Trends in heavy metals, polychlorinated biphenyls and toxicity from sediment cores of the inner River Thames estuary, London, UK†
Received
25th September 2019
, Accepted 9th January 2020
First published on 10th January 2020
Abstract
River islands (Ait or Eyot) within the inner tidal Thames serve as unique recorders of current and historical estuarine chemical pollution. Sediment cores from Chiswick Ait were assessed for contamination using Microtox® solid phase bioassay, stable isotopes (δ13C, δ15N), heavy metals and polychlorinated biphenyls (PCBs). Microtox® classified these sediments as non-toxic to moderately toxic and bulk isotopes identified a change in organic input. Metals Cu, Zn, Cr, Ni, Cd, Hg and Ag showed parallel rise, peak and fall profiles which when allied to a 207/208Pb and 137Cs based chronology supported major changes in trace metal contributions corresponding to approximate input times of 1940 (rise), 1963 (peak) and 1985 (fall). Metals ranged from Cu 15 to 373 mg kg−1 (mean 141 mg kg−1), Zn 137 to 1331 mg kg−1 (mean 576 mg kg−1), Cr 14–351 mg kg−1 (mean 156 mg kg−1), Pb 10 to 1506 mg kg−1 (mean 402 mg kg−1), As 1 to 107 (mean 38 mg kg−1), Ni 11 to 113 mg kg−1 (mean 63 mg kg−1), Cd 0.2 to 53 mg kg−1 (mean 9 mg kg−1), Hg 1 to 8 mg kg−1 (mean 4.6 mg kg−1) and Ag from 0.7 to 50 mg kg−1 (mean 7.5 mg kg−1). Down core total PCBs ranged from 10.5 to 121 μg kg−1 and mean of 39 μg kg−1. The rise, peak and fall of Cu, Zn, Cr, Ni, Cd and Ag pollution matched local sewage works' treatment discharge records. Whereas the Hg, Pb and As profiles were disconnected, reflecting alternative historic sources and or partitioning behaviour. Comparison to marine sediment quality guidelines indicate that Zn, Pb, Ni, Cd and Hg exceed action level 2, whereas sedimentary Cu, Cr and As concentrations were above action level 1 (no action) but below action level 2 (further investigation required). The river islands of the tidal Thames capture a unique contaminant chemistry record due in part to their location in the tidal frame (salinity minimum) and close proximity to west London.
Environmental significance
Priority pollutants accumulating in urban river-estuaries are an important societal issue because they impact ecological health and may indirectly transfer to humans via water or aquaculture. This study provides new insights into geochemical processing by examining long term contamination trends from a tidally flooded mud island situated in the Thames, London. Sediments were dated using a combination of PCB, radiogenic (137Cs) and isotopic (206/207Pb) chemistry. Heavy metal content regularly exceeded non-statutory limits used for marine management and were concentrated above background by anthropogenic input and salinity controlled physicochemical partitioning processes. Peak organic and metal concentrations were concomitant and occurred from 20 to 40 cm depth whereas surface equivalents were lower reflecting improved river management and heightened environmental legislation from the 1980s.
|
1. Introduction
The tidal River Thames (UK) is an exemplar urban catchment grossly affected by atmospheric, terrestrial and fluvial transported organic compounds and heavy metals originating from multiple sources including the city of London and upstream catchment inputs.1–8
Understanding the spatial and temporal variation of sedimentary pollution in the tidal Thames is of interest to environmental scientists, marine, estuary and river managers, waste water utilities as well as the public because: (1) individually or together the compounds and elements have been implicated to have adverse effects on human health; (2) shipping and port/harbours activities require dredging for clear navigation and berth clearance which in turn demands sediment quality evaluations for regulatory disposal compliance; (3) accelerating global climate change effects may drive an increase in intensity and frequency of fluvial and coastal flooding which mobilises buried legacy contamination and potentially transports them from river back to land; (4) the outer estuary supports about 22 fish species and hosts one the largest cockle fisheries in the UK.
Sedimentary pollution in the River Thames estuary has been investigated along the entire salinity gradient (∼120 km) using shallow cores and or surface grabs from intertidal foreshore and assessed for brominated flame retardants, sewage input, phosphorus and mercury.1,4,6,7 This includes specific local conservation sites or salt marshes of the outer estuary for heavy metals (copper), organo-metals (organotins) and halogenated organic compounds.9–11 Investigation of the toxic heavy metal, mercury (Hg), in foreshore samples reported mean concentrations of 2.1 mg kg−1 (n = 351) with some of the highest intertidal Hg concentrations in Europe at 7 to 12 mg kg−1 occurring at 40 to 50 cm from surface within central London sections (Waterloo Bridge, Rotherhithe, Tower Bridge, Millwall, Deptford and Greenwich peninsula).7
Taken together these earlier studies confirm that Thames sediments retain an appreciable but spatially and temporally variable pollution legacy that accumulates as a function of: (1) current velocity, salinity and particle-contaminant behaviour controlled by physicochemical sorption to organic matter coating clay and fine silts and chemical complexation and; (2) proximity to current and historical industrial and municipal works discharge points.7
The suspended sediment load of the tidal Thames is classified into four zones. Zone 1 stretches 27 km from Teddington Lock to Lower Pool (∼60 to 140 mg L−1); Zone 2 stretches 24 km connecting Lower Pool to Erith Reach (500 to 600 mg L−1); Zone 3, spans 20 km and covers Erith to Lower Gravesend Reach (200 to 400 mg L−1) and finally Zone 4 which extends from Gravesend to Sea Reach spanning a distance of 27 km and opens into the southern North Sea.12 This spatial division of the tideway supports the general view that the upper reaches of the tidal Thames (Zone 1) are sediment starved with little to no deposition and the majority of suspended sediment is transported through to Zones 2 to 4.6,12,13 Consequently few studies have reported the presence of contaminants (sediment quality) or commented on their possible environmental impact above the Boroughs of Chelsea and Kensington (Syon, Mortlake, Corney Reaches) where sub-surface sampling is confounded by scouring to bedrock and anthropogenic disturbance from bridges, slipways and other engineered infrastructure.1,6,7 Nevertheless, the upper sections of the tidal Thames contain ten ancient (Medieval) mud river islands known as Aits or Eyots which may when not disturbed retain chemical records of historic contamination.
The most downstream of Thames river islands, Chiswick Ait island, is situated within the London Borough of Hounslow whereas its east bank lies within the Borough Richmond. It is approximately 290 m in length and 45 m wide with a surface area of around 8700 m2 at the mean high-water mark. It is known to overtop and deposit silt and clay sediment and plastic waste several times each month (Fig. 1). At the current time Chiswick Ait is subject to erosion due to increasing flooding, more extreme rainfall events (which cause bank failure) and wave action induced by river traffic on the north-eastern bank. One of the last remaining willow-holts in the Thames consisting of several hundred trees has existed at the site since the middle ages.
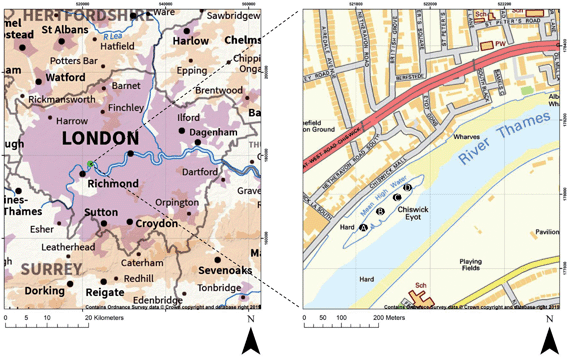 |
| Fig. 1 Study area and core locations on Chiswick Ait, River Thames, London, UK. | |
Chiswick Ait and its surrounding waters are important because they are used for public recreation including canoeing, sailing and rowing14 and also hosts the ‘The Boat Race’, a culturally significant rowing race between Oxford and Cambridge University (1856-present) which runs from Putney Bridge up to Chiswick Bridge with Chiswick Ait marking the halfway point of the race. A variety of current and past industries as well as major municipal works are located in close proximity, including, Fullers Brewery (1816-present day), Thorney Croft shipyard (Church Wharf 1864–1904), Cherry Blossom (later Rickett and Coleman) shoe polish works at Chiswick (1886–1972) as well as outfall from Mogden Sewage treatment works (established in 1939) which is one of the UKs largest facilities treating and discharging the waste of about 2 M people. The present study aimed to utilise Chiswick Ait's position close to the saline limit of the Thames and urbanised, but yet undisturbed, location to assess the combined point source and diffuse pollution inputs entering the tidal Thames through recent time (1930–2018 CE).
In this study we set out to:
(a) Determine whether the sediments in Chiswick Ait contained a discernible anthropogenic pollution chronology using established methods (trace metals, persistent organic pollutants).
(b) Evaluate potential toxicological impacts using Microtox® laboratory bioassay.
(c) Establish if the pollution comes from direct or indirect (diffuse) sources.
(d) Ascertain whether the trace metal geochemistry exceeded non-statutory river-estuarine sediment management criteria for the protection of ecology; and on-shore human health generic assessment criteria.
(e) Determine the total amount of contaminants contained in the 0–60 cm interval of Chiswick Ait and thereby predict the effect and impact of erosion of the Ait into the upper tidal Thames.
2. Methods
2.1 Core collection and preparation
Four sediment cores (A–D) were collected along an axial transect (51 29 13.4 N, 14 48.18 W to 51 29 17.1 N, 14 41.64 W) on Chiswick Ait (Eyot/Island), London, England on 28 March 2018 (Fig. 1). Photographs taken during the sampling campaign are presented in the ESI.† At each site, a clear polycarbonate tube (120 cm length, 6 cm I.D) fitted with a stainless steel basket catcher was manually driven into the exposed sediment and extracted to recover the core material. Upon collection, the core catcher (bottom ∼8 cm) was manually removed and the disturbed material discarded and the intact (undisturbed) sediment core transported to the laboratory in a cool box (∼4 °C). Each core was sectioned continuously at 5 cm intervals from 0–5, 5–10, 15–20 cm in order to identify any recent changes in pollution and then 10 cm intervals from 20–30, 30–40, 40–50, 50–60 cm in order to define historical pollution. Whole sediments were freeze-dried, sieved through a mesh aperture of 2 mm and the <2 mm fraction ground to a fine powder in an agate ball-mill.15,16
2.2 Microtox® solid phase toxicity bioassay
The Microtox® Solid Phase Test (SPT) was used to assess toxicity within the sediment using the test organism, Vibrio Fischeri (strain NRRL B-1117). For each interval, 7 g dry weight was transferred to a beaker and 35 ml SPT diluent (3.5% NaCl) added and 1.5 ml of the suspension transferred to SPT tubes. 1
:
2 serial dilutions were performed to give 2 controls and 13 dilutions in duplicate. Freeze dried bacteria were reconstituted and left in the reagent well of a Microtox® M500 Toxicity Analyser. Then 20 μl test reagent was added to each SPT tube. After 20 minutes the samples were filtered to remove the sediment and 0.5 ml of filtrate was transferred into glass cuvettes and analysed. The measurements were used to create a dose response curve and an EC50 value was calculated.
