DOI:
10.1039/D3MH01078C
(Communication)
Mater. Horiz., 2024,
11, 184-195
Porous SnO2 nanosheets for room temperature ammonia sensing in extreme humidity†‡
Received
12th July 2023
, Accepted 26th October 2023
First published on 31st October 2023
Abstract
Gas sensors based on tin dioxide (SnO2) for the detection of ammonia (NH3) have become commercially available for environmental monitoring due to their reactive qualities when exposed to different gaseous pollutants. Nevertheless, their implementation in the medical field has been hindered by certain inherent drawbacks, such as needing to operate at high temperatures, lack of selectivity, unreliable operation under high-humidity conditions, and a lower detection limit. To counter these issues, this study created 2D nanosheets of SnO2 through an optimized solvothermal method. It was found that tuning the precursor solution's pH to either neutral or 14 led to aggregated or distributed, uniform-size nanosheets with a higher crystallinity, respectively. Remarkably, the SnO2 nanosheet sensor (SNS-14) displayed a much lower response to water molecules and specific reactivity to ammonia even when subjected to reducing and oxidizing agents at 25 °C due to the micropores and chemisorbed oxygen on the nanosheets. Furthermore, the SNS-14 was seen to have the highest sensitivity to ammonia at 100 ppm, with rapid response (8 s) and recovery times (55 s) even at a high relative humidity of 70%. Its theoretical detection limit was recorded to be 64 ppt, better than any of the earlier SnO2-based chemiresistive sensors. Its exceptional sensing abilities were credited to its optimal crystallinity, specific surface area, defects, chemisorbed oxygen, and porous structure. NH3-TPD measurements and computational simulations were employed to understand the ammonia interaction with atomistic details on the SnO2 nanosheet surface. A real time breath sensing experiment was simulated to test the efficacy of the sensor. Reaching this advancement is an achievement in bypassing past boundaries of SnO2-centered sensors, making it feasible to detect ammonia with enhanced precision, discrimination, dependability, and velocity for probable usages in medical diagnostics and ecological surveillance.
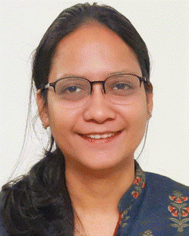
Ritu Gupta
| Congratulations to Materials Horizons, one of the most prestigious materials journals, on reaching its 10th-anniversary milestone!! I’ve had the honor of serving as an advisory board member, both presently and previously as a Materials Horizons Community Board member since 2020. I’ve consistently been impressed by the journal's unwavering dedication to uncompromised high-quality research in cutting-edge areas that foster innovation. We are thrilled to present this article, demonstrating the successful detection of ammonia in extremely high humidity using porous SnO2 nanosheets. We wish another decade of continued success, excellence, and many more years of groundbreaking contributions to Materials Horizons! |
New concepts
The chemiresistive sensor is designed to distinguish ammonia from humidity using 2D SnO2 nanosheets purely based on its structural, morphological, and surface properties. The SnO2 nanosheet formation is optimized to have a microporous and oxygen rich-surface for selective interaction with ammonia in the presence of humidity. A direct electron transfer between NH3 molecules and adsorbed oxygen takes place at room temperature with limited access to water molecules. This approach led us to develop an ammonia sensor with the lowest detection limit of 64 ppt ever reported in the literature with a rapid response/recovery (8 s/55 s). The concept of control over porosity to achieve a humidity-tolerant nature in metal oxide-based sensing materials is demonstrated in this work and can also be extended to other classes of sensing materials for reliable sensors under extreme humidity conditions. Consequently, this work is indeed a step forward to real-time breath sensors for diagnostics where moisture is a ubiquitous interference source, especially for those operating at room temperature.
|
1. Introduction
The skyrocketing growth in chemical industries, agricultural activities, and the advent of the Internet of Things (IoT) has demanded constant environmental surveillance to minimize the circulation of harmful gases.1–4 Of them, ammonia (NH3) is a prominent gas produced through fertilizer production and industrial procedures worldwide.5,6 Ammonia is a cause for concern not only for the environment but also for human health. The existence of NH3 in breath can signify an uneven level of urea, which could be an outcome of renal disease or gastrointestinal troubles.7,8 Normally, individuals with good health have 0.4–1.8 ppm of ammonia in their respiration, while it can reach 14.7 ppm in people suffering from end-stage renal disease.9,10 Considering the criticality of NH3 monitoring for both environmental and health-related reasons, there is an immense demand for fast, selective, and efficient sensors that can work in real-life scenarios.
To address this need, sensors based on semiconductor metal oxides (SMO)11,12 have garnering substantial attention due to their incredible sensitivity, simple construction, affordability, straightforward fabrication, and capacity for real-time measurements. Among the metal oxides, tin dioxide (SnO2) is an especially encouraging material owing to its inexpensive cost, simple synthesis procedures, and one-of-a-kind physical and chemical properties.13 SnO2 has a wide bandgap of 3.6 eV and a tetragonal lattice structure that improves its efficacy as a gas sensor, giving it a competitive edge over other semiconductor metal oxides. Despite the various advantages of SnO2-based chemiresistive sensors, they suffer from high operational temperatures and low sensitivity towards ammonia. Therefore, there is a pressing requirement to address these limitations to create better sensors for ammonia detection in environmental and healthcare domains.
In the literature, doping and the engineering of heterostructures with SnO2 nanomaterials have been explored to improve the selectivity towards ammonia in humid settings and reduce the operating temperature of SnO2-based sensors. For instance, Yuan et al.14 and Xu et al.15 built sensors out of Ce-doped SnO2 and SnO2–SnS2 hybrids, respectively, that showcased augmented responses to ammonia at room temperature and various humidity levels.16–18 Nevertheless, the sensors continue to endure a considerable detection limit and become affected by humidity at the standard temperature. Another approach to bolster sensitivity and selectivity includes using different SnO2 nanostructures of various morphologies such as nanoparticles, nanowires, nanoneedles, nanowhiskers, nanobelts, and nanosheets to detect various gases and volatile organic compounds (VOCs) like acetone,13 ethanol,19–21 H2S,22 and formaldehyde.11,23 As an illustration, Kim et al.13 and Zhao et al.19 put together sensors employing SnO2 nanosheets and hollow SnO2 structures that both demonstrated heightened responses to acetone and ethanol. Among different SnO2 nanostructures, nanosheets have been discovered to be the most sensitive and selective.24 Unfortunately, these nanosheet-based sensors are only keenly sensitive to VOCs and necessitate elevated operating temperatures, making them inapplicable for ammonia detection in humid conditions at room temperature.
This study advances a new methodology to handle these difficulties by synthesizing porous SnO2 nanosheets via a pH-controlled, single-step solvothermal process. The investigation characterizes and optimizes the structural, morphological, and surface features of the SnO2 nanosheets, resulting in better sensing performance. The sensors produced from these SnO2 nanosheets demonstrate an exceptional sensitivity towards ammonia and can work proficiently in moist conditions at room temperature. This serves as a major leap in addressing the limitations of pre-existing SnO2-based sensors.
2. Experimental section
2.1. Synthesis of SnO2 nanosheets
SnCl2·2H2O (2.53 mmol) was dissolved in 160 mL of ethanol–water (1
:
1) solution and stirred for one hour. The precursor solution was adjusted to a pH of 14 by adding 10 mL of 1M NaOH before being transferred to a Teflon-lined hydrothermal vessel. The reaction was conducted for 12 h in an oven at 120 °C. The resultant dispersion was repeatedly centrifuged at 12
000 rpm with ethanol and water until its pH became neutral. The product was dried in a vacuum oven at 60 °C for 12 h. The same process was adopted for synthesizing SnO2 nanosheets at different pH conditions of 7 and 11 in place of 14. The synthesized material is homogenized by grinding and then dispersed in distilled water (0.1 mg mL−1) to fabricate sensors.
