DOI:
10.1039/D2QM00960A
(Review Article)
Mater. Chem. Front., 2023,
7, 44-64
Breakthroughs in nanozyme-inspired application diversity
Received
19th September 2022
, Accepted 22nd November 2022
First published on 22nd November 2022
Abstract
The rapid advancement of nanomaterials has promoted the booming of nanozymes capable of mimicking natural enzymes. As ideal alternatives to natural enzymes, nanozymes are characterized with a wide range of enzymatic activities, high stability, and easy modification. Recent biomedical application-based research has contributed to the unprecedented development of nanozymes, leading to a plethora of practical applications in nanozyme-based disease therapy. In this review, we firstly present the brief mechanisms and characteristics of noble metals, metal oxides, and other types of nanozymes. Subsequently, recent therapeutic advances of various nanozymes are summarized to investigate the extensive prospects in oncological and non-oncological fields. Finally, we discuss the future challenges in nanozyme-based therapeutic field, expecting to shed new light on nanozyme-oriented applications.
1. Introduction
The essential role of natural enzymes in biocatalytic functions has long been the mainstay in biological reactions.1 Acknowledging that enzymes are characterized by excellent catalytic and selective properties,2 however, certain intrinsic weaknesses, i.e., easy denaturation, complex preparation, economic unfriendliness, and recycling difficulties, hinder their potential applications.3 Nanozymes that combine “nanomaterial” with “enzyme”4 are described to be a family of nanomaterials with enzymatic properties that could physiologically exhibit enzyme-like reaction kinetics and catalytic mechanisms.5 After the discovery that Fe3O4 nanoparticles with catalytic activity resemble horseradish peroxidase (HRP),6,7 this terminology not only disrupted the long-standing perception of the biologically-inert characteristics of inorganic materials but also inaugurated the promising development of nanozyme-related research. To date, the highly eye-catching nanozymes have surmounted the deficiencies of natural enzymes and demonstrated unique enzyme-mimicking characteristics with high stability, friendly-economy, and high catalytic activity compared with traditional artificial enzymes,4,7,8 which enlighten the future utilization of nanozymes.4 Currently, the vast majority of nanomaterial libraries have been explored to demonstrate enzyme-like catalytic activities, i.e., peroxidase (POD), catalase (CAT), oxidase (OXD), superoxide dismutase (SOD), glutathione peroxidase (GPx), etc.,9 which provide extensive applications in various fields.10
Despite the intrinsic capability of imitating natural enzymes, nanozymes could also be modulated to further realize highly-efficient applications.11 Specifically, Liang et al. summarized the mechanisms of nanozymes and compared the advantages and disadvantages among nanozymes, natural enzymes, and nanomaterial-based catalysts, which have attracted attention in improving the practical applications of nanozymes.5 Besides, Wei et al. discussed different chemical modulations of nanozymes to activate their catalytic activity and selectivity in biomedical explorations.2 Moreover, Wu et al. introduced various types of different material-based nanozymes and their broad applications. They also outlined the clear current status of nanozymes with the analysis of current challenges, expecting to bring enlightenment in future investigations.12
The ideal catalytic activity of nanozyme-based strategies has been probed in the therapeutic management of diseases. The demand for novel therapeutic strategies remains urgent due to the current challenges of drug resistance, chronic toxicity, and poor targeting in disease treatment in the escalating exploitation of nanozymes in biomedical research. Despite these barriers, the potential of nanozymes for future biomedical therapies should not be overlooked or underestimated. Therefore, the study is focused on highlighting the latest therapeutic applications of nanozymes in oncologic therapy (including TME-oriented tumor therapy, synergistic therapy with PDT/PTT/SDT, and anti-tumor immunotherapy) and non-oncological related anti-inflammatory therapy, cardiovascular disease therapy, and nervous system disease therapy (Scheme 1). Subsequently, we summarized the current challenges of nanozymes and revealed future opportunities in the field of nanozyme-related therapeutics.
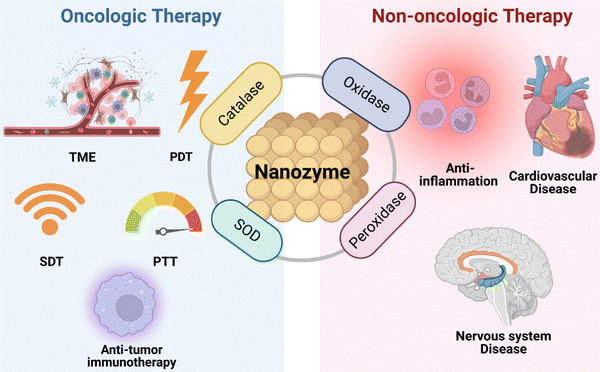 |
| Scheme 1 Schematic illustration related to this study. According to different treating orientations and modalities, nanozyme-based therapeutic applications are mainly categorized as oncologic/non-oncologic therapy in the present review. | |
2. Action mechanisms and classifications of nanozymes
Until now, numerous nanomaterials with enzyme-like functions have been found. Mostly, the composition of nanozymes includes noble metal nanomaterials, metal oxide nanomaterials, and other materials, which mainly exhibit four main catalytic properties such as peroxidase (POD), oxidase (OXD), catalase (CAT), and superoxide dismutase (SOD). Scientists have developed a number of methods to enhance their catalytic properties, enabling them to react with specific target molecules in a selective and efficient manner.