Microtox® SPT data are usually presented as effective concentration for a 50% biological effect (EC50 value) expressed either as mg L−1 or as EC50% which is calculated from EC50 expressed in mg L/10
000. The concentration or percentage values are then benchmarked against toxicity criteria (rating) elicited from either unpolluted control, sediment reference material and or published criteria.17,18 We used the very toxic <5000 mg L−1 (<0.5%), moderately toxic >5000 to <10
000 mg L−1 (0.5 to 1.0%) and non-toxic >10
000 mg L−1 (>1.0%) benchmarks.19 These criteria were supported by our analysis of a certified reference material (CRM) NIST 1941b, a sediment collected from the mouth of Baltimore Harbor, with moderately elevated concentrations gave a mean EC50 of 4318 mg L−1 and a median EC50 value of 4292 mg L−1 (0.42%) which on the basis of the published benchmarks would be taken to be just within the very toxic rating (Fig. 2).
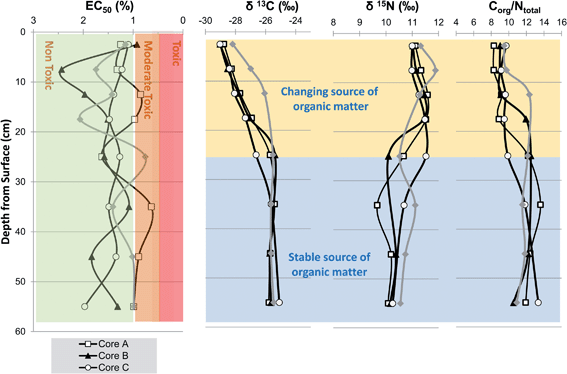 |
| Fig. 2 Vertical trends in sedimentary Pb isotope ratios 207/206Pb and 137Cs as chronological event markers at Chiswick Ait, River Thames, UK. | |
2.3. Stable carbon isotopes and C/N
Sediments were treated with 1 M HCl, left overnight, washed with deionized water, and oven dried at 60 °C.20 Analysis was performed with a Europa Scientific Elemental Analyser.21 The reference material used for δ13C analysis was A-R001 (δ13C = −26.43‰). For quality control purposes check samples of IA-R001, IA-R005 (δ13C = −26.03‰), and IA-R006 (δ13C = −11.64‰) were also analysed during the batch analysis of the samples. Replicate analysis of samples indicated an average standard deviation of 0.08‰. Total nitrogen of the original samples was analyzed using the same instrument. The reference material used during nitrogen analysis was IA-R001 (total nitrogen = 1.88%). All measured C/N values are reported on a weight for weight basis. All literature molar C/N values were converted to wt/wt using the conversion of 1.17 (i.e. 14/12).
2.4 Metal and Pb isotope ratio determinations
In preparation for determining concentrations of stable Pb isotopes, 0.25 g of sediment was dissolved by a mixture of concentrated HF/HClO4/HNO3 in Savillex™ PFA vials. Samples were reconstituted in dilute nitric acid and diluted to: (i) within the calibration range of Pb and Sb chemical standards for concentration measurements; and (ii) within the pulse counting range (<1 Mcps) of the ICP-MS for isotope ratio measurements. Concentration and isotope ratio determinations were made using a quadrupole ICP-MS instrument (Agilent 7500c) with a conventional glass concentric nebuliser. Details of accuracy and post data collection processing methodology have been reported previously.22 The limits of detection (mg kg−1) for metals used herein for sediment quality assessments were Cr < 0.05, Cr < 0.3, Ni < 0.9, Cu, Zn < 0.4, As < 0.03 and Cd < 0.4. Quality assurance was achieved by comparison with three reference materials, namely BCR-2 basalt, NIST 2711a Montana soil II and MESS-4 marine sediment (ESI†).23,24
2.5 Caesium-137 dating
Weighed dry portions of sediment (28–86 g) were transferred into containers of known geometry and sealed prior to being counted for 17–92 hours, achieving a relative counting uncertainty of 7% and a minimum detectable activity of 0.9–4.6 Bq kg−1. Analysis was carried out using a Mirion Canberra high-resolution gamma spectrometer fitted with a planar hyper-pure germanium detector. Calibration was carried out using Mirion Canberra's source-less calibration software and validated using the Certified Reference Material (Irish Sea Sediment IAEA-385; Cs-137 activity 33.0 Bq kg−1): measured value was 31.2 ± 2.0 Bq kg−1.
2.6 Mercury determination
Total mercury (Hg) was determined using a Milestone Mercury Analyser (DMA-80) instrument. Operating conditions were identical to those previously reported for evaluation of surface sediments of the Clyde estuary.25 Quality assurance was accomplished by analysing high (PACS-2, 3.04 mg kg−1), MID (TH2-1, 0.620 mg kg−1) and low level (MESS3-1, 0.091 mg kg−1) sediment Hg reference materials. Measured values were 3.24 mg kg−1 (RSD 2.41%, n = 4), and 0.66 mg kg−1 (RSD 2.1%, n = 2), 0.10 mg kg−1 (11.25%, n = 4) respectively and a limit of quantification of 0.005 mg kg−1.
2.7 Polychlorinated biphenyls
Sediment (10 g dry weight) was placed in a glass beaker and 20 ml of hexane
:
acetone
:
triethylamine (50
:
45
:
5% v/v) added. The sediment-organic solvent slurry was manually stirred (1 min), agitated on a rotary shaker for 30 min after which deionised water added to remove the acetone. The resulting hexane–triethylamine mixtures were then centrifuged and 1 ml of extract quantitatively transferred to a 2 ml vial and 10 μl of working internal was added. Concentrations of seven PCB were measured using a Varian 1200L triple quadrupole mass spectrometer (GC/MS). The MS was operated in selected ion mode with a scan time of 0.5 s. The limit of detection (LoD) for individual congeners was <3 μg kg−1, the LoD of International Council for the Exploration of the Sea (ICES-7), PCB 28, 52, 101, 118, 138, 153, 180) was <21 μg kg−1, the LoD for the World Health Organisation 12 (WHO-12) sum PCB 77, 81, 126, 169, 105, 114, 118, 123, 156, 157, 167, 189 LoD was 36 μg kg−1. Quality assurance was accomplished by analysing NIST1941b Organics in Marine Sediment reference material (n = 3). Comparison of certificate ranges and mean measured values were as follows: PCB 28, 4.52 to 4.49 μg kg−1; PCB 52, 5.24 to 5.50 μg kg−1; PCB101, 5.11 to 5.34 μg kg−1; PCB 118 4.23 to 4.20 μg kg−1; PCB 153 5.47 to 5.90 μg kg−1; PCB 138, 3.60 to 3.71 μg kg−1; PCB 180, 3.71 to 3.70 μg kg−1.
3. Results and discussion
3.1 Vertical trends in grain size and organic matter
The four sediment cores were visually inspected and found to be of remarkably constant sediment composition being comprised of about 50% clay, 30% fine-medium silt 20% and no discernible sand or pebble particles. The consistent clay–silt dominated composition of the cores was confirmed by trace element analysis which revealed uniform profiles of the common elements found in close association with clay mineral fraction namely, Al, Ti, Li and Rb (ESI†). Therefore, it was decided that grain size normalisation was inappropriate and that raw concentrations and/or ratio profiles should be used to elicit an anthropogenic sediment history at Chiswick and the inner Thames.
Stable carbon isotopes (δ13C) and C/N ratios have been widely employed to provenance sedimentary organic matter in coasts as well as track lateral and vertical (down core) transformations in organic rich sub-environments such as salt marshes and mangroves.21,26–28 In this current study, the δ13C, δ15N and C/N profiles exhibit a change at 25 cm depth such that the δ13C −25.5‰, δ15N +10.3‰ and C
:
N 11.9 values at 55 up to and including 25 cm reach near surface values (0–5 cm) δ13C −29.4‰, δ15N 11.3‰ and C
:
N 8.3 (Fig. 2). Previous monitoring of suspended particulate organic matter (POM) in the Thames showed two main sources of natural organic, namely, a riverine phytoplankton-detritus end-member characterized by δ13C −30‰, δ15N +7.9‰ and C
:
N 7.8 and a marine end member with δ13C −21‰, δ15N‰ 6 and C
:
N 14.8.29 A downstream transect across the salinity range (9 sites) revealed a step wise change in δ13C and C/N from POM with significant riverine-phytoplankton to marine values at a salinity 0.4, after-which these values were maintained out to sea. In contrast POM δ15N ranged from 7.8 to 11.7 but did not exhibit a clear seaward trend.29
The values encountered between 25–55 cm depth are broadly similar to a mixed terrestrial-marine signature (δ13C −25.5‰, δ15N 10.3‰ and C
:
N 11.9) whereas the upper 0–25 cm are characterized by very depleted δ13 values (−30 to −28‰) are more comparable to those previously attributed to riverine phytoplankton and their detritus.29,30 On balance, the profiles presented in Fig. 2 partly suggest that the natural organic matter supply changed from a typical mid-Thames to one that contains a more significant input of riverine phytoplankton. Based on the geochemical event chronology by Pb isotope and Cs-137, these changes could reflect a change in organic matter input from the inferred date of 1980 to present day (Fig. 3). Urban-industrial estuaries such as the Thames receive appreciable amounts of anthropogenic pollution such as sewage, industrial effluent and/or agricultural run-off that can overprint natural organic matter signatures and effect local biogeochemical cycling.4 Also, the study site at Chiswick Ait is situated 8 km downstream of the discharge point of the UKs third largest sewage treatment works (Mogden STW) and close to a major beer brewery (Fullers thus a Mogden sewage or other industrial effluent signature might be expected. Depending on extent of treatment, sediments with sewage input are reported to have δ13C of −26 to −22‰ and δ15N‰ +1 to +15‰.31–33 Sediments containing raw sewage exhibit lower δ15N values of 1–2‰ whereas those receiving more extensive tertiary treatment have enriched δ15N of 15‰.34,35 However, these wide ranges in δ13C and δ15N together with a lack of appropriate effluent end-member samples confound a clear-cut indication of sewage input or other effluent and/or the effect of known historical improvements to sewage treatment (primary to tertiary) at Mogden STW.