2.2. Sensor Fabrication
The glass substrates were cleaned thoroughly by ultrasonication. A carbon fiber shadow mask was manually placed on the glass substrate and tied at both ends with the help of scotch tape. Au was deposited by physical vapor deposition (VEC Vacuum, India) to fabricate ∼10 μm Au-coated gap electrodes. The sensing device was fabricated in a two-electrode geometry by drop-casting 5 μL dispersion (0.1 mg mL−1) across the gap. The fabricated sensor was vacuum-dried for 1 hour at 60 °C. Copper wire contacts were made using silver paste (SPI) for sensor measurements. The I–V measurements of all three sensing devices are performed under ambient conditions (∼50–60% RH and 25 °C). The I–V curve shows linearity in the voltage range of −1 V to +1 V, confirming the formation of ohmic contacts between the Au electrode and SnO2 nanosheets (Fig. S1, ESI‡).
2.3. Characterization
The crystal structure was studied using an X-ray diffractometer (D8, Bruker) equipped with Cu kα radiation (1.5418 Å). The chemical fingerprint and vibration modes were observed using a 532 nm laser diode Raman Spectrometer (Renishaw, UK). The specific surface area and pore size distribution were identified using the nitrogen adsorption–desorption isotherm derived from Brunauer–Emmett–Teller (BET) experiments (Autosorb IQ3). The pore size distribution is computed using nonlocal density functional theory (NLDFT), whereas the surface area is estimated using Multi-point BET measurements. X-ray photoelectron spectroscopy (XPS) was carried out using the Thermo scientific NEXSA surface analysis equipment utilizing the He1 radiation source (21.2 eV). Field emission scanning electron microscopy (FESEM) imaging is performed using (Tescan-Mira 3 LMH) for surface morphology. Transmission electron microscopy (TEM) was performed at Talos 2000S G2, FEG, with an EDS detector. Gatan GMS 3 software (open source) was used to analyze high-resolution TEM (HRTEM) pictures and electron diffraction (ED) patterns. The topology of the samples was characterized using atomic force microscopy (AFM Park system, XE-100). The zeta potential of the dispersion (1 mg mL−1) was measured using dynamic light scattering (DLS) from Malvern Instruments, Zetasizer Nano ZS90 (ZEN 5600). Ultraviolet-diffuse reflectance spectroscopy (UV-DRS) was used to determine the bandgap using a UV-Vis Spectrometer (Varian Cary 4) in an integrated sphere geometry. NH3 temperature-programmed desorption (NH3-TPD) measurements on the SnO2 nanosheet samples were performed using a chemisorption analyser (AMI-300, Altamira Instruments) connected to an NH3 cylinder with composition (5% NH3 + 95% Helium). Before NH3 adsorption, the SnO2 nanosheet samples were treated with Helium gas at 300 °C (10 °C min−1) for 1 h. Then, the adsorption of NH3 was regulated at 25 °C for 2 h. Finally, the NH3-TPD patterns were collected by ramping the temperature from 30 °C to 600 °C at a rate of 10 °C min−1 by using Helium gas.
2.4. Gas/VOC and humidity sensing measurements
Dynamic tests were performed in a custom-designed setup that entailed producing a VOC/gas of desired concentration using a calibrated syringe of 50 mL and dosing it on a sensor mounted inside a closed chamber at a controlled flow (∼100 SCCM) using a syringe pump as reported previously.25 In a 2 L round-bottom flask, VOCs and Ammonia of the required concentration were created by evaporation of liquid, and the target gas concentration was estimated using the static liquid–gas distribution technique using the formula:26 | 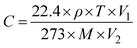 | (1) |
where C (ppm) is the target VOC concentration, T is the testing temperature (K), ρ (g mL−1) is the density of the VOC, V1 (μL) is the volume of VOC, V2 (L) is the volume of the chamber, and M (g mol−1) is the molecular weight of the VOC. Then, volatile organic compounds/gases were directly injected into the testing chamber using a syringe, while ambient air served as a diluent.
The response of the sensor was calculated using the formula:
| 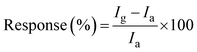 | (2) |
I
a and Ig represent the current at 1 V in the presence of ambient air and gases/VOCs, respectively. The response and recovery times are derived from the transient response as the time required for 90% of the total resistance change in the response/recovery profile. The selectivity is evaluated by analysing the response to different interfering polar and non-polar gases/VOCs.
Various concentrations of VOC/gas were calibrated using a Honey Wells (ppbRAE3000) pre-calibrated PID detector. The relative inaccuracy of the gas concentration readings generated using the designed apparatus was ∼14%. The humidity was created using a mist humidifier, and the VOC/gas response was calibrated under known humidity levels. I–t measurements were conducted at 1 V on the sensor positioned within the testing chamber in the presence or absence of VOCs. The commercial Testo 410-2 detector was also placed within the gas/VOC sensing chamber to monitor humidity and temperature continuously. For quantifying the repeatability of the sensor, relative standard deviation (RSD) was determined using the ratio of standard deviation to mean. The high RSD value is understandable, considering the RSD of 12% from gas calibration with the custom-designed setup. Unless otherwise noted, all gas sensing measurements were conducted at 30% RH and room temperature (∼25 °C).
2.5. Computational details
The computational study used first-principles density functional theory (DFT)-based calculations implemented in the Vienna Ab Initio Simulation Package (VASP).27 A plane-wave basis set with a cutoff of 420 eV, the projector augmented wave (PAW) method, and the generalized gradient approximation (GGA)-based exchange–correlational method are used for all the simulations.28,29 The dispersion correction is included as prescribed by Grimme (DFT-D3).30 3 × 3 × 1 and 6 × 6 × 1 k-point meshes are used for geometry optimization and self-consistent field (SCF) calculations, respectively. The geometries are relaxed until the forces per atom reduce to 0.01 eV Å−1. The energy convergence for SCF is fixed as 10−6 eV. To model the SnO2 surface, a supercell of the 2 × 2 × 2-unit cell (48 atoms) is generated, including a vacuum of >15 Å along the non-periodic z-direction. To understand the molecular adsorption, (110) and (200) surfaces are chosen. The adsorption energy is calculated using the formula, Eads = Etot − Es − Em, where Etot, Es, and Em are the total energies of the oxide slab with the molecule adsorbed on the surface, the SnO2 slab before the molecule adsorption, and the isolated molecules, respectively. As the formula indicates, the negative Eads signifies an exothermic reaction.
3. Results and discussion
3.1. Structural and morphological characterization
The SnO2 nanosheets are synthesized following a modified method reported in the literature.31 Briefly, the Sn(II) precursor is added to an alkaline deionized water and ethanol mixture for a hydrothermal reaction. The Sn4+ ions undergo rapid hydrolysis and oxidation, resulting in Sn(OH)62− complex ions that decompose under solvothermal conditions to form SnO2 nanosheets (Fig. S2, ESI‡). During the reaction, the small nuclei grow into crystals of SnO2 following the Ostwald Ripening Mechanism to minimize the Gibbs free energy. The crystal nuclei aggregate and self-assemble side-by-side in an orderly way along the (110) or (101) plane to minimize the high surface energy and form 2D nanosheets of SnO2. The pH conditions of the precursor solution greatly affect the crystallinity, morphology, and size of the formed SnO2 nanosheets. The amount of H+ and OH− ions in the precursor solution changes the nucleation and growth rates during the solvothermal reaction.32,33 The pH value of the precursor solution was finely tuned to control the hydrolysis rate of Sn(II) ions and, consequently, the size and crystallinity of the formed nanosheets.
