J. Bourhis, R. J. Gildea, J. A. K. Howard and H. Puschmann, OLEX2: a complete structure solution, refinement and analysis program, J. Appl. Crystallogr., 2009, 42, 339–341 CrossRef CAS.
- S. K. Kurtz and T. T. Perry, A Powder Technique for the Evaluation of Nonlinear Optical Materials, J. Appl. Phys., 1968, 39, 3798–3813 CrossRef CAS.
- W. Kohn, Nobel Lecture: Electronic structure of matter—wave functions and density functionals, Rev. Mod. Phys., 1999, 71, 1253–1266 CrossRef CAS.
- S. J. Clark, M. D. Segall, C. J. Pickard, P. J. Hasnip, M. I. J. Probert, K. Refson and M. C. Payne, First principles methods using CASTEP, Z. Kristallogr. - Cryst. Mater., 2005, 220, 567–570 CrossRef CAS.
- J. Z. Zhou, Y. Chu, J. J. Li and S. L. Pan, Ba2BS3Cl and Ba5B2S8Cl2: first alkaline-earth metal thioborate halides with [BS3] units, Chem. Commun., 2021, 57, 6440–6443 RSC.
- P. Kubelka and F. Munk, An article on optics of paint layers, Z. Tech. Phys., 1931, 12, 259–274 Search PubMed.
- M. R. Sun, G. L. Wang and J. Y. Yao, The Kurtz–Perry Powder Technique Revisited: A Study of the Effect of Reference Selection on Powder Second-Harmonic Generation Response, Molecules, 2023, 28, 1116 CrossRef CAS.
- Y. G. Shen, X. L. Xue, W. Y. Tu, Z. Q. Liu, R. W. Yan, H. Zhang and J. R. Jia, Synthesis, Crystal Structure, and Characterization of a Noncentrosymmetric Sulfate Cs2Ca2(SO4)3, Eur. J. Inorg. Chem., 2020, 854–858 CrossRef CAS.
- Y. Zhou, X. Y. Zhang, Z. Y. Xiong, X. F. Long, Y. Q. Li, Y. X. Chen, X. Chen, S. G. Zhao, Z. S. Lin and J. H. Luo, Non-π-Conjugated Deep-Ultraviolet Nonlinear Optical Crystal K2Zn3(SO4)(HSO4)2F4, J. Phys. Chem. Lett., 2021, 12, 8280–8284 CrossRef CAS.
- Q. Wu, C. Yang, J. Ma, F. Liang, C. L. Teng and Y. S. Du, Synthesis, Crystal Structure, and Nonlinear Optical Property of an Anhydrous Sulfate KTb(SO4)2, Inorg. Chem., 2021, 60, 15041–15047 CrossRef CAS.
- Y. Q. Li, S. G. Zhao, P. Shan, X. F. Li, Q. R. Ding, S. Liu, Z. Y. Wu, S. S. Wang, L. N. Li and J. H. Luo, Li8NaRb3(SO4)6·2H2O as a new sulfate deep-ultraviolet nonlinear optical material, J. Mater. Chem. C, 2018, 6, 12240–12244 RSC.
- Z. X. Lu, F. F. Zhang, A. Tudi, S. J. Yu, Z. H. Yang and S. L. Pan, Ba3Ca4(BO3)3(SiO4)Cl: a new non-centrosymmetric complex alkaline-earth metal borosilicate chloride with a deep-ultraviolet cut-off edge, Inorg. Chem. Front., 2019, 6, 2200–2208 RSC.
- Z. J. Li, W. Q. Jin, F. F. Zhang, Z. L. Chen, Z. H. Yang and S. L. Pan, Achieving Short-Wavelength Phase-Matching Second Harmonic Generation in Boron-Rich Borosulfate with Planar [BO3] Units, Angew. Chem., Int. Ed., 2022, 61, e202112844 CrossRef CAS.
Footnote |
† Electronic supplementary information (ESI) available: Thermal stability, elemental analysis, crystallographic data (Tables S1–S5), Raman spectrum, crystal photograph, and crystal thickness. CCDC 2293547 for Na10Zn(NO3)4(SO3S)4 (1). For ESI and crystallographic data in CIF or other electronic format see DOI: https://doi.org/10.1039/d3qi02107f |
|
This journal is © the Partner Organisations 2024 |