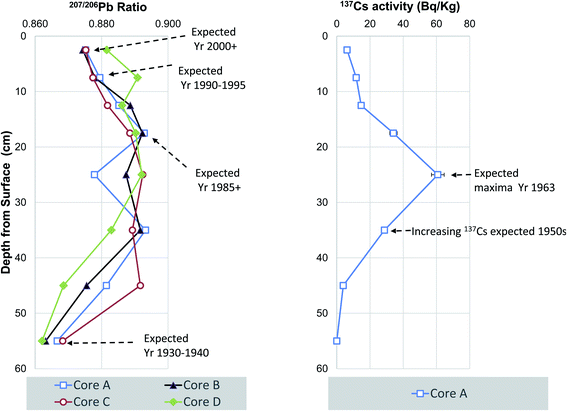 |
| Fig. 3 Vertical trends in sediment toxicity, bulk isotope and Corg/Ntotal at Chiswick Ait, River Thames. (Corg/Ntotal are expressed on wt/wt basis). | |
All four cores exhibit a decrease in C/N from 11 to 12 to 8 to 9 close to the surface (0–20 cm), whilst this could be due to changing source it is also consistent with preferential decay of N containing organic structures (e.g. amino-acids in proteins) by bacteria during early diagenesis (Fig. 2).36,37 The extent of the change in near surface C/N varying according to a range of factors, including degree of post depositional decay (transport distance time, re-working), physical integrity of detritus and biological community.38,39
3.2 Sediment toxicity screening
The Microtox Solid Phase Test (SPT) was used to assess the general toxicity within the sediment using the test organism, Vibrio Fischeri (strain NRRL B-1117), a luminescent bacterium. This strain of the organism can be used to evaluate both acute and chronic toxicity as it is sensitive to a broad range of chemicals (organic, trace metals and organo-metals) and used in studies of riverine, estuarine and marine sediments from Europe, North and Central America.19,40–43 The EC50 profiles (i.e. 50% decrease in light output) show that sediments in cores A, B and D are non-toxic to moderately toxic whereas all core C intervals were non-toxic (Fig. 2). Overall, no interval gave values below the 0.5% EC50 (<5000 mg L−1) benchmark which suggests that none of the sediments on Chiswick Ait fall within the very toxic category (Fig. 2). However, there are some limitations to the approach applied herein, firstly the test was applied to dry rather than wet sediments and secondly it has been shown that the luminescent bacteria can yield artificially low EC50 values (suggesting enhanced toxicity) due to physical adsorption to fine silty sediments.44 Identification of the main chemical causes of moderate toxicity is challenging because of attenuating factors such as bioavailability, bioaccessibility, speciation effects as well as antagonistic and additive effects (e.g. cocktail of organic and inorganic contaminants).42,45–47
3.3
207/206Pb and 137Cs geochronology
In the UK the trends in sedimentary Pb isotopes, namely 207/206Pb can be compared to historical vegetation time-series spanning 170 years that mainly record atmospheric Pb pollution.48,49 These archives reveal that 207/206Pb values remained at about 0.85 through the nineteenth century and increased from 1920 onwards owing to the use of leaded petrol and overseas industrial lead sourced in the main from Broken Hill, Australia. Maximal 207/206Pb values of 0.89 are observed in the 1980s and then declined once commercial sale of unleaded petrol came into effect after 1985. These archives allow the construction of 207/206Pb chronologies that may be used in conjunction with chemical event markers such as the ban on chlorinated persistent organic contaminants such as PCB and certain PBDEs.50 The 207/206Pb to depth bi-plots all show a consistent low 207/206Pb values from 0.8621 to 0.8683 (at 55 cm depth, variable maximal 207/206Pb values ranging from 0.8291 to 0.8928 from 45 to 25 cm and on-set of a drop in 207/206Pb values to 0.8818 to 0.8887 at 12.5 cm with the lower 207/206Pb of 0.8744 to 0.8816 near surface (Fig. 3). Based on previous published 207/206Pb values we assign an approximate date of 1930–1940 to the base of the cores (A–D), a date of about 1985 at 17.5 cm depth for cores for cores (A–C) and due to the slight disconnect in the profile the same date is ascribed to 7.5 cm for core D. Finally, the uppermost near surface sediment intervals yielded 207/206Pb values expected for 2000 or later, whereas surface sediments of core D had a higher ratio commensurate with an expected date of approximately 1990 to 1995 (Fig. 3).
Caesium-137, the man-made radionuclide produced via fission with a half-life of 30 years, is widely distributed throughout parts of the world due to the large scale atmospheric testing of nuclear weapons from 1954 to 1963 which reached its zenith in 1963. Therefore, notwithstanding down-core diffusion and post depositional mixing and discharge/generation record to deposition, maximal sedimentary 137Cs activity is assigned a date around the mid to late 1960's. Other 137Cs sources relevant to the UK, include discharges from the reprocessing of nuclear fuel at Sellafield nuclear into the Irish sea approximately 1952, 1958 and 1970s as well as deposition of atmospheric fallout from Chernobyl nuclear power generation accident in 1986. Therefore, either single or multiple peaks in 137Cs activity can in some instances and be used to identify time – stratigraphic markers in UK aquatic sediments.51–55
The 137Cs activity measured in core A and shows a consistent low 137Cs values from <1.9 to 3.9 Bq kg−1 at 40 to 50 cm depth, a single peak maxima of 60.8 Bq kg−1 at 20 to 30 cm and drop in 137Cs activity to 6.2 Bq kg−1 at the surface (0 to 5 cm depth) (Fig. 3). Based on previous published 137Cs data, we attribute this to atmospheric fallout from nuclear weapons testing with an approximate date of 1963 to 30 cm depth which is commensurate with the dates suggested by 207/206Pb. The absence of a second smaller Chernobyl related 137Cs peak is most likely because deposition in the UK did not occur in the London area but along the west coast of Britain, North Wales, Cumbria and Southwest Scotland instead.56
3.4 Historical trends of heavy metals
Trace metal concentration profiles exhibit a consistent basal low to mid core high to near surface low profile resulting from increased pollution in the 1950 to 1970 and recent fall in pollution from 1980s (Fig. 4 and 5). These trends support earlier studies that suggest that levels of sediment heavy metal pollution in the Tidal Thames are decreasing as a consequence of environmental legislation, improved effluent treatment procedures and river management.7 Heavy metal concentrations presented herein decrease from an inflection point assigned a date from mid 1960s to early 1970s, this systematic decrease toward surface follows the national atmospheric emissions inventory which reported reductions in As, Cd, Ni and Pb of 82–98% observed from 1970 to 2002.57
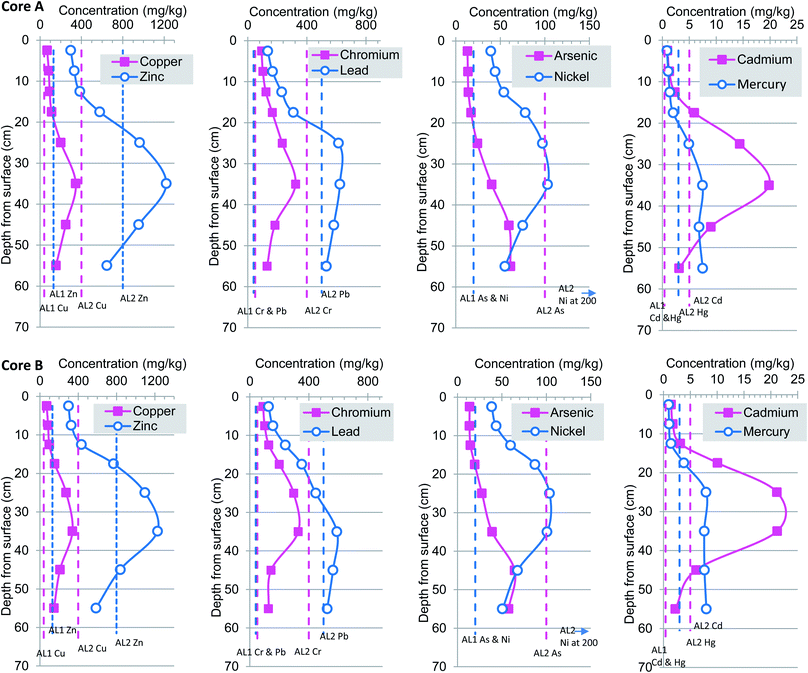 |
| Fig. 4 Heavy metal concentrations profiles for cores A and B. Dashed vertical lines represent non-statutory sediment quality guidelines. | |
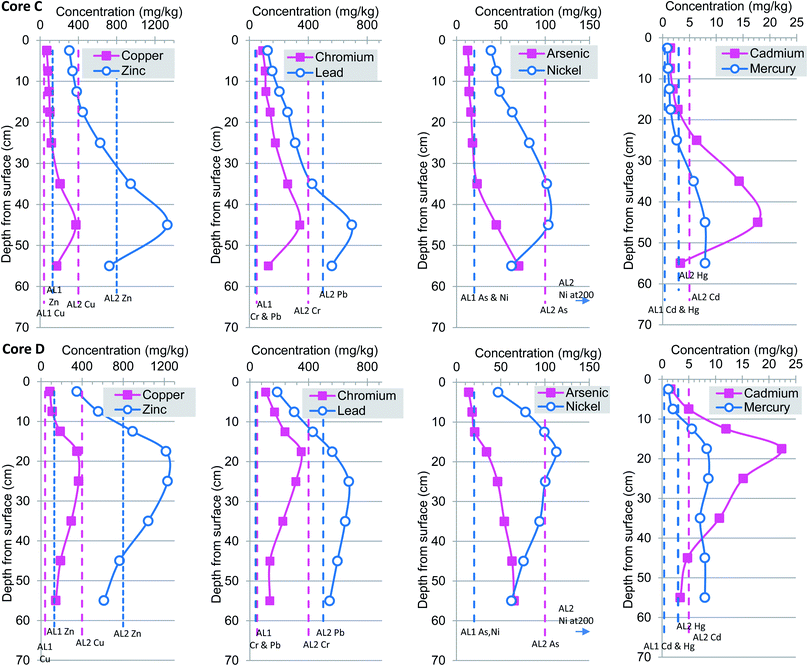 |
| Fig. 5 Heavy metal concentrations profiles for cores C and D. Dashed vertical lines represent non-statutory sediment quality guidelines. | |
In this current study, concentrations of heavy metals exhibit considerable variation with depth and ranged from Cu 15 to 373 mg kg−1 (mean 141 mg kg−1), Zn 137 to 1331 mg kg−1 (mean 576 mg kg−1), Cr 14–351 mg kg−1 (mean 156 mg kg−1), Pb 10 to 1506 mg kg−1 (mean 402 mg kg−1), As 1 to 107 (mean 38 mg kg−1), Ni 11 to 113 mg kg−1 (mean 63 mg kg−1), Cd 0.2 to 53 mg kg−1 (mean 9 mg kg−1), Hg 1 to 8 mg kg−1 (mean 4.6 mg kg−1). The vertical changes in heavy metal concentrations for Cu, Zn, Pb, Cr, As, Ni, Cd and Hg together with appropriate marine-river sediment action levels 1 and 2 are presented in Fig. 4 and 5. For ease of comparison these are plotted as pairs based on similar concentration ranges rather than atomic mass. Inspection from the base of cores (A–D) upwards reveals a clear rise-peak-decrease of Cu, Zn, Cr, Ni, Cd concentrations which are interpreted to reflect changing levels of historical anthropogenic pollution (Fig. 4 and 5). A somewhat different down-profile was observed for As, Pb and Hg suggesting an earlier period of input but with a concomitant decrease in metals from about 1980s. Overall, the highest concentrations of Cu, Zn, Pb, Cr, As, Ni, Cd and Hg occurred in cores A and B at 35 cm, core C at 45 cm and core D nearer the surface at 25 cm. The changes in depth correlation observed in core D are most likely due to local differences in connection frequency to the main Thames channel. Specifically, core D is situated in close proximity to two small inlets which permit greater in-flow of particulates and or erosion of sediment as compared to sites A–C (Fig. 1). In contrast the more minor variations in depth correlation between cores A–C maybe attributed to: (1) core site elevation; (2) differential compaction of the clay–silt host sediment during collection-transport; and or; (3) smearing, averaging effects caused by fairly coarse sampling regime at 5 or 10 cm increments.