TEM images in Fig. 1a–c show the effect of pH conditions of the precursor solution on the morphological evolution of SnO2 nanosheets. In the precursor solution with pH 7, slow nucleation and growth resulted in small-sized aggregates of nanosheets (Fig. 1a). However, as the pH of the precursor increases to 11, free OH− ions reacting with Sn2+ lead to an increased growth rate, forming a mix of large-size and aggregated SnO2 nanosheets (Fig. 1b). At a pH of 14, the excess OH− ions result in much faster hydrolysis, leading to continual and gigantic nuclei formation. This results in well-dispersed, uniform, ultrathin, and flexible nanosheets of ∼300–400 nm, as observed from TEM in Fig. 1c. The relatively transparent regions in the TEM image correspond to thin planar sheets, while the dark areas indicate crumbled perpendicular nanosheets. The uniform distribution of the nanosheets can be seen in the TEM images in Fig. S3 (ESI‡). Furthermore, the high-resolution TEM images in Fig. 1d–f revealed resolved fringes with a d-spacing corresponding to the (110) lattice planes of the tetragonal SnO2 crystal in all pH conditions, which is also the lowest surface energy plane. Eventually, the nuclei grow into crystals along the most stable crystal face of 110 or 101. The pH 14 nanosheets are analyzed further in detail (Fig. 1g). The Moiré patterns are observed dominantly along the sheet folding line due to different orientations between two or more SnO2 nanosheets stacked together. The Moiré fringes marked in two other regions in Fig. 1g are filtered by i-FFT, resulting in a lattice spacing of 0.24 nm corresponding to 200 planes. The intensity variation of the lattice planes shown by the yellow color has a bow-bending effect, indicating the line of the fold. The HRTEM image focusing on the proper edge areas is analyzed in detail to determine the thickness of the SnO2 nanosheets. The layer thickness from the vertical cross-section in the TEM image (Fig. 1g, marked) is observed to be 4–5 nm, matching with AFM thickness analysis (Fig. S4, ESI‡); thus, these can be called 2D nanosheets. The selected area electron diffraction (SAED) pattern of pH 14 reveals concentric circles indicating planes 110, 101, and 200, confirming the presence of the polycrystalline SnO2 phase (Fig. 1h). All SnO2 samples at different pH values form a stable aqueous dispersion with zeta potential values >−25 mV (Fig. S5, ESI‡). The large-size dispersed nanosheets in pH 14 are associated with the highest zeta potential value of −43.1 mV due to higher surface charge and enhanced stability.
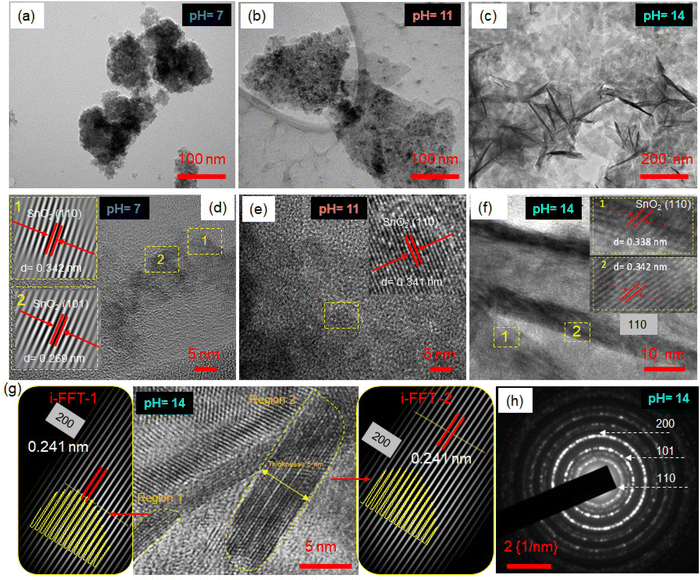 |
| Fig. 1 (a)–(c) TEM images and (d)–(f) HRTEM image analysis of SnO2 nanosheets prepared at pH 7, 11, and 14, (g) magnified HRTEM image and corresponding i-FFT analysis from different regions and (h) selected area electron diffraction (SAED) pattern of SnO2 nanosheets at pH 14. | |
X-ray diffraction patterns reveal that the obtained products are similar to the SnO2 (JCPDS data 41-1445) tetragonal crystal structure (Fig. 2a). Notably, most peak intensities in the XRD patterns of the SnO2 nanosheets increase as the pH value increases, illustrating improved crystallinity. The FWHM analysis presents an increase in crystallite size from 3.44 nm to 6.12 nm with an increase in pH from 7 to 14 (Table S2, ESI‡). In the pH 14 sample, Rietveld refinement of the XRD pattern indicates the presence of pure cassiterite SnO2 phase with a tetragonal lattice (Fig. S6, ESI‡). Furthermore, Raman spectra of all materials have a characteristic broad A1g peak centered at ∼624 cm−1 of Sn–O vibrations with the displacements perpendicular to the c-axis (Fig. S7, ESI‡).34 The peak broadening indicates the small size effect in all samples due to the presence of 2D nanosheets. Furthermore, the nitrogen adsorption–desorption curve shows a typical Type-IV isotherm with H3/H4 hysteresis, in agreement with the morphological changes. The aggregated sheets in pH 7 and pH 11 host mesopores (slit pores between the sheets) correspond to the typical H3 hysteresis (Fig. 2b and c).26 The specific surface area of 236.12 m2 g−1 and a maximum pore volume of 0.11 cm3 g−1 are obtained for the sample synthesized at pH 7, which is quite high among the values reported for metal oxides (Table S3, ESI‡). Increasing the pH to 14 reduces the specific surface area to 64.16 m2 g−1 and pore volume to 0.025 cm3 g−1 as the pore size and geometry changes to microporous narrow slit type with H4 hysteresis. Interestingly, these transitions in pore geometry, size, and volume with morphological evolution of SnO2 nanosheets at different pH conditions can play a crucial role in dictating the selective gas adsorption and diffusion behavior during sensing.
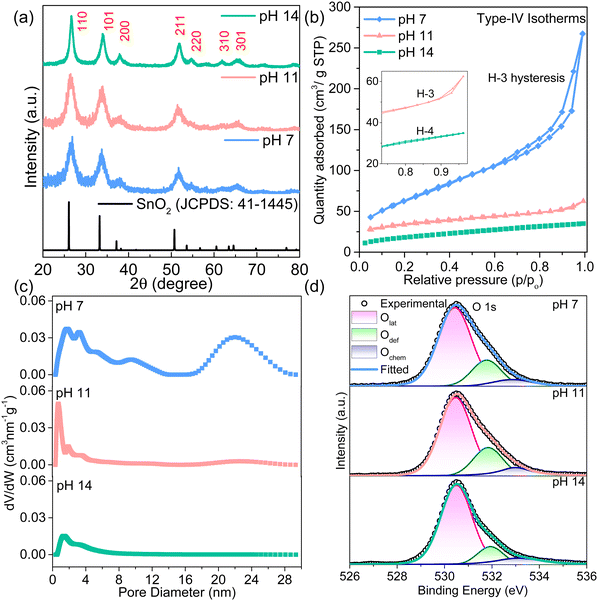 |
| Fig. 2 (a) XRD patterns, (b) nitrogen adsorption–desorption isotherms and corresponding (c) NLDFT pore size distribution of the synthesized samples at different pH conditions. (d) High-resolution O 1s core-level XPS spectra of all SnO2 nanosheets at pH 7, 11, and 14. | |
The XPS survey spectrum in Fig. S8 (ESI‡) depicts the presence of Sn, O, and C elements in all the samples, confirming the purity of the synthesized samples. The binding energy of C 1s at 284.6 eV was used as a reference. The deconvoluted C 1s and Sn 3d5/2 spectra are given in Fig. S9 (ESI‡). A low-intensity peak at 486.3 eV (Sn2+) corresponds to oxygen vacancies at the surface, and the intensity of this peak decreases with the increase in the pH (Table S4, ESI‡). The high-resolution O 1s spectrum is deconvoluted into three peaks at 530.5 eV, 531.9 eV, and 533.1 eV corresponding to lattice oxygen (O–Sn4+), oxygen deficiency (O–Sn2+) and adsorbed oxygen (Ochem), as shown in Fig. 2d. The area under the curve and intensity of the O–Sn2+ peak confirm the oxygen deficiencies in the SnO2 matrix of different pH samples that decrease with increasing pH values (Table S5, ESI‡). However, the chemisorbed oxygen initially increases slightly and saturates for pH 14 SnO2 nanosheets. The highest saturated chemisorbed oxygen in the pH 14 sample can enhance the active sites for the interaction of gas molecules. Thus, SnO2 nanosheets synthesized at pH 7, 11, and 14 are further used for sensing device fabrication and referred to as SNS-7, SNS-11, and SNS-14, respectively.