2.1 Noble metal nanomaterial
At present, metal nanozyme materials mainly consist of noble metals, including Au, Ag, Pt, Pd, Ir etc., as well as the combination of these metal–metallic materials that have already been extensively acknowledged in various applications due to their multi-enzymatic activities.13 Among them, the remarkable optical, electrical, and catalytic capabilities of gold nanostructures have sparked a great deal of attention during the rapid advancements in nanoscience and nanotechnology. For instance, the pH-shift enzyme-mimicking properties of Au{111} could induce the adsorption and breakdown of H2O2 at various pH levels. Besides, the outstanding optical and photothermal conversion capabilities, ease of synthesis, and multifunctional surface alterations of Au NPs have also made them a potential candidate for medical applications.14,15 Despite the fact that nanoscale gold particles have been shown to behave as catalysts for a variety of chemical reactions in various experimental setups, it is extensively believed that Au NPs larger than 10 nm are inert and safe for biological systems. For instance, after being taken up by cells, Au NPs are biocompatible and do not cause cytotoxicity or immunogenic effects.16 Additionally, researchers found that negatively charged and smaller Au NPs were more hazardous than positively charged and bigger Au NPs.17
Furthermore, other metals, including Ag, Pt, and Pd, showed very similar reaction pathways and pH-dependent enzymatic activity.18 The tunable catalytic properties of Ag are also exploited for biomedical applications. Previous studies hypothesized that the breakdown of Ag NPs coincided with the generation of hydroxyl radicals under acidic circumstances. On the other hand, the production of O2 in alkaline solutions containing H2O2 and Ag NPs showed that Ag NPs have CAT-like activity in the initial state.19 Additionally, the production of ROS and Ag NP dissolution have been suggested as the two main explanations for the toxicity brought on by Ag NPs. As excessive ROS vary in their oxidizing/reducing activities, identifying the types is crucial to understanding the toxicity of Ag NPs.
Recently, it was discovered that Pt nanoparticles exhibited activities similar to catalase, superoxide dismutase (SOD), peroxidase, and oxidase. The remarkable catalytic activity of nanosized Pt nanoparticles with a diameter of 2–4 nm is discovered by considering the size and shape-dependent characteristics. Small nanoparticles, on the other hand, are unstable and have the propensity to aggregate into larger clusters, and may lose their initial catalytic activity in the process. Until now, there have been numerous attempts to create innovative Pt nanostructures for catalytic applications that are more active and less expensive due to their small size, strong catalytic activity, and remarkable biocompatibility.20 Nonetheless, expensive template molecules (e.g., ferritin, lysozyme, or polyamidoamine dendrimer), strict control of reaction conditions, and complicated purification procedures were frequently required. Hence, the preparation process for these small-sized Pt-based nanozymes was typically laborious and time-consuming.
Owing to their intriguing characteristics and possible use as catalysts, palladium (Pd)-based nanomaterials have attracted increasing attention in recent years. Numerous attempts have been made to modify the size, shape, and structure of Pd nanoparticles in the field of catalytic oxidation reactions in order to achieve high catalytic efficiencies.21 In fact, it has been extensively used in catalyst design for oxidation processes or oxidation-related coupling reactions, and catalytic efficiency depends on these factors. However, the relationship between catalytic capacity and oxygen activation has somehow been disregarded. The solution to fully resolving the roles of Pd nanoparticles in numerous relevant applications, as well as to more logically design Pd-based nanomaterials for superior performance, lies in a basic comprehension of the mechanisms of oxygen reactivity and species generation.22
Based on its high activity, stability, and stereoselectivity, iridium-based catalyst has been acknowledged as an excellent contender in the field of catalysis. Among all the noble metal-based electrocatalysts, only iridium has been discovered to have high activity for both the oxygen reduction reaction (ORR) and the oxygen evolution reaction (OER) in acidic conditions.23 Iridium-based nanomaterials, specifically, with their high surface-to-volume ratio and high density of active sites, have demonstrated distinct physical and chemical properties from their bulk counterparts, which encourage research into the novel qualities and capabilities of iridium-based nanomaterials.24
2.2 Metal oxide nanomaterial
Ferromagnetic nanoparticles (Fe3O4) have been shown to possess intrinsic peroxidase-like activity, according to a 2007 study by Yan and colleagues.6 Their breakthrough opened up the subject of nanozyme, and a lot of intriguing research has since been done in the study of enzyme mimics made of metal oxide nanoparticles. Subsequently, a study used peroxidase mimics based on Fe3O4 MNP to detect both H2O2 and glucose.25 Iron oxide-based peroxidase mimics, including doped ferrites, Fe3O4 (magnetite), and Fe2O3 (hematite), were extensively researched and examined as a result of the groundbreaking study. It is acknowledged that ferromagnetic nanoparticles have good temperature tolerance and can function as catalysts over a wide pH range. Researchers later proved that size,26 morphology,27 and surface modification of Fe3O4 nanoparticles may also improve their catalytic activity.28