The similarity in trace metal profiles (A–D) combined with 207/206Pb isotope based chronology (Fig. 3–5) suggests a very consistent physio-chemical behaviour which may, in part, be attributed to the rather uniform clay/silt lithology as supported by grain size proxy data (Al and Ti) (ESI†). In addition, the correspondence may be explained by the fact that the Ait is only occasionally flooded which, in turn, minimises the effects of suspension and resuspension of particulates that occur and contribute to the mixed geochemical profiles reported within the intertidal and subtidal Thames.7,12,26 Previous studies of heavy metal deposition in the Orwell and Stour estuaries of eastern England reported that greater amounts of sediment were deposited in winter than in the summer; similarly the Ait is mostly subject to episodic (seasonal?) flooding from November–March.58 Therefore, we hypothesise that the pollution accumulating on the Ait is mainly a winter deposit. Furthermore, no lateral (spatial) distribution trends were evident so that higher concentrations were observed in core A as compared to D or vice versa, which is entirely in keeping with the relatively short lateral transect distance of ∼280 m and distance between sites (Fig. 1–5). This finding differs from that reported for foreshore sediments collected ∼1 km apart which reported low unvarying total Hg concentrations from Brentford (Grand Union Canal), Chiswick Bridge, Barnes Bridge and Hammersmith Bridge as compared to rise-peak and fall at Wandsworth a site some 5 km downstream.7 This suggests that historic protected (clay–silt) islands such as Chiswick Ait have steadily accumulated polluted sediment and provide a higher resolution geochronology of anthropogenic contaminants than their foreshore or channel counterparts.
3.5 Historical trends in sedimentary silver (Ag)
Owing to its biological toxicity and strong affinity for fine particulates in low salinity water the sedimentary accumulation of silver (Ag) in international and UK estuaries has been reported.59,60 These studies confirmed that Ag in surface sediments from rural catchments (e.g. Dyfi, Axe, Hamble, Rother) range from about 0.1 to 0.2 mg kg−1, whereas those from more industrialised estuaries (Humber, Tyne Tees, Mersey) display values ranging from 0.4 to 2 mg kg−1 with the highest concentrations reported from river-estuaries accumulating mining waste or effluent and sludge.
In this current study, the mean surface Ag concentrations were 2.53 mg kg−1 (0 to 5 cm) and 3.46 mg kg−1 (0 to 10 cm) which are amongst the highest reported in UK river-estuaries including those with a high urban population or industrial legacy (Fig. 6). The elevated Ag content in surface and near surface sediment of the Thames at Chiswick is most likely due to two factors. Firstly the actual anthropogenic input must be high and most likely explained by local sewage treatment works effluent (Mogden) and or an unknown industrial source. Secondly, the elevated Ag concentrations at Chiswick follows earlier laboratory partitioning experiments that report preferential Ag sorption and partitioning from dissolved to particulate phases, at low salinities such that at 0.1‰ salinity Ag concentration is about ten times higher than at 23‰ salinity.61 Chiswick Ait is situated in salinity Zone 1 which spans Teddington to London Bridge and has a low salinity in the range of 0.14–1.96.3,12 Therefore, the elevated Ag concentrations observed in this current study may be due to sedimentary sequestration of Ag driven by the salinity variable.
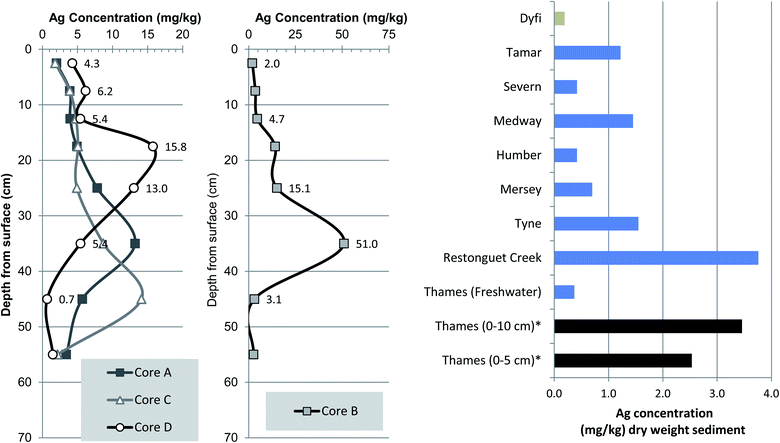 |
| Fig. 6 Vertical trends in Ag (silver) concentrations and comparison of mean values with other UK river-estuaries. | |
A 2014 survey of river bed-sediments from sites within the freshwater Thames catchment (Wallingford, Bracknell, Newbury, Caldecott and Littlebrook) revealed considerable spatial variation in Ag content with maximal concentrations ranging from 0.038 to 5.290 mg kg−1 and mean Ag of 0.031 to 1.189 ng kg−1.62 The same study also revealed that sewage sludge cake, the anaerobically treated and partially dewatered commercial product used in agriculture and landscaping, had concentrations of 3.5 to 42 mg kg−1, with a mean of 2.77 mg kg−1, median 3.56 mg kg−1. However, to the best of our knowledge no data from the tidal Thames have been reported in peer review literature.59,60
Inspection of the sediment core Ag profiles reveal the same rise and fall trends as Cd, Cr and Ni suggesting a common pollution history and possibly related input source(s) or partitioning behaviour (Fig. 4–6). Cores A, C and D all showed similar maximal Ag concentrations of 13.2, 14.1 and 17.5 mg kg−1 respectively (Fig. 6). However, core B, 35 cm depth interval had the highest Ag concentration at 51.0 mg kg−1, which is to the best of our knowledge is one of the highest ever reported in UK river-estuary sediments. It is also important to note that although there is a clear decrease in values toward the base of each core, the lowermost intervals Ag concentrations ranging from 1.5 to 3.4 mg kg−1 are elevated as compared to surface sediments from 19 other UK estuaries; a notion further confirmed when typical riverine background of about 0.3 mg kg−1 is considered.59 Overall, the elevated Ag concentrations observed here, in the inner tidal Thames, are in agreement with the process based understanding that Ag enrichment in contaminated estuaries can attain concentrations up to 50 times higher than the natural background.59,60
Anthropogenic Ag in coastal and estuarine sediments mainly originates from sewage sludge, effluent discharges, landfill, mining, smelting as well as possible photographic sources.59,63 The most plausible explanation for the elevated Ag content of Thames sediments is that it stems, at least in part, from Mogden STW which discharges treated and occasionally untreated effluent flow close to Isleworth Ait which is situated 8.2 km upstream of the cores sites at Chiswick Ait (Fig. 1). This source attribution is, in part, substantiated by the parallel increase in Ag content and on-set of PCB (1960s) which tracks increasing volumes of effluent discharge and various upgrades to the STW in 1962 and 1989. Unfortunately the Pb206/207 based chronology is not precise enough to identify whether the deepest interval pre-dates the first operations at Mogden STW in 1936.
Lateral and vertical concentrations of trace metals in river-estuaries can be modified by either adsorption or precipitation onto clay mineral surfaces (grain size effect), complexing with natural organic matter (TOC effect) and or scavenging of metals by Fe–Mn oxide coatings.7,52,64–66 The grain size effect is usually considered the predominant process and can be easily countered by normalisation with elements (Al, Ga Ti, Li, Rb) that are associated with the clay fraction in order to present a less biased, grain size variable free concentrations.52,67 However, in this current study the Al, Ti, Li, Rb grain size proxies were remarkably consistent confirming that normalisation was, in this instance, inappropriate; a view substantiated by field observation fine silt–clay through-out all cores (ESI†).