3.2. Gas/VOC sensing in humid conditions
As detailed in the experimental section, chemiresistive sensors were fabricated to investigate the effect of morphology on the efficacy of humidity, gas, and VOC sensing. The sensing performance is tested using the custom-designed setup shown in Fig. S10 (ESI‡). The sensor displayed a stable yet low baseline current at 40–60% RH humidity in the custom-designed chamber at room temperature (Fig. S11, ESI‡). The base current in the nA range was obtained at 60% RH due to a discontinuous water film formation at the surface of the sensing material (Fig. S11, ESI‡). Consequently, the selectivity of the sensor is tested in a natural environment (∼25 °C and ∼60% RH) in the presence of numerous interfering gases. The dip-stick method was used, where a swab soaked with a specific VOC was brought close to the sensor surface, and the sensor displayed an increase in current toward all molecules. It was interesting to observe that all three materials showed a significant response towards ammonia and humidity with a negligible response to other interfering gases (triethylamine, ethanol, acetone, 2-propanol, ethyl acetate, n-hexane, chloroform, toluene, benzene, and xylene), proving the SnO2 nanosheets selective interaction with ammonia and humidity (Fig. 3a). Furthermore, the humidity response follows the order SNS-11 > SNS-7 > SNS-14. Higher humidity response can be directly related to the mesopores of larger pore size and higher pore volume that allows more water molecules to be absorbed, increasing the humidity response.35 Thus, due to the additional presence of mesopores, SNS-7 and SNS-11 have much higher humidity response as compared to SNS-14 with only micropores. It also justifies the relatively higher NH3 response of SNS-14 as fewer water molecules can interact, leaving enough active sites for more ammonia molecules to react with the adsorbed oxygen on the microporous SNS-14 surface. At room temperature, the low dipole moment and small molecule size of NH3 relative to other interfering gases account for the high selectivity of all the sensors towards ammonia.36
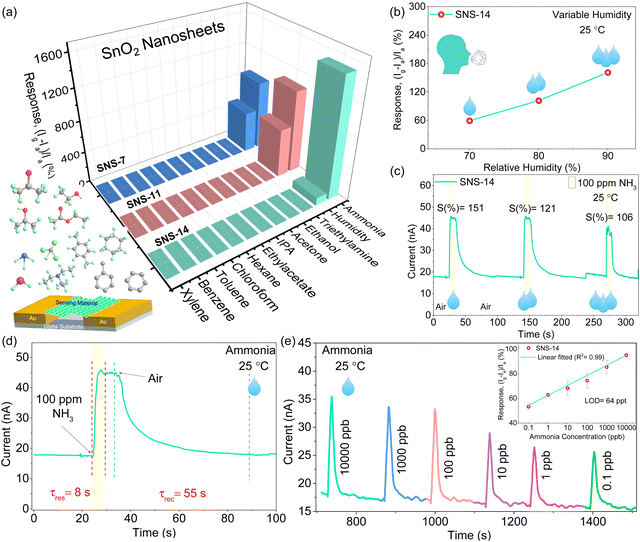 |
| Fig. 3 (a) A schematic of the fabricated sensor and the response of the sensors toward different gases/VOCs measured under the same test conditions using a dip-stick test. (b) SNS-14 response to humidity variation and (c) dynamic sensing transients toward 100 ppm ammonia at a varying relative humidity of 70–90%. (d) The response and recovery time of the SNS-14. (e) Sensing transients of SNS-14 toward different concentrations of ammonia gas and the inset shows the corresponding ammonia gas calibration plot at ∼25 °C and 70%RH. | |
Therefore, the strong permeation ability and electron-donating capacity of NH3 to SnO2 nanosheets increases the sensitivity at ambient temperature. However, among the three devices, SNS-14 exhibited the highest current response for NH3 (72% and 44% higher than that of SNS-7 and SNS-11, respectively). The relative difference in response may additionally arise from the difference in crystallinity, size of nanosheets, and specific surface area in all cases. The humidity-tolerant ammonia response of optimized SnO2 nanosheets (at pH 14) at room temperature is remarkable and a noteworthy addition to the VOC sensing field in general. It is a step forward to real-time breath sensors for diagnostics where moisture is a ubiquitous interference source, especially for those operating at room temperature. Exhaled breath typically contains humidity levels between 70 and 90% RH, which might degrade the sensor's performance in the long run.37 Thus, SNS-14 was evaluated in a highly humid environment (70–90%) to probe the influence of simulated breath humidity on the sensor. Although a linear trend was observed when humidity was increased from 70% to 90% RH, it was intriguing to see that the sensor exhibited only a little variation in response, boosting its durability for real-time operation (Fig. 3b and Fig. S12, ESI‡).
Furthermore, the ammonia response of the SNS-14 sensor was tested in variable humidity conditions (60–90% RH). The SNS-14 sensor tested at 100 ppm ammonia exhibited a significantly higher response at 60% RH compared to high humidity conditions (70–90%), which is expected due to less interference of water molecules and intrinsically low conductivity of SnO2 nanosheets (Fig. S13, ESI‡). However, on increasing the humidity to 70–90%, an appreciable sensing response with minimal variation is obtained (Fig. 3c). The SNS-14 sensor exhibited a rapid response and recovery time of 8 s and 55 s towards 100 ppm ammonia gas, much faster than the literature-reported values, Table 1 (Fig. 3d). The excellent response of SNS-14 to ammonia is evaluated further in the presence of calibrated gas concentration (10
000–0.1 ppb) at room temperature and high humidity (70%RH). A non-saturated response at low concentration values is obtained since the gas was exposed to the sensor for a specific time using a dynamic sensing technique (detailed in the Experimental Section). In its initial state, due to the adsorption of water molecules, the sensor displays a current in the nA range; upon introducing ammonia, the current rises (Fig. 3e). In addition, when the ammonia exposure concentration declines, so does the response, showing a linear relationship with the concentration of ammonia gas (see inset, Fig. 3e). The limit of detection (LOD) was determined to be 64 ppt using the formula (LOD = 3(SD)/sensitivity). The low LOD value indicates the potential of sensors in disease diagnosis from exhaled breath. The LOD value and response/recovery time of SNS-14 are better than those reported in the literature (Table 1). Thus, SNS-14 is an ideal choice for a ppt-level NH3 sensor due to its better room temperature sensing characteristics. Accuracy and stability are other crucial sensor reliability criteria for real-time ammonia gas monitoring. The long-term stability of the sensor towards 100 ppm ammonia in high humidity conditions (90% RH) was also tested for 540 hours, and the sensor demonstrated moderate stability (Fig. S14, ESI‡). Furthermore, the repeatability data depicted in Fig. S15 (ESI‡) indicate that even after five cycles of continuous 100 ppm ammonia exposure, the sensor maintained an RSD of ∼2%.