From the lanthanide series of the periodic table, cerium oxide is a rare earth oxide substance. It has previously been applied in a number of areas, including lengthening the lifespan of neuronal cultures and shielding cells from radiation harm. The electron shuttle between the mixed oxidation states of nanoceria (Ce3+ and Ce4+), which were among the first nanomaterials with SOD-mimicking properties to be discovered, and numerous investigations have demonstrated that greater Ce3+/Ce4+ ratios are associated with increased SOD-mimicking activity.29 Given the relationship between Ce3+ and oxygen vacancies, a method was used to ensure high Ce3+ by shrinking cerium oxide and increasing the number of surface oxygen vacancies. As a result, small cerium oxides—typically less than 5 nm in size–were thoroughly investigated as SOD mimics. Doping cerium oxide with Zr/La atoms would produce more oxygen vacancies, which could further improve the SOD-like activities.30,31 The stability, dispersity, and location of cerium oxide in a cellular environment were optimized for the biological application of cerium oxide by the introduction of multiple techniques, including surface coating and hydrogel formation.32,33
Mn oxides, such as MnO2, MnO, Mn2O3, Mn3O4, and MnOx, are chemically enhanced that can stay in the tumor site to modulate TME after reasonable nanosizing. For example, trans MNs can react with acid and exhibit responsive behavior in acidic TME.34 However, MnO2 without surface modification has poor structural stability under physiological settings and is challenging to synthesize with precise control over size and morphology, which raises questions about how reactive the nanomaterial will be. In order to create MnO2 nanoparticles with uniform shape, high stability, and biocompatibility for biomedical applications, this is extremely desirable.35,36
As oxidase mimics, copper-containing nanoparticles have also received a lot of attention. For instance, it has been reported that GOx-like Cu2O/polypyrrole composites can catalyze the oxidation of glucose to produce H2O2 under basic conditions (0.5 M NaOH). Although composites of Cu2O/polypyrrole with strong glucose oxidation activities made glucose detection possible, the reacting conditions still need to be improved to mimic a physiological setting for broader applicability. In an alkaline solution, the Cu2O/polypyrrole (Ppy) complex was shown to have the enzyme activity of glucose oxidase (GOx) and horseradish peroxidase (HRP). The persistent polypyrrole covering and high-index facets of the Cu2O octahedra were credited with the catalytic activity of this complex.37
2.3 Other materials
One of the promising iron-based nanozymes, prussian blue (PB) nanozymes, are alternately used to create cyanide-bridged cubic networks. Due to the strong affinity for cyanide ion binding of PB, it can travel through the body without harm. It is important to note that PB nanozymes are electron transporters that can imitate the activities of many enzymes, in contrast to the catalytic process that IONPs use to produce free radicals (e.g., POD, CAT, SOD, GPx, ascorbate oxidase, and ascorbate peroxidase). Besides, research also indicated that PB could function with POD-mimic properties under low pH conditions, while it mostly displays CAT-like activity when pH is higher, suggesting the pH-dependent feature of PB.38
Due to their high metal atomic utilization, superior specificity, and high catalytic activity, single-atom catalysts (SACs) have received a lot of attention in the field of heterogeneous catalysis. Recently, SACs, also known as single-atom nanozymes, have emerged as a new contender in the field of nanozymes due to their atomically distributed metal active cores (SAzymes).39 For conventional nanozymes, the activity mainly comes from few active sites on the surface of nanomaterials, which is often difficult to distinguish and quantitatively control, which not only makes the catalytic mechanism extremely complicated but also leads to a considerable difference in activity from that of natural enzymes. Numerous studies have shown that smaller sized nanozymes expose more catalytic active sites due to higher surface-to-volume ratios, thus increasing the catalytic activity. When nanoparticles shrink at the atomic level, they can exhibit not only the highest atomic utilization but also a simple atomic structure with well-defined coordination, active sites, catalytic mechanisms, and superior catalytic performance. SAzymes can fill the gap between homogeneous and multiphase catalysis and therefore break through the limitations of material design.40
The enzymatic activity of carbon-based nanomaterials has drawn significant attention among nonmetallic nanozymes, especially graphene quantum dots (GQD). The graphene quantum dot (GQD), the newest member of the graphene nanomaterial family, is a zero-dimensional graphene material with an extremely small size of under 10 nm and one or a few layers of graphene sheets. The GQD has demonstrated significant application potential in the disciplines of chemical/biological sensing, catalysis, and other areas because of its distinctive structural and optical features.41,42 Additionally, a large percentage of edge groups are produced by the amazing size of GQD. Consequently, the hydrophilic groups, such as oxygen-containing groups, around the margins of the GQD usually have extraordinary water solubility. In order to create functional composites, GQDs can also engage with functional small molecules through non-covalent interactions (such as electrostatic interactions and hydrophobic interactions). GQD is anticipated to work well as a matrix nanomaterial to aid in the dispersion of functional molecules in water due to its great biocompatibility and high stability of carbon compounds.43,44
3. Nanozyme in oncologic therapy
Currently, cancer has become one of the most inexorable human health killers globally.45 Conventional therapies such as chemotherapy and radiotherapy have long been considered effective. However, such treatments are also usually associated with severe side effects, causing poor patient compliance, and these deficiencies have hindered their further application in oncology therapy. Nanozymes, as inorganic materials employing their own enzymatic activity, have been demonstrated to be efficient in cancer therapy. On the one hand, based on generating excessive reactive oxygen species (ROS), nanozymes can strike a precise blow to tumor cells; on the other hand, it could catalyze the production of O2 by alleviating the hypoxic environment of the tumor, thus boosting the efficiency of cancer treatment.12 Recently, the applications of nanozymes in ameliorating the tumor microenvironment (TME) and enhancing photodynamic therapy (PDT), photothermal therapy (PTT), sonodynamic therapy (SDT), and immunotherapy have attracted more attention.