3.6 Vertical distribution of polychlorinated biphenyls
Polychlorinated biphenyls (PCBs) were commercially available from 1929 and were used into the late 1970s in a variety of products including dielectric and coolant fluids in electrical apparatus, carbonless copy paper, paint, resins, sealants as well as hydraulic and heat transfer fluids and plaster board.68–71 Although approximately 100 ton of PCBs were imported to the UK from 1930s to early 1950s the chemical product was not widely available until the onset of UK production in 1954; thereafter, approximately 66
748 ton were produced in the UK of which 39
466 ton were sold in the UK.72 It is generally accepted that their presence in the UK broadly followed sales figures from the main manufacturers (Monsanto Industrial Chemicals Company, USA) which increased from 1957 to peak sales in 1968–1970 and then declined in the 1970s (Fig. 7).73 Growing concern over the toxicity and possible carcinogenic effects led to increasing incremental UK regulation (1972 to 1977) before enclosed uses were highly restricted in 1981. Consequently, accepting a variety of caveats such as lag times from manufacture to environmental release, sedimentary mixing and erosion and post depositional decay the rise (1954), peak (1970) and fall (1980s) of PCBs can be used to date salt marsh and river-estuarine sediment cores.50,55,66 We therefore assign these dates to the rise-peak-fall of the PCB profiles shown in Fig. 7 and attribute the clear decline in concentrations in the upper sections (0–20 cm) of the cores to be due to a combination of improved disposal methods, greater import controls and manufacturing ban from 1977 to present day. This notion of declining absolute PCB concentrations supports earlier UK time series trends observed in soils and air from 1970s to mid-1990s in the UK.74,75
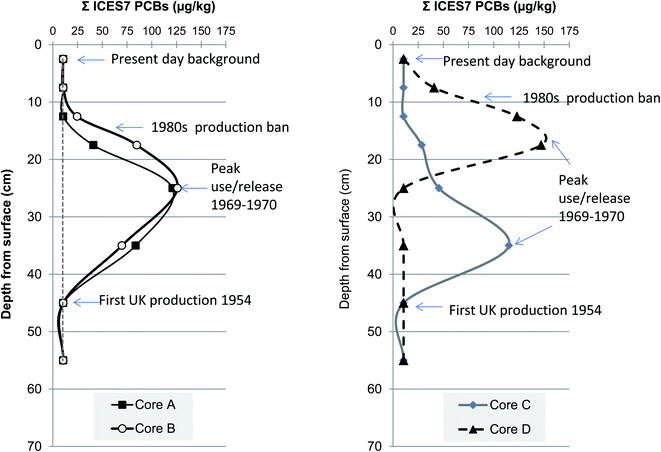 |
| Fig. 7 Vertical changes in polychlorinated biphenyls (PCB) concentration in four sediment cores from Chiswick Ait, River Thames. | |
In this current study the concentrations of PCBs (Σ ICES-7) in the Chiswick Ait cores (n = 32) ranged from 10.5 μg kg−1 to 121 μg kg−1 with a mean of 39.0 μg kg−1 (Fig. 7). These values are broadly comparable although higher on average than those reported for ICES-7 in mud-flat sediments within middle portion of the Thames estuary, Two Tree Island (n = 21) which ranged from 5–191 μg kg−1, mean of 12 μg kg−1.11 In contrast, sediment cores collected close to Tilbury docks have reported PCB concentrations of 39 μg kg−1 in the upper 582 cm (OD) and 309 μg kg−1 from 582 to 955 cm (OD).76 The abrupt step change in total PCB concentrations was interpreted to reflect local introduction of activated sewage treatment plant at Beckton and Crossness (1963–1974).76 More recently Lu and co-workers applied a fugacity model to predict the partitioning of chemicals between air–water–sediment phases in the Thames catchment.5 The fugacity models output(s) estimated that sediment concentrations of PCBs in Thames sediments were between 9 and 13 μg kg−1, a value well below the mean of all four cores examined herein, but still relatively close considering the complexity and size of the Thames catchment.5 The same study also hypothesised that Thames sediment would under increasing anthropogenic climate change pressures become an even more important sink and secondary source for PCBs as compared to atmosphere or water compartments.5 Whilst this current study is unable to substantiate this notion, because we only examine sediment and not water nor air counterpart matrices, the ability of tidal Thames sediment to host and act as long term reservoir for PCBs and presumably other persistent organic contaminants well above background is demonstrated (Fig. 7). If sediments of Chiswick Ait are considered soils rather than river-estuarine deposits then a comparison to London's urban soils is appropriate. Previous evaluation of persistent organic pollutants in east London's urban soils reported PCB (ICES-7) range from 1 to 750 μg kg−1 and mean of 21.4 μg kg−1.77 Therefore, the mean value observed here at Chiswick of 39 μg kg−1 are slightly higher than that of typical London soils; the narrower range of PCB concentrations observed at Chiswick maybe be simply due to the smaller number of samples evaluated (n = 44 to n = 74) or could be caused by differences in loading of water deposited particulates as compared to soil accumulating in different land-uses or mixing.77
Overall the results presented here suggest that some areas of the upper tidal Thames may contain a similar burden of chlorinated organic contamination as that in the lower more historically industrial reaches. Possible reasons include the concentrating effect and subsequent input from local STW, leaking land fill sites, local industries. upstream-freshwater releases or from former industrialised tributaries such as the Grand Union Canal which joins 5 km upstream at Thames Locks, Isleworth, London. The increase in total concentrations of PCB contaminated sediment/effluent sourced from connecting tributaries or canals has also been reported in the Clyde and Mersey. For example, a recent survey of persistent organic pollutants (POPs) in surface sediments from a 160 km stretch of the River Clyde, Scotland revealed the main source of PCBs were from Glasgow's urban tributaries and an earlier evaluation of sediments from the Mersey estuary showed elevated POPs concentrations at the confluence of the Manchester Ship canal at Eastham Locks.16,25,66 Comparison of absolute PCB concentrations presented here with other UK sediment surveys suggests that the upper Thames is similarly polluted. For example, the inner Clyde reported (ICES-7) ranged 1.20 to 76.8 μg kg−1, mean 25.8 μg kg−1, whereas those from the inner Mersey estuary (n = 22) 0.15–410 μg kg−1 and mean 33.8 μg kg−1 and Humber (n = 18) varied from 1 to 84 μg kg−1, mean 30.9 μg kg−1.66 However, the concentrations observed here at Chiswick Ait are lower than those of commercially or other strategically important European river-estuaries such as the Scheldt (Belgium) 105–400 μg kg−1 mean 220 μg kg−1, Seine (France) 500 to 3000 μg kg−1 Rhine (Germany), Rhône (France) ∼2 to 281 μg kg−1 but higher than sediments of the Danube (multiple countries) < 2 μg kg−1 and Volga (Russia) < 5 μg kg−1.78–83
3.7 Coupled or de-coupled toxicity test vs. chemical measurements
In order to understand the relationships between the chemical analysis data of the core material and the Microtox® data the combined data set was subjected to Principal Component Analysis (PCA) to reduce the dimensionality of the data (32 core samples 59 variables) into a smaller number of underlying features which should be more easily interpreted. The PCA was carried out in the R programming language using the base function “prcomp” in combination with the “factoextra” library for additional data analysis and visualisation. The data were mean centred and scaled to unit standard deviation prior to data analysis. The amount of variance explained by the first 10 principal components (PCs) is shown in the form of the scree plot in Fig. 8a. There is a clear “knee” in the scree plot at after 3 PCs which explain 87.3% (PC1 61.6%, PC2 14.3% and PC3 11.4%) of the variance in the data suggesting that there are three meaningful underlying PCs in this data set.
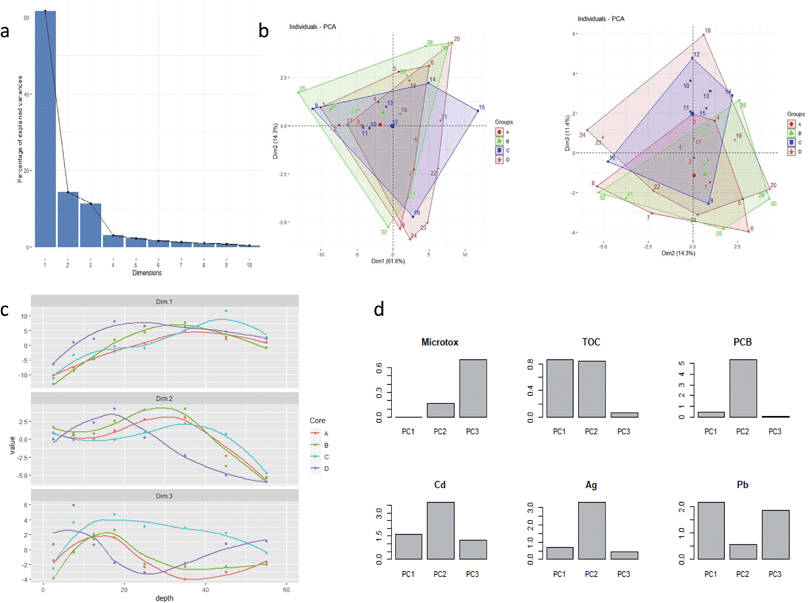 |
| Fig. 8 Panel: (a) amount of variance explained by the first 10 PCs; (b) score biplots of PC1 vs. PC2 and PC2 vs. PC3 with convex hulls around points belonging to the 4 core samples; (c) score depth profiles for the four core samples for PC dimensions 1–3; (d) percentage variance explained for selected variables between the three principal components. | |
Plotting the sample loadings in biplots of PC1 vs. PC2 and PC2 vs. PC3 with the data coloured to show the 4 different core samples (Fig. 8b) shows that core sample plot on top of one another suggesting there is no difference in the geochemical makeup of the 4 cores and that they are derived from the same geochemical sources. Plotting the scores for each PC for each core against depth provides information on how the three underlying features represented by the three PCs vary along the cores. Fig. 8c shows that the trends for each PC within each core are broadly the same: dimension 1 shows an increasing trend with depth; dimension 2 shows a peak value at ca. 30 cm for cores A, B and C whereas core D peaks at a shallower depth of ca. 18 cm; dimension 3 shows a maximum value between 0–20 cm for cores A, B and C with lower values at >20 cm although core D is again anomalous showing an increase at depth.
Having identified trends in the depth profiles for the three PC dimensions the PCA loadings can be used to calculate how much of the variance from each PC can be attributed to each geochemical variable and hence make some judgment the geochemical sources represented by the three PCSs. Fig. 8d shows the percent variance contributions of all variables to PC1. The variance of this PC is clearly dominated by the rare earth elements suggesting this may be a silty component of the core, most likely derived from Thames suspended particulate sediments (London Clay).7 The percent variance contributions of all variables to PC2 are presented in ESI.† The variance of this PC is clearly dominated by group II elements (Sr, Ca, Mg) suggesting is likely to be a carbonate rich component and PCBs are strongly associated with this component. Similarly, ESI† shows the percent variance contributions of all variables to PC3. High contributions from Cs, K and Rb suggest this component is likely to represent a clay source. Microtox is most strongly associated with this component.