Table 1 Sensing performance of SnO2 nanosheets in comparison to recently reported ammonia sensors
Sensing material |
Temp (°C) |
Concn (ppm) |
Response (a, b, c) |
T
res (s) |
T
rev (s) |
LOD (ppb) |
RH (%) |
Publication year (Ref.) |
NA: Data not available. ΔR/Ra (%) or ΔG/Ga (%) or ΔI/Ia (%). Ra/Rg or Ga/Gg or Ia/Ig. |
g-C3N4@WO3 |
25 |
10 |
19.1b |
35 |
9 |
108 |
30 |
2022 (38) |
Ppy/f-MWCNT |
25 |
100 |
26.6a |
16 |
110 |
5000 |
30 |
2022 (39) |
SiMW |
25 |
100 |
50a |
200 |
>500 |
200 |
30 |
2022 (40) |
MoS2 @5keV |
25 |
200 |
340a |
30 |
47.9 |
NA |
50 |
2023 (41) |
Ti3C2Tx/SnS2 |
18 |
10 |
42.9a |
161 |
80 |
10 |
26 |
2023 (42) |
WS2/W18O49 |
25 |
5 |
25a |
73 |
189 |
50 |
18 |
2023 (43) |
Bi2Se3/Bi2O3 |
25 |
180 |
8.5b |
134.5 |
23.5 |
5000 |
20 |
2023 (44) |
WS2/MoO3 |
25 |
3 |
31.58a |
57 |
226 |
200 |
30 |
2022 (45) |
SnO2 Nanosheets |
25 |
100 |
106.5a |
8 |
55 |
0.064 |
90% |
This work |
To assess the capability of the SNS-14 device in sensing for medical diagnostics, NH3 sensing was performed using simulated breath samples containing small quantities of ammonia following the method reported in the literature.46 A healthy person's exhaled breath was collected in a 1 L balloon and injected into a round bottom (RB) flask of 100 mL volume. Then, a 10 mL volume of healthy breath is taken from the RB flask and injected over the sensor's surface using a syringe pump at a constant flow rate in ambient environmental conditions (without a custom-designed setup). Upon introducing healthy breath into the system, the sensor showed minimal fluctuations in current, primarily attributed to changes in humidity, signaling the absence of ammonia (Fig. S16, black line, ESI‡). Subsequently, to replicate a patient's exhaled breath, which typically contains approximately 1 ppm of NH3, 10 mL of 10 ppm NH3 was injected into the RB flask containing the healthy breath. Following the injection of this simulated diseased breath onto the sensor's surface, there was a substantial increase in the current response of the SNS-14 device, providing clear evidence of trace amounts of ammonia (Fig. S16, red line, ESI‡). Consequently, the SNS-14 sensor proves its potential utility in medical diagnosis.
3.3. Plausible NH3 sensing mechanism
Further insights into the gas sensing mechanism were obtained by studying the interaction of ammonia on the SnO2 surface by NH3-TPD measurements. The desorption profile of adsorbed ammonia at room temperature with a temperature ramp was analysed. NH3-TPD profiles of SnO2-based samples show two dominant peaks in the 40–200 °C range and 200–400 °C range, corresponding to the desorption of NH3 from two distinct acidic sites (Fig. 4a). The SnO2 nanosheets (pH 14) exhibited broad peaks at ∼120 °C and ∼400 °C, confirming the presence of weak and medium-strength acid sites for the interaction of ammonia. The Brønsted acid sites of weak strength are due to the amount of –OH and –O terminations at the surface of the SnO2 nanosheets, while Lewis acid sites with medium strength are due to coordinated unsaturated tin cations inducing oxygen vacancies in SnO2.47 Interestingly, for SnO2 nanosheets (pH 14), there is an additional peak at ∼475 °C attributed to the desorption of ammonia decomposed products in the literature.48,49 Thus, it can be concluded that pH 14 SnO2 nanosheets favor the interaction of ammonia at unsaturated Sn4+ centers and –OH and –O terminated sites, contributing to the enhanced sensing properties of the SnO2 nanosheets.
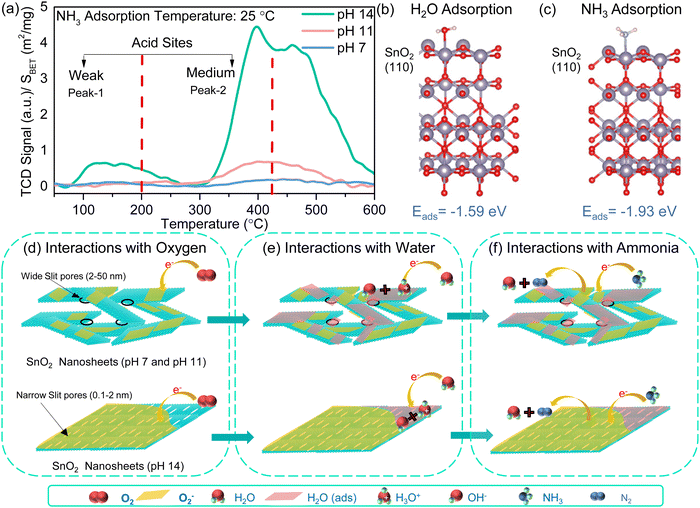 |
| Fig. 4 (a) NH3 TPD patterns of SnO2 nanosheet powders synthesized at pH 7, 11, and 14. The computationally optimized geometries of (b) H2O and (c) NH3 molecules on the (110) surface of SnO2. Schematic demonstrating the plausible sensing mechanism for SNS-7 and SNS-14 for (d) the oxygen adsorption step, (e) water adsorption, and (f) the interaction of ammonia with adsorbed oxygen on the SnO2 nanosheet surface. | |
Density functional theory (DFT)-based computational simulations are performed to understand the atomistic details of the interaction between the SnO2 surface and absorbing molecules. The dominant stoichiometric (110) surface of SnO2 has been considered for all the molecular adsorption processes.50 The (200) surface is also considered as this crystal plane forms the edge of the SnO2 sheet. We find insignificant surface reconstruction of these (110) and (200) facets upon computational geometry optimization. Note that the modelled surfaces do not contain any ionic vacancies. Here, water and ammonia are selected as the sensing efficiency of these molecules on the SnO2 surface is strikingly different. The optimized geometries in Fig. 4b and c depict that both molecules interact and chemically bind to the undercoordinated surface Sn atom on the (110) plane.51,52 The calculated adsorption energies (Eads) for NH3 and H2O on (110) facets are −1.93 and −1.59 eV, respectively. Moreover, the Eabs for NH3 and H2O on (200) are −0.20 and −0.17 eV, respectively. The negative binding energies indicate that both molecules can spontaneously bind through exothermic reactions and remain energetically stable in their absorbed form on the SnO2 surface. Nevertheless, the order of Eb reveals that in the presence of both the molecules, (110) and (200) surfaces of SnO2 would preferentially absorb NH3 molecules due to its higher thermodynamic stability. Close comparison between the optimized geometries of the SnO2 surface with and without absorbed NH3 molecules show minimal structural modifications upon molecular adsorption. These findings indicate that the oxide surface remains undistorted by the NH3 adsorption and desorption process, allowing it to be employed multiple times for sensing applications. Also, due to its stronger molecule binding than the (200) surface, we consider the (110) surface for further analysis. The electronic coupling and charge transfer between SnO2 and molecules play key roles in the stability and sensing applications. The Bader charges on the (110) surface and molecules are evaluated to quantify the direction and magnitude of charge transfer. The computed Bader charges illustrate that NH3 and H2O molecules donate charge to the surface but to different extents. The NH3 donates 0.11 e−, which is significantly higher than that for H2O (−0.04 e−). The charge density difference plots in Fig. S17 (ESI‡) also depict the same trend: the NH3 molecule depletes more charge to the surface than in the case of the H2O molecule. The stronger binding and higher charge donation from the NH3 molecule indicate its better detection on top of the SnO2 surface.