3.1 Nanozyme in TME related tumor therapy
Some nanozymes could exert POD and OXD-like activity in acidic TME, producing large amounts of ROS to destroy tumor cells. Based on this principle, once internalized in TME, these nanozymes may achieve corresponding antitumor efficacy. Inspired by this theory, Wang et al. reported a novel strategy to cure kidney tumors. In this work, the authors designed cobalt-doped Fe3O4 (Co@ Fe3O4) nanozyme to achieve enhanced POD-like activity. When Co@ Fe3O4 nanozyme was preferentially distributed in the acidic microenvironment of the lysosomes, the growth of human kidney carcinoma cells (A-498) was significantly inhibited due to the ROS burst induced by H2O2 decomposition. Consequently, the Co@ Fe3O4 nanozyme demonstrated desirable antitumor activities both in vitro and in vivo.46
With the understanding of the potential to exert enzymatic properties and modulate ROS in TME, Ali et al. established a cerium-based CeZrO4 nanoparticle that revealed the enzymatic activity of POD- and OXD. Encouragingly, the nanoparticle exhibited the best efficiency of ROS generation under acidic conditions (pH = 4) to manifest the most effective anti-tumor efficacy. Besides, highly reactive ROS generation demonstrated significant cancer cell death while causing minimal normal cell damage.47 Likewise, to raise the efficiency of ROS production, Yao et al. engineered a self-driven catalytic promotion system, TENG-CatSystem, to significantly inhibit the growth of 4T1 breast cancer cells, which not only realized a quadruple increase in POD mimetic properties but also achieved high efficiency in cancer therapy. More importantly, this TENG-CatSystem could serve as the safe electric pulse provider to reach four-folds POD-mimic properties without tissue impairment.48
Since intrinsic H2O2, glutathione (GSH), and hypoxia in TME may still hinder further applications of metallic nanozymes, the attempt to develop novel synthetic strategies is essential. Yang et al. thus constructed AuPt@SF (APS) through the combination of AuPt bimetallic nanozyme and silk fibroin (SF) to overcome TME restriction. By mimicking oxidase and peroxidase, the APS nanozyme could effectively convert excessive H2O2 into superoxide radicals (˙O2−) and hydroxyl radicals (˙OH). Besides, the consumption of GSH led to ROS depletion inhibition, which further achieved irreversible antitumor efficiency both in vitro and in vivo with desirable biosafety and minimal side effects.49
The combination strategy of nanozymes and natural enzymes has been extensively explored in TME-related tumor therapy.50 Wei et al. adopted ultra-small IrRu alloy nanoparticles (IrRu NPs) together with the natural enzyme GOx (IrRu-GOx@PEG NPs) to achieve dual enzyme activity for cancer treatment. Specifically, in the first phase, GOx catalyzed glucose into H2O2 to realize starvation therapy, thereby prohibiting tumor growth; while in the second phase, IrRu NPs catalyzed TME-associated H2O2 into singlet oxygen (1O2) for oxidative therapy, thus inducing apoptosis of cancer cells. Interestingly, the O2 generated in the second catalytic phase could significantly reverse hypoxia, which exerted a sustained effect of starvation therapy. Except for inhibiting tumor growth and lung metastasis, this synergistic strategy also accommodated desirable biocompatibility and safety in vivo through intravenous injection of IrRu-GOx@PEG NPs.51
Aiming to overcome the deficiency of low catalytic efficiency of nanozymes due to the deficit of H2O2, Yao et al. established a self-sustaining nanozyme bound to GOx, named as FePBG@GOx. The multi-layer structure of FePBG@GOx could exploit GOx to generate excessive H2O2, which further induced FePBG to generate huge toxic ˙OH to achieve apoptosis of tumor cells. As a promising candidate for future application, the biosafety of FePBG@GOx was identified. Results indicated blood samples of mice on the 1st and 7th day were normal, and histological outcome also suggested ideal biocompatibility. Compared with natural GOx, this multi-nanozyme strategy offers advantages such as responses for TME, self-supply, and easy degradation, which could provide evidence for further clinical applications (Fig. 1).52
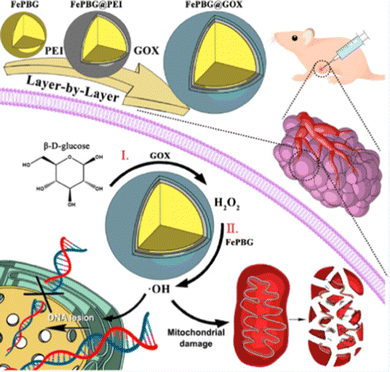 |
| Fig. 1 Schematic illustration of synthesis and mechanism of FePBG@GOx. Reprinted with permission.52 Copyright 2022 American Chemical Society. | |
3.2 Nanozyme in combined tumor therapy
Apart from inducing excessive ROS in TME to kill tumor cells, the CAT-mimic property of nanozymes could also yield O2 originating from intrinsic H2O2 to alleviate tumor hypoxia, providing a plausible way to effectively augment treating results, especially therapies that rely deeply on O2 supplement.53 Furthermore, given that monotherapy may not exert enough killing effort, novel treatments like photodynamic therapy (PDT), photothermal therapy (PTT), and sonodynamic therapy (SDT) have been combined with nanozyme and explorations are in progress. To make a clear overview of this section, the related parameters of PDT, PTT, and SDT are summarized (Table 1).