3.8 Environmental pollution sediment quality
The Centre for Environment, Fisheries and Aquaculture (CEFAS) dredging disposal guidelines are widely utilised by UK marine and estuary managers as part of a multifaceted weight of evidence based approach to the management of the disposal of dredged material.84 These guidelines are based upon in-house and published laboratory and field studies that examined the ecological effects of a range of metals on multiple sensitive sediment-dwelling organisms; the criteria are similar but in some cases less conservative (higher) and other marine/estuarine sediment quality guidelines.85–87 For each trace metal two action levels are used which places them within one of three sediment management categories, namely, below action level 1 (AL1), no concern from a dredging and disposal standpoint, in-between action level 1 and 2, further assessment required and above action level 2 (AL2) possibly unsuitable for disposal at sea. However, before undertaking a benchmarking exercise it is important to note that in the UK there are no statutory sediment quality criteria and that the main guiding principal applied to sedimentary chemical pollution is that there is no deterioration from the current state for compounds/elements on the EC dangerous Substance List 1. This balanced, pragmatic approach avoids setting overly conservative criteria which would lead to non-compliance particularly in urban industrial stretches of UK river-estuary-coasts (e.g. Clyde, Thames, Mersey) that contain large inventories of legacy contamination.16
Benchmarking all sediments (n = 44) against CEFAS guideline values for the disposal of dredged material at sea designed to protect marine biota revealed that the majority of surface (0 to 20 cm) and basal (50 to 60 cm) sediments were of no immediate concern, whereas sediments from middle depths 20 to 50 cm exceeded AL2 (Fig. 3 and 4). Specifically, for Cu 35 intervals were between AL1 and AL2 criteria (68 to 373 mg kg−1, mean 171 mg kg−1), 9 intervals were below AL1 of no concern, and no intervals exceeded the upper Cu benchmark (400 mg kg−1). A different situation was apparent for Zn which exceeded AL2 12 times (839 to 1331 to mg kg−1, mean 1080 mg kg−1) and by definition are unsuitable for disposal at sea, whereas the remaining 32 sediments fell between AL1–AL2 (137–769 mg kg−1, mean 387 mg kg−1) further assessment suggested. Inspection of Pb concentrations showed that 17 intervals (524 to 1506 mg kg−1, mean 753 mg kg−1) exceeded AL2, 21 were intermediate to AL1–AL2 (80 to 447 mg kg−1, mean 223 mg kg−1) and four near surface samples were below AL1 meaning the Pb content was designated no immediate concern. No sediment from Chiswick Ait exceeded the Cr AL2 benchmark whereas 39 were between AL1–AL2 (40 to 351 mg kg−1, mean 172 mg kg−1) and five were beneath AL1, of no immediate concern. Regarding, Ni three intervals exceeded AL2 (104 to 107 mg kg−1, mean 105 mg kg−1), 21 spanned AL1–AL2 (2 to 89 mg kg−1, mean 51 mg kg−1) and 20 samples fell below AL1. In total 38 samples Ni concentrations fell between AL1–AL2 (37 to 113 mg kg−1 mean 70 mg kg−1) and five intervals near surface were below AL1 (11–19.8 mg kg−1, mean 14.6 mg kg−1).
Comparison of Chiswick Ait PCB sediment concentrations against appropriate CEFAS guideline values (ICES7) for the disposal of dredged material at Sea AL1 (only) 10 μg kg−1 and (sum 25 congeners) AL1, 20 μg kg−1 and AL2 200 μg kg−1 suggests that the lower 50–60 cm and the majority of surface (0 to 10 cm) of sediment falls below AL1 criteria whereas sediments in the middle depths (20–40 cm) exceed AL1 but do not exceed AL2 which would from a river management-dredging standpoint direct that additional testing is required.
3.9 Total pollution budget
The mean pollutant concentration within all four Chiswick Ait cores was Hg 4.6 mg kg−1, Cu 155.6 mg kg−1, Ni 62.7 mg kg−1, Cd 9.2 mg kg−1, Pb 402 mg kg−1, As 32.0 mg kg−1, Cr 181.2 mg kg−1 and PCB 39.6 μg kg−1. The total sediment volume of Chiswick Ait (0–60 cm) was 4.6841 × 10−6 km3 and we assumed sediment density of 1.5 Mg m−3 based on published proportions silt/clay for the upper tidal Thames7 and applied these to the equation mass = volume × density to give a sediment mass of 7
026
150 kg which gave a total mass of 0.28 kg PCB, Hg 32.5 kg, 1093 kg Cu, 440 kg, Ni, 2824 Cd, 2824 kg Pb, 224 kg As and 1273 kg Cr.
4. Conclusions
(1) This study demonstrates a combined radiogenic, heavy metal and organic pollutant approach to elucidating river-estuarine contaminant history over the past 80 years. Bulk C/N, isotopes δ13 and δ15N were inconclusive in terms of contaminant source apportionment but did indicate broad environmental input changes down core. Metals such as Cu, Zn, Cr, Ni, Cd exhibit a rise and peak 1960–1970 before a rapid decline in 1980s, whereas As, Pb and Hg had longer profiles suggesting, at least initially, different industrial source(s) emerging from before 1930–1940s. Total PCB profile differed from the heavy metals spanning narrower time-frame of the core reflecting on-set from mid-1950s to peak use and subsequent environmental release in 1970s to a graduated cessation of use in 2000s and different industrial sources. Although sometimes divergent the use of multiple contaminants with different sources, transport processes and chemical affinities as well as chemical event “take-off” points provides a more complete inventory of the pollution contained in the inner Thames sediments.
(2) Sediment toxicity (Microtox®) EC50 profiles did not consistently track heavy metal and or organic pollutant concentrations, this divergence is explained by: (1) synergistic and antagonistic interactions of the contaminants; (2) highly complex relationship between contaminant concentration and bioavailability and bioaccessibility and or; (3) presence of other contaminants not considered in this current studies analytical programme. These differences support a tiered bioassay, chemical measurement in situ biological community approach to sediment quality assessment in the Thames.
(3) To the best of our knowledge this is the first ever environmental geochemical assessment of Thames (London's) mud islands (Aits). Both heavy metal and organic pollutant profiles suggest that they serve as novel depositional environments within estuaries capturing a temporally consistent record of anthropogenic environmental pollution which is less mixed or prone to anthropogenic disturbance than adjacent foreshore and or channel bed sediments of the tidal Thames. Further, the uniform clay/silt sediment and consistent grain size as evidenced from (Al and Ti) provides an ideal medium to sorb contaminants. We speculate that London's river islands are not unique in this respect and suggest that other regularly flooded urban river islands may act as a similar sink/source for pollutants that are potentially harmful to biota and humans.
(4) We observed some of the highest silver (Ag) concentrations of any river-estuary in UK. These elevated concentrations probably occur as a result of anthropogenic input (sewage or other industrial sources) in combination with preferential accumulation due to Chiswick Ait's position close to the maximum salt water incursion.
(5) Chiswick Ait sediments were compared to English sediment quality guidelines regularly used as part of weight of evidence approach to the evaluation of coastal and estuarine sediments. Surface sediment heavy metal and PCB concentrations were below non-statutory guideline criteria and were therefore considered to be of no immediate concern. In contrast, Cu, Zn, Cr, Pb, As, Ni, Cd, Hg content at 25 to 35 cm depth frequently exceeded action level 2 suggesting that these were potentially unsuitable for disposal at sea since they could pose a risk to sediment dwelling biota. Older sediment intervals 40–45 cm were mainly below action level 1 or fall between these clear cut assessment criteria. Similarly, PCB concentrations between 10 and 45 cm depth were designated as intermediate to the upper and lower sediment quality values.
Conflicts of interest
There are no conflicts to declare.
Acknowledgements
This paper is published by permission of the Executive Director of the British Geological Survey, UKRI.
References
- A. P. Ganci, C. H. Vane, M. A.-E. Abdallah, T. Moehring and S. Harrad, Legacy PBDEs and NBFRs in sediments of the tidal River Thames using liquid chromatography coupled to a high resolution accurate mass Orbitrap mass spectrometer, Sci. Total Environ., 2019, 658, 1355–1366 CrossRef CAS PubMed.
- M. D. Jürgens, C. Chaemfa, D. Hughes, A. C. Johnson and K. C. Jones, PCB and organochlorine pesticide burden in eels in the lower Thames River (UK), Chemosphere, 2015, 118, 103–111 CrossRef PubMed.
-
W. J. Langston, P. E. Gibbs, P. L. Pascoe, B. S. Chesman, G. R. Burt, N. D. Pope and J. McEvoy, Tributyltin (TBT) Impact in the Thames Estuary, NERC, 2000 Search PubMed.
- R. A. Lopes dos Santos and C. H. Vane, Signatures of tetraether lipids reveal anthropogenic overprinting of natural organic matter in sediments of the Thames Estuary, UK, Org. Geochem., 2016, 93, 68–76 CrossRef CAS.
- Q. Lu, A. C. Johnson, M. D. Jürgens, A. Sweetman, L. Jin and P. Whitehead, The distribution of Polychlorinated Biphenyls (PCBs) in the River Thames Catchment under the scenarios of climate change, Sci. Total Environ., 2015, 533, 187–195 CrossRef CAS PubMed.
- M. A. Tye, J. Rushton and C. H. Vane, Distribution and speciation of phosphorus in foreshore sediments of the Thames estuary, Mar. Pollut. Bull., 2018, 127, 182–197 CrossRef PubMed.
- C. H. Vane, D. J. Beriro and G. H. Turner, Rise and Fall of Mercury (Hg) Pollution in Sediment Cores of the Thames Estuary, London, UK, Earth Environ. Sci. Trans. R. Soc. Edinburgh, 2015, 105, 285–296 CrossRef CAS.
- C. Nwokoro, C. Ewin, C. Harrison, M. Ibrahim, I. Dundas, I. Dickson, N. Mushtaq and J. Grigg, Cycling to work in London and inhaled does of black carbon, Eur. Respir. J., 2012, 40, 1091–1097 CrossRef CAS PubMed.
- D. Murray, P. Dempsey and P. Lloyd, Copper in the Thames Estuary in relation to the special protection areas, Hydrobiologia, 2011, 672, 39–47 CrossRef CAS.
- N. D. Pope and W. J. Langston, Sources, distribution and temporal variability of trace metals in the Thames Estuary, Hydrobiologia, 2011, 672, 49–68 CrossRef CAS.
- M. D. Scrimshaw, Organochlorine contamination in sediments of the inner Thames Estuary, J. Chart. Inst. Water Environ. Manage., 1995, 9, 519–525 CrossRef CAS.
-
M. Littlewood and M. Crossman, Planning for flood risk management in the Thames estuary, Environment Agency, 2003 Search PubMed.
- R. J. Uncles and S. B. Mitchell, Turbidity in the Thames Estuary: How turbid do we expect it to be?, Hydrobiologia, 2011, 672, 91–103 CrossRef CAS.
-
C. Lane, S. Surman-Lee, J. Sellwood and J. V. Lee, The Thames recreational users study, City of London Port Authority and Helath Protection Agency, 2007 Search PubMed.
- D. J. Beriro, C. H. Vane, M. R. Cave and C. P. Nathanail, Effects of drying and comminution type on the quantification of Polycyclic Aromatic Hydrocarbons (PAH) in a homogenised gasworks soil and the implications for human health risk assessment, Chemosphere, 2014, 111, 396–404 CrossRef CAS PubMed.