Thus, based on the surface chemistry insights of ammonia interaction with the SnO2 surface from XPS, NH3-TPD and computational techniques, the proposed sensing mechanism based on an oxygen adsorption model is well suited for enhanced sensitivity of SnO2 nanosheets toward ammonia in SNS-14 even at high humidity conditions involving a three step process of (I) interactions of oxygen with the sensing material, (II) interactions of humidity with the sensing material, and (III) interactions of ammonia with the adsorbed oxygen and humidity molecules. Under a dry air environment, oxygen species adsorb at the active sensor (SNS-7 and SNS-14) based on the sensor's temperature. At room temperature, the oxygen is adsorbed as O2− by following the reaction in the equation below.
| O2 (ads) + e− ↔ O2− (ads) | (4) |
Specifically, SNS-14 exhibits the highest chemisorbed oxygen (as observed in XPS analysis), thus possessing enormous active surface sites for sensing (Fig. 4d). The H2O molecules in humid air interact with the surface of SNS-7 and SNS-14, exhibiting increased surface adsorption. Thus, based on the Grotthuss conduction mechanism, an overall decrease in the base resistance (Ra) of the sensing devices is observed with increasing humidity following the reaction scheme, as shown in the equation below.
| H2O (ads) + H2O (ads) → H3O+ (ads) + OH− | (6) |
| H3O+ (ads) + H2O (ads) → H3O+ (ads) + H2O (ads) | (7) |
Interestingly, SNS-14 demonstrates minimal response toward humidity. This is expected because of the absence of mesoporosity in the SNS-14 and fewer sites for direct interaction (because of low specific surface area) with water molecules to form conducting water channels (Fig. 4e). Thus, for SNS-14, the humidity sensing performance saturates at high humidity conditions and shows an insignificant change in the humidity response (70–90% RH). As a result, SNS-7, with less chemisorbed oxygen and high adsorbed H2O molecules, displays poor ammonia response compared to SNS-14. Overall, SNS-14 acts as a catalytic material, and the ammonia molecules transform into non-reactive gas products to give a reversible sensing response by following the reaction scheme shown in the equation below.
| 4NH3 (ads) + 3O22− → 2N2 + 6H2O + 3e− | (8) |
Thus, SNS-14 can sense ammonia reliably because of the synergy of high chemisorbed oxygen and porosity that induces a direct electron transfer reaction between NH3 molecules and adsorbed oxygen and the insignificant impact of high humidity conditions (Fig. 4f).
4. Conclusions
One-step solvothermal synthesis by optimizing the pH conditions at 14 resulted in uniform SnO2 nanosheets with high chemisorbed oxygen, crystallinity, optimum specific surface area, and microporosity. At room temperature, the chemiresistive sensor based on SnO2 nanosheets showed exceptional ammonia sensitivity with minimal cross-interference from other gases or volatile organic compounds. Additionally, the ammonia sensitivity was remarkably tolerant to humidity levels, which has never been the case before. The room temperature ammonia detection down to the 0.1 ppb level (LOD = 64 ppt) with high selectivity and sensitivity of 150% (at 1000 ppb) accompanied by a faster response (8 s) and recovery (55 s) time greatly increases its potential for practical applications. Furthermore, SnO2 nanosheet-based sensors showed good cycling stability and consistent response even under extreme humidity conditions. As such, a sensor that can function in a damp environment is urgently required, and this research has the potential to be expanded in the direction of wearable healthcare sensing devices.
Author contributions
M. V.: synthesis of materials, sensor development and testing, data curation, characterization, analysis of results, validation, figures preparation, first draft writing, review, and editing of the manuscript. G. B.: discussions, reviewing, and editing of the manuscript. S. S.: reviewing and editing of the manuscript. A. K.: computational work analysis, writing, and editing. D. G.: computational work analysis, writing, and editing. H. H.: discussions, reviewing, and editing of the manuscript. R. G.: conceptualization, methodology, analysis of results, reviewing and editing of the manuscript, funding acquisition, and supervision of the project work.
Conflicts of interest
There are no conflicts to declare.
Acknowledgements
The authors acknowledge CRDSI, IIT Jodhpur and CRF, IIT Delhi for research facilities. The financial support from the Science and Engineering Research Board of India (Grant No. CRG/2020/003465 and SIRE Fellowship SIR/2022/000380) is acknowledged. M.V. thanks MHRD, Government of India, for the student fellowship. MV thanks Mr Shubhendra Shukla for technical assistance and Mr Himanshu Yadav for Rietveld Refinement. D.G. acknowledges the IIT Delhi SEED Grant (PLN12/04MS), the Science and Engineering Research Board (SERB), Department of Science and Technology (DST), India for Start-up Research Grant SRG/2022/00l234 for financial support, and the IIT Delhi HPC facility for computational resources. The computational studies were performed, in part, at the Center for Integrated Nanotechnologies, an Office of Science User Facility operated for the U.S. Department of Energy (DOE) Office of Science by Los Alamos National Laboratory (Contract 89233218CNA000001) and Sandia National Laboratories (Contract DE-NA-0003525).
References
- D. W. Jorgenson and K. Vu, Information Technology and the World Growth Resurgence, Ger. Econ. Rev., 2007, 8(2), 125–145 CrossRef.
- J. Zhang, X. Liu, G. Neri and N. Pinna, Nanostructured Materials for Room-Temperature Gas Sensors, Adv. Mater., 2016, 28(5), 795–831 CrossRef CAS PubMed.
- H. Tang, L. N. Sacco, S. Vollebregt, H. Ye, X. Fan and G. Zhang, Recent Advances in 2D/Nanostructured Metal Sulfide-Based Gas Sensors: Mechanisms, Applications, and Perspectives, J. Mater. Chem. A, 2020, 8(47), 24943–24976 RSC.
- H. Yousefi, A. Mahmud, D. Chang, J. Das, S. Gomis, J. B. Chen, H. Wang, T. Been, L. Yip, E. Coomes, Z. Li, S. Mubareka, A. Mcgeer, N. Christie, S. Gray-Owen, A. Cochrane, J. M. Rini, E. H. Sargent and S. O. Kelley, Detection of SARS-CoV-2 Viral Particles Using Direct, Reagent-Free Electrochemical Sensing, J. Am. Chem. Soc., 2021, 143(4), 1722–1727 CrossRef CAS PubMed.
- B. Timmer, W. Olthuis and A. Van Den Berg, Ammonia Sensors and Their Applications - A Review, Sens. Actuators, B, 2005, 107(2), 666–677 CrossRef CAS.
- D. Kwak, M. Wang, K. J. Koski, L. Zhang, H. Sokol, R. Maric and Y. Lei, Molybdenum Trioxide (α-MoO3) Nanoribbons for Ultrasensitive Ammonia (NH3) Gas Detection: Integrated Experimental and Density Functional Theory Simulation Studies, ACS Appl. Mater. Interfaces, 2019, 11(11), 10697–10706 CrossRef CAS.