Table 1 Parameters related to nanozyme synergistic PDT/PTT/SDT therapies
Modality |
Nanozyme |
Size |
Irradiation source |
Intensity |
Time (min) |
Ref. |
PDT |
PLT-MnO2/DCPy (PMD) |
116 nm |
400–700 nm, white light |
0.1 W cm−2 |
20 |
54
|
CPT-TK-HPPH/Pt NP |
179.67 ± 2.45 nm |
660 nm, laser |
200 mW cm−2 |
5 |
55
|
Ce6/Ftn@MnO2 |
15.5 nm |
660 nm, laser |
25 mW cm−2 |
10 |
56
|
PTT |
Ti3C2Tx-Pt-PEG |
200 nm |
1064 nm, NIR |
0.75 W cm−2 |
5 |
57
|
PEG/Ce-Bi@DMSN |
Bi2S3@DMSN: 110.6 ± 18.6 nm; CeO2: 3–4 nm |
1064 nm, NIR |
1.25 W cm−2 |
10 |
60
|
PM-Fe-SAzyme (PMS) |
108 nm |
1064 nm, NIR |
0.5 W cm−2 |
5 |
62
|
SDT |
Pd/H-TiO2-PEG NSs |
61.9 nm |
1.0 MHz, US |
1.5 W cm−2 |
3 |
65
|
Pt@ZIF-90@Gem@IR780 (PZGI NPs) |
128.1–154.9 nm |
3 MHz, US |
0.1 W cm−2 |
5 |
67
|
Ang-IR780-MnO2-PLGA (AIMP) |
166.27 ± 1.50 nm |
US |
3 W cm−2 |
5 |
68
|
PEG-TiO1+xNRs |
28.68 ± 4.24 nm |
40 MHz, US |
3.0 W cm−2 |
5 |
69
|
CDP@HP-T |
250 nm |
US |
1.5 W cm−2 |
5 |
70
|
RT |
Au@MnS@ZnS |
110 nm |
X-Ray |
4 and 6 Gy |
— |
168
|
A-MnO2 NPs |
15 nm |
X-Ray |
10 Gy |
— |
170
|
P-RuCu |
6–12 nm |
X-Ray |
3 Gy |
— |
171
|
3.2.1 Nanozyme combined PDT.
PDT, as the promising therapeutic strategy, is featured with non-invasiveness and high selectivity. Undesirably, due to the intrinsic hypoxic condition of TME, the efficiency of PDT has been hindered. Recently, AIEgen has been explored for bioimaging and PDT-oriented ROS generation extensively. Since hypoxia may still be the major obstacle influencing the therapeutic efficiency of PDT, Duo et al. thus constructed MnO2 nanozyme/AIEgen composite and further coated it with platelet (PLT) membrane (PMD) for PDT in a hypoxic tumor environment. Due to the presence of PLT membrane, PMD was capable of targeting tumor vascular-deficient sites while avoiding macrophage mediated phagocytosis. In addition, PDT treating efficiency was later improved by MnO2-catalyzed O2 generation in mice with orthotopic colon tumors without obvious side effects.54
Prodrugs are a series of drugs with bioactivity and target releasing property upon stimulation. The ROS-responsive prodrug, especially, could not only realize such characteristics, but also achieve PDT activity as an excellent photosensitizer. Hao et al. created CPT-TK-HPPH/Pt NPs to achieve multi-treatment effects. Specifically, under 660 nm laser irradiation, the platinum nanozymes (PtNP) in this multifunctional prodrug could effectively catalyze H2O2 into O2, which not only enhanced the efficacy of PDT by alleviating hypoxia, but also ensured the simultaneous release of the chemotherapeutic drug CPT. With this refined engineering strategy, the growth of colon tumors was significantly inhibited both in vitro and in vivo. Compared with the single application of CPT, CPT-TK-HPPH/Pt NP showed minimum side effects and good biocompatibility.55
MnO2 has been extensively acknowledged as a desirable O2 provider, while Ferritin (Ftn), renowned as the excellent delivery protein, has been proven to exert high affinity toward transferrin receptor 1 (TfR1) overexpressed in most tumor cells. To counteract the negative hypoxia in breast cancer, Zhu et al. formulated a PDT platform that delivers O2via Ce6, MnO2, and Ftn (Ce6/Ftn@MnO2). The small size (15.5 nm) and high stability of Ce6/Ftn@MnO2 exhibited exceptional biodegradability and biocompatibility. Furthermore, by breaking down endogenous H2O2 into O2, Ftn exhibited its excellent catalytic activity in this nanoplatform, which not only alleviated hypoxia by significantly reducing hypoxia-inducible factor (HIF)-1α, but also significantly enhanced the therapeutic efficiency of PDT. Due to its ideal distribution in vivo, Ce6/Ftn@MnO2 also revealed ideal fluorescence imaging and manganese resonance imaging capabilities56 (Fig. 2).
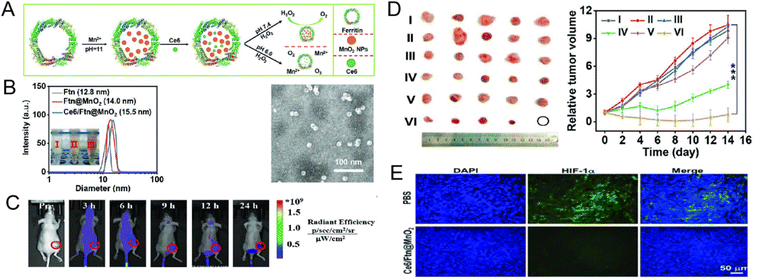 |
| Fig. 2 (A) Illustration of Ce6/Ftn@MnO2 synthesis. (B) Size of Ce6/Ftn@MnO2 both in DLS and TEM. (C) Tumor site accumulation of Ce6/Ftn@MnO2in vivo. (D) The exhibition of xenograft tumors and tumor volume growth ((I): PBS, (II): PBS + laser, (III): Ce6, (IV): Ce6 + laser, (V): Ce6/Ftn@MnO2). (E) Immunofluorescence images of hypoxia staining by DAPI (blue) and anti-HIF-1α antibody (green), respectively. DLS: dynamic light scattering; TEM: transmission electron microscope. Reprinted with permission.56 Copyright 2022 Wiley-VCH GmbH. | |
3.2.2 Nanozyme combined PTT.
Other than PDT, nanozymes could also be applied for PTT. A Ti-based MXene nanocomposite (Ti3C2Tx-Pt-PEG) was established by Zhu et al. to achieve amplification of PTT and nanozyme-based cancer treatment. In this work, Pt nanozyme exhibited its POD-like activity to catalyze H2O2 into toxic ˙OH for cancer cell death. Moreover, the efficiency of POD was significantly enhanced by the corresponding photothermal effect exerted by Ti3C2Tx, revealing an inspiring synergistic nanozyme strategy in combination with PTT. Additionally, according to the H & E staining results, such nanocomposite revealed no substantial toxicity or side effect in vivo, demonstrating its promising biosafety.57 Previous research indicated that nanozymes may sometimes suffer from unsatisfactory catalytic efficiency in tumor TME.58,59 Dong et al. developed a PEG/Ce-Bi@DMSN nanozyme system. As a result of dual properties of nanozymes (POD and CAT), this system was able to modulate TME by promoting oxidative stress, alleviating hypoxia, and depleting GSH. Moreover, the catalytic effect and tumor treatment efficiency of this dual nanozyme system was remarkably enhanced by the hyperthermia induced by PTT.60
Enhancement of PTT efficiency should be realized at relatively low temperatures to avoid inflammatory damage to proximal tissues.61 Noticing that the distinctive feature of multifunctional single-atom nanozyme (SAzyme) could be employed by its catalytic and photothermal activity, Qi et al. formulated a platelet membrane (PM)-coated Fe-SAzyme (PMS) to show the desirable photothermal performance with POD activity, minimal toxicity, and tumor targeting capability. Due to the PM coating, PMS not only demonstrated long-lasting blood circulation, but also effective mitochondria-targeted damage. Consequently, it could effectively improve PTT efficiency under mild temperatures, which was finally verified by suppressing tumor growth effectively (Fig. 3). In addition, both histopathology and blood biochemistry analysis were investigated, further confirming the good biosafety and biocompatibility of PMS in vivo.62
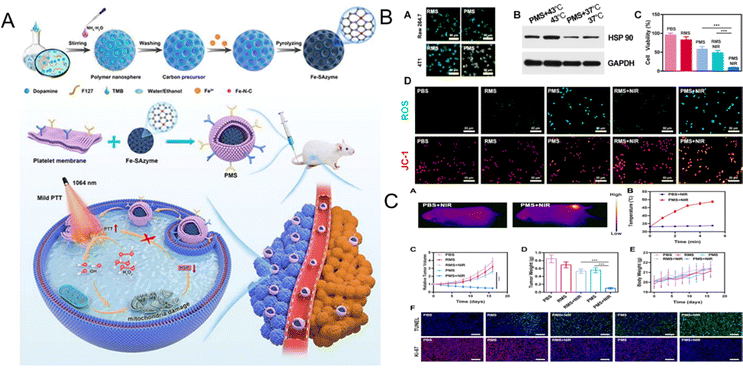 |
| Fig. 3 (A) Scheme of the working mechanisms of PMS in PTT. (B) The target ability, significant inhibition of HSP90 expression, the lowest cancer cell viability, and the generation of ˙OH and ROS of PMS with NIR. (C) The infrared thermal images under NIR exposure show the heat elevation at the tumor site, with remarkable tumor volume suppression and negligible weight change. PTT: photothermal therapy. NIR: near infrared. Reprinted with permission.62 Copyright 2022 American Chemical Society. | |