- C. H. Vane, R. A. Lopes dos Santos, A. W. Kim, V. Moss-Hayes, F. M. Fordyce and J. M. Bearcock, Persistent organic pollutants (PAH, PCB, TPH) in freshwater, urban tributary and estuarine surface sediments of the River Clyde, Scotland, UK, Earth Environ. Sci. Trans. R. Soc. Edinburgh, 2019, 108, 299–314 CrossRef CAS.
- K. R. Beg and S. Ali, Toxicity of reference sediment samples, Am. J. Environ. Sci., 2008, 4, 347–352 CrossRef CAS.
- R. Guerra, A. Pasteris, M. Ponti, D. Fabbri and L. Bruzzi, Impact of dredging in a shallow coastal lagoon: Microtox® Basic Solid-Phase Test, trace metals and Corophium bioassay, Environ. Int., 2007, 33, 469–473 CrossRef CAS PubMed.
- K. K. Kwan and B. J. Dutka, Comparative assessment of two solid phase toxicity bioassays: The direct sediment toxicity testing procedure (DSTTP) and the Microtox solid phase test (SPT), Bull. Environ. Contam. Toxicol., 1995, 55, 338–346 CrossRef CAS PubMed.
- A. L. Lamb, C. H. Vane, G. P. Wilson, J. G. Rees and V. L. Moss-Hayes, Assessing δ13C and C/N ratios from organic material in archived cores as Holocene sea level and palaeoenvironmental indicators in the Humber Estuary, UK, Mar. Geol., 2007, 244, 109–128 CrossRef.
- A. C. Kemp, C. H. Vane, N. S. Khan, J. C. Ellison, S. E. Engelhart, B. P. Horton, D. Nikitina, S. R. Smith, L. J. Rodrigues and R. P. Moyer, Testing the Utility of Geochemical Proxies to Reconstruct Holocene Coastal Environments and Relative Sea Level: A Case Study from Hungry Bay, Bermuda, Open Quat., 2019, 5, 1–17 CrossRef.
- A. C. Kemp, C. K. Sommerfield, C. H. Vane, B. P. Horton, S. Chenery, S. Anisfeld and D. Nikitina, Use of lead isotopes for developing chronologies in recent salt-marsh sediments, Quat. Geochronol., 2012, 12, 40–49 CrossRef.
-
S. A. Wilson, The collection, preparation and testing of USGS reference material BCR-2, Columbia River, Basalt, U.S. Geological Survey Open-File Report 98-00x., 1997 Search PubMed.
-
E. A. Mackey, S. J. Christopher, R. M. Lindstrom, S. E. Long, A. F. Marlow, K. E. Murphy, R. L. Paul, R. S. Popelka-Filcoff, S. A. Rabb, J. R. Sieber, R. O. Spatz, B. E. Tomlin, L. J. Wood, L. L. Yu, R. Zeisler, J. H. Yen, S. A. Wilson, M. G. Adams, Z. A. Brown, P. L. Lamothe, J. E. Taggart, C. Jones and J. Nebelsick, Certification of Three NIST Renewal Soil Standard Reference Materials for Element Content: SRM 2709a San Joaquin Soil, SRM 2710a Montana Soil I, and SRM 2711a Montana Soil II, U.S. Department of Commerce, 2010 Search PubMed.
- C. H. Vane, V. Moss-Hayes, A. W. Kim, K. E. Edgely, M. R. Cave and J. M. Bearcock, Mercury, n-alkane and unresolved complex mixture hydrocarbon pollution in surface sediment across the rural-urban-estuarine continuum of the River Clyde, Scotland, UK, Earth Environ. Sci. Trans. R. Soc. Edinburgh, 2019, 108, 315–326 CrossRef CAS.
- S. N. Khan, C. H. Vane, B. P. Horton, C. Hillier, J. B. Riding and C. Kendrick, The application of δ13C, TOC and C/N geochemistry to reconstruct Holocene relative sea levels and paleoenvironments in the Thames Estuary, UK, J. Quat. Sci., 2015, 30, 417–433 CrossRef.
-
N. S. Khan, C. H. Vane, S. E. Engelhart, C. P. Kendrick and B. P. Horton, The application of δ13C, TOC and C/N geochemistry of mangrove sediments to reconstruct Holocene paleoenvironments and relative sea levels, Puerto Rico, Mar. Geol., 2019, 415, 105963 Search PubMed.
- Y. Milker, B. P. Horton, C. H. Vane, S. E. Engelhart, A. R. Nelson, R. C. Witter, N. S. Khan and W. T. Bridgeland, Annual and seasonal distribution of intertidal foraminifera and stable carbon isotope geochemistry, Bandon Marsh, Oregon, USA, J. Foraminiferal Res., 2015, 45, 146–166 CrossRef.
- L. A. Bristow, T. D. Jickells, K. Weston, A. Marca-Bell, R. Parker and J. E. Andrews, Tracing estuarine organic matter sources into the southern North Sea using C and N isotopic signatures, Biogeochemistry, 2013, 113, 9–22 CrossRef CAS.
- J. J. Middelburg and J. Nieuwenhuize, Carbon and nitrogen stable isotopes in suspended matter and sediments from the Schelde Estuary, Mar. Chem., 1998, 60, 217–225 CrossRef CAS.
- A. C. Cabral, M. M. Wilhelm, R. C. L. Figueira and C. C. Martins, Tracking the historical sewage input in South American subtropical estuarine systems based on faecal sterols and bulk organic matter stable isotopes (δ13C and δ15N), Sci. Total Environ., 2019, 655, 855–864 CrossRef CAS PubMed.
- J. E. Andrews, A. M. Greenaway and P. F. Dennis, Combined Carbon Isotope and C/N Ratios as Indicators of Source and Fate of Organic Matter in a Poorly Flushed, Tropical Estuary: Hunts Bay, Kingston Harbour, Jamaica, Estuarine, Coastal Shelf Sci., 1998, 46, 743–756 CrossRef CAS.
- J. Tucker, N. Sheats, A. E. Giblin, C. S. Hopkinson and J. P. Montoya, Using stable isotopes to trace sewage-derived material through Boston Harbor and Massachusetts Bay, Mar. Environ. Res., 1999, 48, 353–375 CrossRef CAS.
- C. Savage, P. R. Leavitt and R. Elmgren, Effects of land use, urbanization, and climate variability on coastal eutrophication in the Baltic Sea, Limnol. Oceanogr., 2010, 55, 1033–1046 CrossRef CAS.
- S. Vaalgamaa, E. Sonninen, A. Korhola and K. Weckström, Identifying recent sources of organic matter enrichment and eutrophication trends at coastal sites using stable nitrogen and carbon isotope ratios in sediment cores, J. Paleolimnol., 2013, 50, 191–206 CrossRef.
- V. Gälman, J. Rydberg, S. Sjöstedt de-Luna, R. B. Bindler and I. Renberg, Carbon and nitrogen loss rates during aging of lake sediment: Changes over 27 years studied in varved lake sediment, Limnol. Oceanogr., 2008, 53, 1076–1082 CrossRef.
- J. H. Lacey, M. J. Leng, C. H. Vane, A. D. Radbourne, H. Yang and D. B. Ryves, Assessing human impact on Rostherne Mere, UK, using the geochemistry of organic matter, Anthropocene, 2018, 21, 52–65 CrossRef.
- J. I. Hedges and R. G. Keil, Organic geochemical perspectives on estuarine processes: sorption reactions and consequences, Mar. Chem., 1999, 65, 55–65 CrossRef CAS.
- J. I. Hedges, R. G. Keil and R. Benner, What happens to terrestrial organic matter in the ocean?, Org. Geochem., 1997, 27, 195–212 CrossRef CAS.
- K. Łukawska-Matuszewska, D. Burska and E. Niemirycz, Toxicity Assessment by Microtox® in Sediments, Pore Waters and Sediment Saline Elutriates in the Gulf of Gdansk (Baltic Sea), Clean, 2009, 37, 592–598 Search PubMed.
- E. Niemirycz, J. Nichthauser, M. Staniszewska, G. Nałęcz-Jawecki and J. Bolałek, The Microtox® biological test: Application in toxicity evaluation of surface waters and sediments in Poland, Oceanol. Hydrobiol. Stud., 2007, 36, 151–163 CAS.
-
C. G. Ingersoll, D. D. Macdonald, B. T. Brumbaugh, T. B. Johnson, N. E. Kemble, J. L. Kunz, T. W. May, N. Wang, J. R. Smith, D. W. Sparks and D. S. Ireland, Toxicity Assessment of Sediments from the Grand Calumet River and Indiana Harbor Canal in Northwestern Indiana, USA, 2002 Search PubMed.
- R. Uribe-Hernández, M. A. Montes de Oca-García, M. A. Amezcua-Allieri, J. A. Zermeño-Eguía Lis and V. E. Martínez-Martínez, Ecotoxicity assessment of weathered waste oil in a Mexican wetland, J. Pet. Sci. Res., 2012, 1, 26–31 Search PubMed.
- A. H. Ringwood, M. E. DeLorenzo, P. E. Ross and A. F. Holland, Interpretation of microtox solid-phase toxicity tests: the effects of sediment composition, Environ. Toxicol. Chem., 1997, 16, 1135–1140 CrossRef CAS.
- M. R. Cave, C. H. Vane, A. Kim, V. L. Moss-Hayes, J. Wragg, C. L. Richardson, H. Harrison, C. Paul Nathanail, R. Thomas and G. Wills, Measurement and modelling of the ingestion bioaccessibility of polyaromatic hydrocarbons in soils, Environmental Technology & Innovation, 2015, 3, 35–45 Search PubMed.
- M. R. Cave, J. Wragg, D. J. Beriro, C. Vane, R. Thomas, M. Riding and C. Taylor, An overview of research and development themes in the measurement and occurrences of polyaromatic hydrocarbons in dusts and particulates, J. Hazard. Mater., 2018, 360, 373–390 CrossRef CAS PubMed.
- W. J. Langston, G. R. Burt, B. S. Chesman and C. H. Vane, Partitioning, bioavailability, and effects of oestrogens and xeno-oestrogens in the aquatic environment, J. Mar. Biol. Assoc. U. K., 2005, 85, 1–30 CrossRef CAS.
- J. G. Farmer, L. J. Eades, H. Atkins and D. F. Chamberlain, Historical Trends in the Lead Isotopic Composition of Archival Sphagnum Mosses
from Scotland (1838–2000), Environ. Sci. Technol., 2002, 36, 152–157 CrossRef CAS PubMed.
- J. R. Bacon, K. C. Jones, S. P. McGrath and A. E. Johnston, Isotopic Character of Lead Deposited from the Atmosphere at a Grassland Site in the United Kingdom Since 1860, Environ. Sci. Technol., 1996, 30, 2511–2518 CrossRef CAS.