- H. Y. Li, C. S. Lee, D. H. Kim and J. H. Lee, Flexible Room-Temperature NH3 Sensor for Ultrasensitive, Selective, and Humidity-Independent Gas Detection, ACS Appl. Mater. Interfaces, 2018, 10(33), 27858–27867 CrossRef CAS PubMed.
- G. Wu, H. Du, Y. L. Cha, D. Lee, W. Kim, F. Feyzbar-Khalkhali-Nejad, T. S. Oh, X. Zhang and D. J. Kim, A Wearable Mask Sensor Based on Polyaniline/CNT Nanocomposites for Monitoring Ammonia Gas and Human Breathing, Sens. Actuators, B, 2023, 375, 132858 CrossRef CAS.
- S.-Y. Jeong, J.-S. Kim and J.-H. Lee, Rational design of semiconductor-based chemiresistors and their libraries for next-generation artificial olfaction, Adv. Mater., 2020, 32, 2002075 CrossRef CAS.
- S. Kundu, S. J. George and G. U. Kulkarni, Parts per Billion Sensitive, Highly Selective Ambient Operable, Ammonia Sensor with Supramolecular Nanofibres as Active Element, Sens. Actuators, B, 2021, 347, 130634 CrossRef CAS.
- Y. Masuda, Recent Advances in SnO2 Nanostructure Based Gas Sensors, Sens. Actuators, B, 2022, 364, 131876 CrossRef CAS.
- E. P. Nascimento, H. C. T. Firmino, G. A. Neves and R. R. Menezes, A Review of Recent Developments in Tin Dioxide Nanostructured Materials for Gas Sensors, Ceram. Int., 2022, 48(6), 7405–7440 CrossRef CAS.
- K. Kim, P. G. Choi, T. Itoh and Y. Masuda, Catalyst-Free Highly Sensitive SnO2 Nanosheet Gas Sensors for Parts per Billion-Level Detection of Acetone, ACS Appl. Mater. Interfaces, 2020, 12(46), 51637–51644 CrossRef CAS PubMed.
- Y. Yuan, G. Zhan, W. Peng, C. Huang, H. Chen and S. Lin, Trace Ppb-Level NH3 Sensor Based on Single Petal-like Ce-Doped SnO2, Mater. Sci. Semicond. Process., 2023, 157, 107335 CrossRef CAS.
- K. Xu, N. Li, D. Zeng, S. Tian, S. Zhang, D. Hu and C. Xie, Interface Bonds Determined Gas-Sensing of SnO2-SnS2 Hybrids to Ammonia at Room Temperature, ACS Appl. Mater. Interfaces, 2015, 7(21), 11359–11368 CrossRef CAS.
- S. M. Majhi, A. Mirzaei, H. W. Kim, S. S. Kim and T. W. Kim, Recent Advances in Energy-Saving Chemiresistive Gas Sensors: A Review, Nano Energy, 2021, 79, 105369 CrossRef CAS PubMed.
- X. Kang, N. Deng, Z. Yan, Y. Pan, W. Sun and Y. Zhang, Resistive-Type VOCs and Pollution Gases Sensor Based on SnO2: A Review, Mater. Sci. Semicond. Process., 2022, 138, 106246 CrossRef CAS.
- R. Kim, J. S. Jang, D. H. Kim, J. Y. Kang, H. J. Cho, Y. J. Jeong and I. D. Kim, A General Synthesis of Crumpled Metal Oxide Nanosheets as Superior Chemiresistive Sensing Layers, Adv. Funct. Mater., 2019, 29(31), 1–10 CrossRef.
- Y. X. Li, Z. Guo, Y. Su, X. B. Jin, X. H. Tang, J. R. Huang, X. J. Huang, M. Q. Li and J. H. Liu, Hierarchical Morphology-Dependent Gas-Sensing Performances of Three-Dimensional SnO2 Nanostructures, ACS Sensors, 2017, 2(1), 102–110 CrossRef CAS.
- Y. Zhao, Y. Liu, Y. Ma, Y. Li, J. Zhang, X. Ren, C. Li, J. Zhao, J. Zhu and H. Zhao, Hollow Pentagonal-Cone-Structured SnO2Architectures Assembled with Nanorod Arrays for Low-Temperature Ethanol Sensing, ACS Appl. Nano Mater., 2020, 3(8), 7720–7731 CrossRef CAS.
- K. Suematsu, Y. Hiroyama, W. Harano, W. Mizukami, K. Watanabe and K. Shimanoe, Double-Step Modulation of the Pulse-Driven Mode for a High-Performance SnO2 Micro Gas Sensor: Designing the Particle Surface via a Rapid Preheating Process, ACS Sens., 2020, 5(11), 3449–3456 CrossRef CAS PubMed.
- P. M. Bulemo, H. J. Cho, N. H. Kim and I. D. Kim, Mesoporous SnO2 Nanotubes via Electrospinning-Etching Route: Highly Sensitive and Selective Detection of H2S Molecule, ACS Appl. Mater. Interfaces, 2017, 9(31), 26304–26313 CrossRef CAS.
- T. Yang, M. Zhu, K. Gu, C. Zhai, Q. Zhao, X. Yang and M. Zhang, Facile Synthesis of SnO2 Nanoparticles for Improved Formaldehyde Detection, New J. Chem., 2018, 42(16), 13612–13618 RSC.
- X. Han, M. Jin, S. Xie, Q. Kuang, Z. Jiang, Y. Jiang, Z. Xie and L. Zheng, Synthesis of Tin Dioxide Octahedral Nanoparticles with Exposed High-Energy {221} Facets and Enhanced Gas-Sensing Properties, Angew. Chem., Int. Ed., 2009, 48(48), 9180–9183 CrossRef CAS.
- C. Wang, Y. Zhou, M. Ge, X. Xu, Z. Zhang and J. Z. Jiang, Large-Scale Synthesis of SnO2 Nanosheets with High Lithium Storage Capacity, J. Am. Chem. Soc., 2010, 132(1), 46–47 CrossRef CAS PubMed.
- M. Verma, G. Bahuguna, A. Saharan, S. Gaur, H. Haick and R. Gupta, Room Temperature Humidity Tolerant Xylene Sensor Using a Sn-SnO2 Nanocomposite, ACS Appl. Mater. Interfaces, 2023, 15(4), 5512–5520 CrossRef CAS PubMed.
- M. Verma, G. Bahuguna, S. Shukla and R. Gupta, SnO2 Nanoparticle-Reduced Graphene Oxide Hybrids for Highly Selective and Sensitive NO2 Sensors Fabricated Using a Component Combinatorial Approach, ACS Appl. Nano Mater., 2022, 5(12), 19053–19061 CrossRef CAS.
- G. Kresse and J. Furthmuller, Efficient Iterative Schemes for Ab Initio Total-Energy Calculations Using a Plane-Wave Basis Set, Phys. Rev. B: Condens. Matter Mater. Phys., 1996, 54(16), 11169–11186 CrossRef CAS PubMed.
- G. Kresse and D. Joubert, From Ultrasoft Pseudopotentials to the Projector Augmented-Wave Method, Phys. Rev. B: Condens. Matter Mater. Phys., 1999, 59(3), 1758–1775 CrossRef CAS.
- I. Csonka, O. A. Vydrov, G. E. Scuseria, L. A. Constantin, J. P. Perdew, A. Ruzsinszky, X. Zhou and K. Burke, Restoring the Density-Gradient Expansion for Exchange in Solids and Surfaces, Phys. Rev. Lett., 2008, 100, 136406 CrossRef.