3.2.3 Nanozyme combined SDT.
Similar to PDT and PTT, the significance of SDT in tumor therapy is also boosting, owing to its encouraging deep-penetrating capability and non-irradiation,63 while limitation of ROS generation and hypoxic TME still plagued the further optimization of SDT.64,65 Qiao et al. engineered two-dimensional (2D) Pd/H-TiO2 nanosheets (NSs) to enhance the action of SDT in tumor therapy. By taking advantage of oxygen deficiency in TME, Pd/H-TiO2 NSs could be exploited to realize multifunctional duties, such as TiO2 as sonosensitizer, Ti3+ for Fenon-like activity, and Pd as nanozyme to generate hydroxyl radicals. Meanwhile, Pd in this special strategy was designed to contribute towards relieving hypoxia through its catalase-mimic property for O2 production to achieve desirable synergistic chemodynamic therapy (CDT) and SDT efficiency. Apart from the excellent tumor suppression result, the combined strategy was also verified with minimal toxicity in mice, indicating its desirable biosafety.65
Similarly, in pancreatic TME, hypoxia is often the cause of poor therapeutic outcomes.66 To reverse this dilemma, Chen et al. constructed PZGI nanoplatform with zeolitic imidazolate frameworks-90 (ZIF-90), Pt, gemcitabine (Gem), and IR780. Pt, in particular, has been employed to relieve hypoxia by its nanozyme property to catalyze excessive H2O2 into O2. Building on this advantage, the efficiency of SDT is significantly elevated by the simultaneous excess generation of cytotoxic singlet oxygen. In combination with the rapid-releasing of Gem, a first-line chemotherapeutic agent, this synergistic nanoplatform exerted excellent hypoxia-relieving and SDT-promoting efficacy. The final pathology result of major organs within 15 days suggested that PZGI NPs together with US could achieve safe pancreatic cancer treatment simultaneously.67
Except for hypoxia TME, the overexpression of GSH is considered one of the adverse factors that hinder SDT efficacy. Liu et al. established a multifunctional nanozyme by synthesizing IR780, MnO2, and Angiopep-2 (Ang) onto PLGA to acquire improved SDT performance guided by multimodal imaging modalities. Upon low-intensity focused ultrasound (LIFU) irradiation, MnO2 demonstrated its nanozyme activity by interacting with H2O2 and GSH, thus achieving satisfactory O2 production and GSH consumption, while sonosensitizer IR780 in this system functioned as the sono-amplifier with mitochondrial targeting capability to induce stronger cellular damage at the tumor site. In addition, in combination with magnetic resonance contrast agent Mn2+, the nanozyme system exhibited multiple imaging functions of magnetic resonance (MR), photoacoustics (PA), and fluorescence (FL). Furthermore, the enhanced SDT efficacy was achieved by suppressing glioblastoma growth and distal metastasis without obvious toxicity. Thus, this multifunctional nanozyme platform provided an all-in-one strategy for cancer diagnosis and treatment68 (Fig. 4).
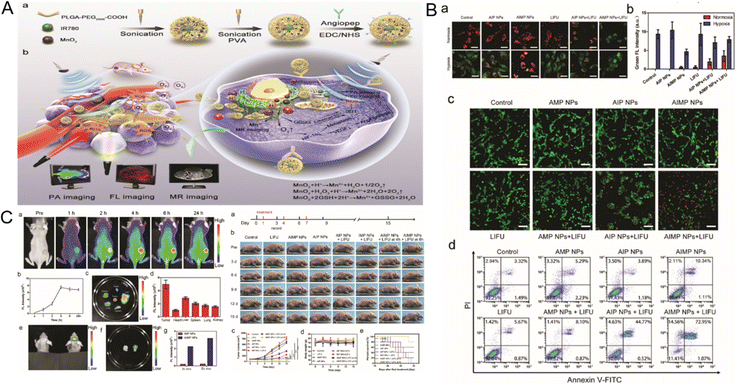 |
| Fig. 4 (A) Schematic illustration of the AIMP NPs synthesis and mechanism in tumor targeting, SDT efficiency enhancing and multimodal imaging. (B) Treating efficiency of AIMP NPs with LIFU exhibited by MMP expression and tumor cell apoptosis. (C) The distribution of AIMP and strengthened SDT efficiency in vivo. SDT: sonodynamic therapy. LIFU: low intensity focused ultrasound. MMP: mitochondrial membrane potential. Reprinted with permission.68 Copyright 2021 RSC Pub. | |
Aiming to improve the therapeutic sensitivity of SDT, Wang et al. fabricated ultrafine titanium monoxide nanorods (TiO1+x NRs) modified with polyethylene glycol (PEG). The novel structure not only exhibited excellent capability as the sonosensitizer upon ultrasound irradiation, but also revealed horseradish-peroxidase-mimic property. TiO1+x NRs can catalyze the formation of ˙OH from H2O2 in tumors, allowing for CDT and elevated SDT efficiency without inducing detectable side effects.69 Similarly, An et al. prepared a unique biodegradable nanoprobe CDP@HP-T to ameliorate the relatively inefficient SDT under hypoxic conditions.37 The nanoprobe, featured with pH sensitivity, could degrade Ce6 and DOX in acidic TME conditions. Besides, as a CAT-mimic catalyst, Pt in this nanoprobe may cause the decomposition of H2O2 into O2, reducing tumor hypoxia and further boosting the therapeutic efficacy of SDT. According to the in vitro and in vivo results, the probe was verified with great biocompatibility.70