- C. H. Vane, S. R. Chenery, I. Harrison, A. W. Kim, V. M. Moss-Hayes and D. G. Jones, Chemical signatures of the Anthropocene in the Clyde estuary, UK: sediment-hosted Pb, 207/206Pb, total petroleum hydrocarbons, polyaromatic hydrocarbon and polychlorinated biphenyl pollution records, Philos. Trans. R. Soc., A, 2011, 369, 1085–1111 CrossRef CAS PubMed.
-
P. G. Appleby, in Tracking environmental change using lake sediments: Basin analysis, coring and chronostratigraphic techniques, ed. W. M. Last and J. P. Smol, Kluwer Academic Publishers, Dordrecht, 2001, vol. 1, pp. 171–203 Search PubMed.
- D. Jones, C. H. Vane, S. Lass-Evans, S. Chenery, B. Lister, M. Cave and I. Wilkinson, Geochemistry and related studies of Clyde Estuary sediments, Earth Environ. Sci. Trans. R. Soc. Edinburgh, 2019, 108, 269–288 CrossRef CAS.
- C. H. Vane, D. G. Jones and T. R. Lister, Mercury Contamination in Surface Sediments and Sediment Cores of the Mersey Estuary, UK, Mar. Pollut. Bull., 2009, 58, 928–946 CrossRef PubMed.
- S. V. Lee and A. B. Cundy, Heavy Metal Contamination and Mixing Processes in Sediments from the Humber Estuary, Eastern England, Estuarine, Coastal Shelf Sci., 2001, 53, 619–636 CrossRef CAS.
- W. M. Fox, M. S. Johnson, S. R. Jones, R. T. Leah and D. Copplestone, The use of sediment cores from stable and developing salt marshes to reconstruct historical contamination profiles in the Mersey Estuary, UK, Mar. Environ. Res., 1999, 47, 311–329 CrossRef CAS.
- M. J. Clark and F. B. Smith, Wet and dry deposition of Chernobyl releases, Nature, 1988, 3332, 245–249 CrossRef PubMed.
-
K. Vincent and N. Passant, Assessment of heavy metal concentrations in the United Kingdom, 2013 Search PubMed.
- P. Wright and C. F. Mason, Spatial and Seasonal Variation in Heavy Metals in the Sediments and Biota of Two Adjacent Estuaries, the Orwell and the Stour, in Eastern England, Sci. Total Environ., 1999, 226, 139–156 CrossRef CAS PubMed.
- G. W. Bryan and W. J. Langston, Bioavailability, accumulation and effects of heavy-metals in sediments with special reference to the United Kingdom estuaries - A review, Environ. Pollut., 1992, 76, 89–131 CrossRef CAS PubMed.
- S. N. Luoma, Y. B. Ho and G. W. Bryan, Fate, bioavailability and toxicity of silver in estuarine environments, Mar. Pollut. Bull., 1995, 31, 44–54 CrossRef CAS.
- G. S. Sanders and G. R. Abe, The role of suspended sediments and phytoplankton in the partitioning and transport of silver in estuaries, Cont. Shelf Res., 1987, 7, 1357–1361 CrossRef.
-
M. D. Jürgens, A. C. Johnson, A. J. Lawlor, S. A. Thacker, R. J. Williams, M. G. Pereira, C. L. Barnett and N. A. Beresford, Assessment of the current exposure of the British natural environment to silver (CB0464): Silver in sewage sludge, soil and river bed-sediments, Centre for Ecology and Hydrology, 2014 Search PubMed.
- E. A. Thomson, S. N. Luoma, C. E. Johansson and D. J. Cain, Comparison of sediments and organisms in identifying sources of biologically available trace metal contamination, Water Res., 1984, 18, 755–765 CrossRef CAS.
- A. J. Plater, J. Ridgway, P. G. Appleby, A. Berry and M. R. Wright, Historical contaminant fluxes in the Tees estuary, UK: Geochemical, magnetic and radionuclide evidence, Mar. Pollut. Bull., 1998, 37, 343–360 CrossRef CAS.
- J. Ridgway and G. Shimmield, Estuaries as Repositories of Historical Contamination and their Impact on Shelf Seas, Estuarine, Coastal Shelf Sci., 2002, 55, 903–928 CrossRef CAS.
- C. H. Vane, I. Harrison and A. W. Kim, Polycyclic aromatic hydrocarbons (PAHs) and polychlorinated biphenyls (PCBs) in sediments from the Mersey Estuary, U.K, Sci. Total Environ., 2007, 374, 112–126 CrossRef CAS PubMed.
-
J. Ridgway, E. Bee, N. Breward, M. Cave, S. Chenery, C. Gowing, I. Harrison, E. Hodgkinson, B. Humphreys, M. Ingham, A. Jarrow, G. Jenkins, A. Kim, R. Lister, A. Milodowski, S. Pearson, K. Rowlands, B. Spiro, M. Strutt and C. Vane, The Mersey Estuary: Sediment geochemistry, British Geological Survey, 2006 Search PubMed.
- D. T. Williams and F. M. Benoit, The determination of polychlorinated biphenyls in selected household products, Bull. Environ. Contam. Toxicol., 1979, 21, 179–184 CrossRef CAS PubMed.
-
M. Kuratsune and Y. Masuda, Polychlorinated biphenyls in non-carbon copy paper, Environmental Health Perspectives, 1972, pp. 61–62 Search PubMed.
- M. Kohler, J. Tremp, M. Zennegg, C. Seiler, S. Minder-Kohler, M. Beck, P. Lienemann, L. Wegmann and P. Schmid, Joint sealants: an overlooked diffuse source of polychlorinated biphenyls in buildings, Environ. Sci. Technol., 2005, 39, 1967–1973 CrossRef CAS PubMed.
- M. Andersson, R. T. Ottesen and T. Volden, Building materials as a source of PCB pollution in Bergen, Norway, Sci. Total Environ., 2004, 325, 139–144 CrossRef CAS PubMed.
- S. Harrad, A. P. Stuart, R. Alcock, R. Boumphrey, V. Burnett, R. Duarte-Davidson, C. Halsal, G. Sanders, K. Waterhouse, S. R. Wild and K. C. Jones, Polychlorinated biphenyls (PCBs) in the British environment: sinks, sources and temporal trends, Environ. Pollut., 1994, 85, 131–146 CrossRef CAS PubMed.
-
R. L. Durfee, G. Contos, F. C. Whitmore, J. D. Bardin, E. E. Hickman III and R. A. Westin, PCBs in the United States-Industrial use and environmental distribution, United States Environment Protection Agency, Washington, D.C., 1976 Search PubMed.
- K. C. Jones, G. Sanders, S. R. Wild, V. Burnett and A. E. Johnston, Evidence for the decline in PCBs and PAHs in rural UK vegetation and air, Nature, 1992, 356, 137–140 CrossRef CAS.
- R. Alcock, A. E. Johnston, S. P. McGrath, M. L. Berrow and K. C. Jones, Long-term changes in the polychlorinated biphenyl content of United Kingdom soils, Environ. Sci. Technol., 1993, 27, 1923–1948 CrossRef.
- M. D. Scrimshaw and J. N. Lester, Estimates of the inputs of polychlorinated biphenyls and organochlorine insecticides to the River Thames derived from the sediment record, Philos. Trans. R. Soc., A, 1997, 355, 189–212 CrossRef.
- C. H. Vane, A. W. Kim, D. J. Beriro, M. R. Cave, K. Knights, V. Moss-Hayes and P. C. Nathanail, Polycyclic aromatic hydrocarbons (PAH) and polychlorinated biphenyls (PCB) in urban soils of Greater London, UK, Appl. Geochem., 2014, 51, 303–314 CrossRef CAS.
- A. Covaci, C. Hura and P. Schepens, Selected persistent organochlorine pollutants in Romania, Sci. Total Environ., 2001, 280, 143–152 CrossRef CAS PubMed.
- A. Covaci, A. Gheorghe, S. Voorspoels, J. Maervoet, E. Steen Redeker, R. Blust and P. Schepens, Polybrominated diphenyl ethers, polychlorinated biphenyls and organochlorine pesticides in sediment cores from the Western Scheldt river (Belgium): analytical aspects and depth profiles, Environ. Int., 2005, 31, 367–375 CrossRef CAS PubMed.
- A. Covaci, A. Gheorghe, O. Hulea and P. Schepens, Levels and distribution of organochlorine pesticides, polychlorinated biphenyls and polybrominated diphenyl ethers in sediments and biota from the Danube Delta, Romania, Environ. Pollut., 2006, 140, 136–149 CrossRef CAS PubMed.
- S. Carpentier, R. Moilleron, C. Beltran, D. Herve and D. Thevenot, Quality of dredged material in the river Seine basin (France). II. Micropollutants, Sci. Total Environ., 2002, 299, 57–72 CrossRef CAS PubMed.
- H. J. Winkels, S. B. Kroonenberg, M. Y. Lychagin, G. Marin, G. V. Rusakov and N. S. Kasimov, Geochronology of priority pollutants in sedimentation zones of the Volga and Danube delta in comparison with the Rhine delta, Appl. Geochem., 1998, 13, 581–591 CrossRef CAS.
- M. Desmet, B. Mourier, B. J. Mahler, P. C. Van Metre, G. Roux, H. Persat, I. Lefèvre, A. Peretti, E. Chapron, A. Simonneau, C. Miège and M. Babut, Spatial and temporal trends in PCBs in sediment along the lower Rhône River, France, Sci. Total Environ., 2012, 433, 189–197 CrossRef CAS PubMed.
-
CEFAS, Monitoring and surveillance of non-radioactive contaminants in the aquatic environment and activities regulating the disposal of wastes at sea, 1995–1996, CEFAS, 1998 Search PubMed.
-
CCME, Canadian Council of Ministers of the Environment, 2002.
-
C. G. Ingersoll, D. D. MacDonald, N. Wang, J. L. Crane, L. J. Field, P. S. Haverland, N. E. Kemble, R. A. Lindskoog, C. Severn and D. E. Smorung, Prediction of sediment toxicity using consensus-based freshwater sediment quality guidelines, U.S.Environment Protection Agency, Chicago IL, USA, 2000 Search PubMed.
- E. R. Long, D. D. MacDonald, S. L. Smith and F. D. Calder, Incidence of adverse biological effects within ranges of chemical concentrations in marine estuarine sediments, Environ. Manage., 1995, 19, 81–97 CrossRef.
Footnote |
† Electronic supplementary information (ESI) available. See DOI: 10.1039/c9em00430k |
|
This journal is © The Royal Society of Chemistry 2020 |