- S. Grimme, J. Antony, S. Ehrlich and H. Krieg, A consistent and accurate ab initio parametrization of density functional dispersion correction (DFT-D) for the 94 elements H-Pu, J. Chem. Phys., 2010, 132, 154104 CrossRef.
- Sukriti and P. Chand, Effect of pH Values on the Structural, Optical and Electrical Properties of SnO2 Nanostructures, Optik, 2019, 181, 768–778 CrossRef CAS.
- H. Wang and A. L. Rogach, Hierarchical SnO2 Nanostructures: Recent Advances in Design, Synthesis, and Applications, Chem. Mater., 2014, 26(1), 123–133 CrossRef CAS.
- W. Wan, Y. Li, X. Ren, Y. Zhao, F. Gao and H. Zhao, 2D SnO2 Nanosheets: Synthesis, Characterization, Structures, and Excellent Sensing Performance to Ethylene Glycol, Nanomaterials, 2018, 8(2), 112 CrossRef.
- Q. Yuan, N. Li, W. Geng, Y. Chi, J. Tu, X. Li and C. Shao, Humidity Sensing Properties of Mesoporous Iron Oxide/Silica Composite Prepared via Hydrothermal Process, Sens. Actuators, B, 2011, 160(1), 334–340 CrossRef CAS.
- G. Jeevitha, R. Abhinayaa, D. Mangalaraj, N. Ponpandian, P. Meena, V. Mounasamy and S. Madanagurusamy, Porous Reduced Graphene Oxide (RGO)/WO3 Nanocomposites for the Enhanced Detection of NH3 at Room Temperature, Nanoscale Adv., 2019, 1(5), 1799–1811 RSC.
- E. Mansour, R. Vishinkin, S. Rihet, W. Saliba, F. Fish, P. Sarfati and H. Haick, Measurement of Temperature and Relative Humidity in Exhaled Breath, Sens. Actuators, B, 2020, 304, 127371 CrossRef CAS.
- Z. Zou, Z. Zhao, Z. Zhang, W. Tian, C. Yang, X. Jin and K. Zhang, Room-Temperature Optoelectronic Gas Sensor Based on Core-Shell g-C3N4@WO3 Heterocomposites for Efficient Ammonia Detection, Anal. Chem., 2023, 95(3), 2110–2118 CrossRef CAS PubMed.
- S. D. Lawaniya, S. Kumar, Y. Yu and K. Awasthi, Flexible, Low-Cost, and Room Temperature Ammonia Sensor Based on Polypyrrole and Functionalized MWCNT Nanocomposites in Extreme Bending Conditions, ACS Appl. Polym. Mater., 2023, 5(3), 1945–1954 CrossRef CAS.
- Q. T. Le, A. S. Shikoh, K. Kang, J. Lee and J. Kim, Room-Temperature Sub-Ppm Detection and Machine Learning-Based High-Accuracy Selective Analysis of Ammonia Gas Using Silicon Vertical Microwire Arrays, ACS Appl. Electron. Mater., 2022, 5, 366 CrossRef.
- M. K. Rajbhar, S. De, G. Sanyal, A. Kumar, B. Chakraborty and S. Chatterjee, Defect-Engineered 3D Nanostructured MoS2 for Detection of Ammonia Gas at Room Temperature, ACS Appl. Nano Mater., 2023, 6(7), 5284–5297 CrossRef CAS.
- T. He, S. Sun, B. Huang and X. Li, MXene/SnS2 Heterojunction for Detecting Sub-ppm NH3 at Room Temperature, ACS Appl. Mater. Interfaces, 2023, 15(3), 4194–4207 CrossRef CAS.
- M. Manoharan, K. Govindharaj, K. Muthumalai, R. Pandian, Y. Haldorai and R. T. Rajendra Kumar, Highly Selective Room Temperature Detection of NH3 and NOx Using Oxygen-Deficient W18O49-Supported WS2 Heterojunctions, ACS Appl. Mater. Interfaces, 2023, 15(3), 4703–4712 CrossRef CAS.
- B. Das, S. Riyajuddin, K. Ghosh and R. Ghosh, Room-Temperature Ammonia Detection Using Layered Bi2Se3/Bi2O3: A Next-Generation Sensor, ACS Appl. Electron. Mater., 2023, 5(2), 948–956 CrossRef CAS.
- Y. Ou, W. Niu, Y. Zhou, Y. Guo, C. Gao and Y. Wang, Mesoporous WS2/MoO3Hybrids for High-Performance Trace Ammonia Detection, ACS Appl. Mater. Interfaces, 2022, 14(34), 39062–39071 CrossRef CAS PubMed.
- H. Y. Li, C. S. Lee, D. H. Kim and J. H. Lee, Flexible
Room-Temperature NH3 Sensor for Ultrasensitive, Selective, and Humidity-Independent Gas Detection, ACS Appl. Mater. Interfaces, 2018, 10(33), 27858–27867 CrossRef CAS.
- A. V. Marikutsa, M. N. Rumyantseva, E. A. Konstantinova, T. B. Shatalova and A. M. Gaskov, Active Sites on Nanocrystalline Tin Dioxide Surface: Effect of Palladium and Ruthenium Oxides Clusters, J. Phys. Chem. C, 2014, 118(37), 21541–21549 CrossRef CAS.
- N. Vorobyeva, M. Rumyantseva, D. Filatova, E. Konstantinova, D. Grishina, A. Abakumov, S. Turner and A. Gaskov, Nanocrystalline ZnO(Ga): Paramagnetic centers, surface acidity and gassensor properties, Sens. Actuators, B, 2013, 182, 555–564 CrossRef CAS.
- M. A. Centeno, R. Scotti, F. Morazzoni, J. Arbiol, A. Cornet and J. R. Morante, NH3 Interaction with Chromium-Doped WO3 Nanocrystalline Powders for Gas Sensing Applications, J. Mater. Chem., 2004, 14, 2412–2420 RSC.
- S. Das and V. Jayaraman, SnO2: A Comprehensive Review on Structures and Gas Sensors, Prog. Mater. Sci., 2014, 66, 112–255 CrossRef CAS.
- F. Shao, M. W. G. Ho, J. D. Prades and J. R. Morante, Interaction Mechanisms of Ammonia and Tin Oxide: A Combined Analysis Using Single Nanowire Devices and DFT Calculations, J. Phys. Chem. C, 2013, 117(7), 3520–3526 CrossRef CAS.
- B. K. Sahu, G. Kaur and A. Das, Revealing Interplay of Defects in SnO2 Quantum Dots for Blue Luminescence and Selective Trace Ammonia Detection at Room Temperature, ACS Appl. Mater. Interfaces, 2020, 12(43), 49227–49236 CrossRef CAS.
Footnotes |
† Dedicated to Prof. Giridhar U. Kulkarni (JNCASR) in celebration of his 60th year. |
‡ Electronic supplementary information (ESI) available: I–V Characteristics; literature comparison table; schematic depiction of the synthesis procedure; TEM images; Zeta potential data; crystallite size determination; Rietveld refinement data; Raman spectra; literature comparison of specific surface area and pore volume; structural characterization by XPS analysis; oxygen defect concentration calculations; schematic of the custom designed gas sensing setup; variation of the baseline current with humidity; humidity sensing transients; ammonia response at variable humidity; long-term stability; repeatability data of the sensor; simulated breath sensing; 3D plots of the charge density difference. See DOI: https://doi.org/10.1039/d3mh01078c |
|
This journal is © The Royal Society of Chemistry 2024 |
Click here to see how this site uses Cookies. View our privacy policy here.