3.2.4 Nanozyme combined RT.
Radiation therapy (RT) is an effective tumor treatment based on high-energy ionizing radiation (e.g., X-rays and γ-rays) interacting directly with cellular DNA leading to damage or indirectly reacts with H2O2 to produce ROS. As a classical method of cancer treatment, although RT has the special advantage of not being limited by tissue depth, its undesirable side effects and radiation resistance can greatly reduce the therapeutic efficiency toward tumor cells. One of the most preferred tactics is to normalize the TME in order to improve RT efficiency, improve hypoxia, and promote radiation-induced DNA damage. Numerous in vitro tests have shown that Au@MnS@ZnS-PEG nanoparticles can improve the cancer cell killing efficiency generated by RT. Li et al. demonstrated that Au@MnS@ZnS-PEG can be employed as a contrast agent for T1-weighted magnetic resonance imaging (MR), which indicates the effective accumulation and retention of nanoparticles in the tumors of mice following intravenous injection. Importantly, tumor growth can be considerably prevented by subjecting animals with tumors who received injections of Au@MnS@ZnSPEG to X-ray irradiation. When compared to RT alone, this finding clearly demonstrates improved treatment efficacy. Additionally, no overt negative effects of Au@MnS@ZnS-PEG are seen in the mice that were injected.168
Likewise, by stimulating the production of O2, a number of nanomaterials are intended to increase tumor radiation sensitivity and reduce hypoxia.169 The A-MnO2 NPs (constructed by albumin and MnO2 NPs) possess remarkable reactivity, stability, and selectivity towards H2O2, which allows them to simultaneously modify hypoxia and acidosis TME with controlled pH. Additionally, by downregulating hypoxia-inducible factor-1a (HIF-1a) and vascular endothelial growth factor (VEGF), A-MnO2 NPs could restore tumor blood vessels. Thus, it is possible to substantially improve the tumor response to radiation by utilizing the designed multifunctional A-MnO2 NPs.170
Hu et al. demonstrated that a bimetallic nanozyme with ultrasensitive peroxidase (POD)-like activity and catalase (CAT)-like activity—copper-modified ruthenium nanoparticles, or RuCu NPs—offers a perfect remedy to raise RT sensitization. When exposed to X-rays, RuCu NPs coated with PEG concurrently increased ROS generation and reduced tumor hypoxia in the acid TME and displayed astounding therapeutic activity in the MDA-MB-231 breast cancer model. This finding indicates the viability of an RT sensitization method that combines the intrinsic properties of high-Z elements with the benefits of nanozymes. Furthermore, in vivo results demonstrate the obvious superiority of P-RuCu along with intratumoral delivery in terms of efficient cancer treatment and biosafety, by not only ensuring the high accumulation in tumor region but also by leading to quick clearance.171
3.3 Nanozyme in anti-tumor immunotherapy
Recently, immunotherapy has attracted widespread interest in tumor therapy, among which modulation of immune checkpoint inhibitors (ICIs) is one of the most common strategies, mainly including cytotoxic T lymphocyte antigen 4 (CTLA4) and programmed cell death protein/programmed cell death ligand 1 (PD-1/PD-L1).71 Immune checkpoint blockade (ICB) therapies are often associated with adverse immune-related results; therefore, it is crucial to combine other advantageous therapies to auxiliary achieve immune cell death and anti-tumor immune responses.72–74 According to this strategy, He et al. prepared a complex nanozyme system by simultaneously synthesizing Pd, catechol-grafted carbon-quantum-dot (DA-CQD), and immune adjuvant CpGODN. Notably, DA-CQD@Pd single atom nanozyme (SAN) exerted a double catalytic mechanism by Pd and DA-CQD to catalyze H2O2 to ˙OH simultaneously. Additionally, DA-CQD@Pd@CpGODN enhanced the anticancer immune response and increased the effectiveness of immunotherapy by acting as a localized immunomodulation niche. Pd converted H2O2 into hydroxyl radicals, causing ICD in tumor cells and producing tumor-associated antigens in the tumor lysate that triggered an immune response against the tumor. Finally, this hydrogel significantly inhibited tumor recurrence and metastasis when combined with ICI anti-PD-L175 (Fig. 5).
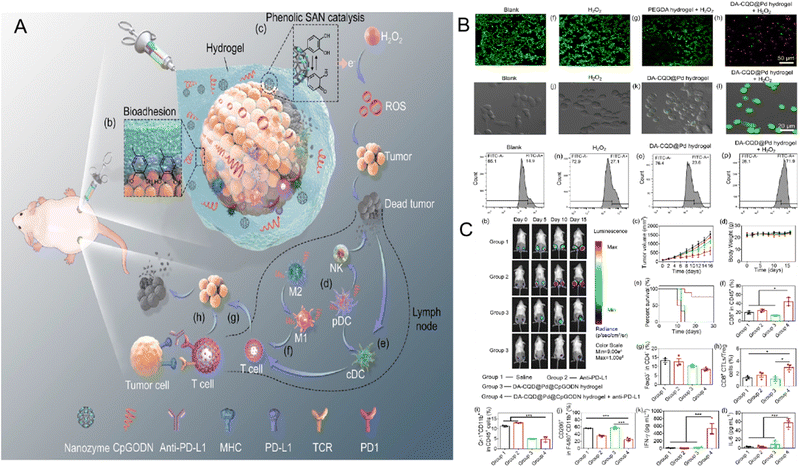 |
| Fig. 5 (A) schematic design and mechanism of DA-CQD@Pd@CpGODN in tumor therapy. (B) Live/dead staining and ROS generation results of 4T1 cells in different groups. (C) Treating efficiency of in vivo therapy combined with immunotherapy, results including bioluminescence imaging, tumor growth curves, mice body weights, survival time, and immune-related indexes in four groups (Saline, Anti-PD-L1, DA-CQD@Pd@CpGODN hydrogel, and DA-CQD@Pd@CpGODN hydrogel + anti- PD-L1). Reprinted with permission.75 Copyright 2021 Elsevier Ltd. | |
Chimeric antigen receptor T cells (CAR T cells) have been considered a promising antitumor combination in preclinical research. Nonetheless, tumor heterogeneity and immunosuppressive TME remain major impediments to the further implementation in solid tumors.76 Zhu et al. established an encouraging therapeutic strategy by combining tumor-specific HA@Cu2−xS-PEG (PHCN) nanozymes with B7-H3 CAR T therapy. It was highlighted that PHCN nanozymes not only exerted remarkable catalytic capability to induce ROS generation for tumor cell damage, but also served as the CAR T cell sensitizer to effectively inverse the immune-unfriendly TME, which in both strengthened the synergistic therapy. Compared with single CAR T cell therapy, the end results suggested this nanozyme-oriented strategy successfully maximized cancer elimination, and overall survival increased by the non-small cell lung cancer (NSCLC) mice model. The findings also suggested the negligible toxicity of PHCNs since no treatment-related side effects were found.77
Except for ROS-related therapeutic capacity, the relationship between nanozymes and anti-tumor immune response has been investigated profoundly.78 Zhao et al. combined GOx, nanozyme ferric protoporphyrin (hemin), and dihydroartemisinin (DHA) into zeolitic imidazolate framework (ZIF-8) altogether to form the nanozyme-initiated system denoted as GHZD NCs. This integration revealed POD, GOx, and GPx properties to realize glycolysis-related H2O2 supplement, ˙OH production, and GSH consumption. The latter two processes amplified oxidative stress, thus resulting in enhanced immunogenic cell death (ICD) and tumor suppression. This system effectively promoted anti-PD-L1 performance via modulating the immune response, which finally inhibited cancer growth and distant lung metastasis. Additionally, the survival period of mice in GHZD + anti-PD-L1 group was greatly extended, and no abnormality was detected, showing its great biosafety.79
4. Nanozyme in non-oncologic therapy
Excessive ROS in acidic TME would inhibit the proliferation of tumor cells, while overexpressed ROS under neutral conditions has been indicated to cause many adverse effects related to lipid, protein, DNA, and other biomolecules80 and is also believed to be tightly associated with corresponding diseases.81,82 Hence, it is well accepted that the regulation of ROS is of remarkable importance for maintaining intracellular redox homeostasis. Given the multiple catalytic activities and superior stability of nanozymes compared to natural enzymes, the excess ROS scavenging-based nanozymes are considered a promising alternative.
4.1 Nanozyme-related anti-inflammatory therapy
The inflammatory process is known as the body's defensive response to irritation and usually manifests as redness, swelling, fever, pain, and dysfunction. As a precursor to many other diseases, prompt management of inflammation could prevent the progression of related diseases. Recently, the emerging role of ROS-oriented nanozyme in anti-inflammatory therapy has been highlighted.83
Considering that inflammatory bowel disease (IBD) is greatly impacted by a pro-inflammatory environment dominated by excessive ROS, Wang et al. developed ZnMn2O4 (ZM) with SOD-mimic properties and later achieved CAT-mimetic and GPx-mimetic properties by doping with Li as optimized LiMn2O4 (LM). The multi-antioxidant property of LM was proved to ameliorate the progression of IBD both at the cellular level and animal level through the reduced dose.84 Furthermore, Yuan et al. noticed that ROS accumulation-induced inflammation is also the leading cause of acute lung injury (ALI). Accordingly, they developed nanoparticles synthesized by Fe and curcumin to exert ROS elimination through its catalytic activity. The strategy exhibited anti-inflammation properties by downsizing the inflammation-related cytokines and inhibiting the NF-κB pathway, which were further verified in mice models. Besides, by indicating the reduction of CD11bloF4/80hi and CD3+CD45+ T cells, this Fe-Cur NPs nanozyme was verified with no toxicity toward major organs and blood circulation to serve as a promising method in relieving ALI-induced inflammation cytokine storm.85
As ROS equilibrium is instrumental in the progression of inflammation, it is essential to reverse this dilemma by utilizing nanozyme-based strategies, of which CeONP has gained considerable attention due to its desirable catalytic properties.86,87 Based on this, Kim et al. optimized the CeONP to improve the renal metabolism of this nanozyme. In the study, they established the well-designed CeONP with significant SOD- and CAT-mimicking capabilities and ROS scavenging power and further demonstrated its anti-inflammatory efficiency in vitro and in vivo by investigating the inflammation-associated cytokines and corresponding macrophage recruitment. Such results conclusively proved the ability of CeONP in antioxidant and anti-inflammatory fields. Additionally, as the important factors for potential clinical application, the toxicity and renal clearance of CeONP were also studied, and results indicated that no toxicity or any adverse effect was found in either control or CeONP group.88 Excessive ROS may unbalance oxidation, which is considered to promote abnormal vascularity. Zhang et al. established a Fe-SANzyme with significant CAT-mimic and SOD-mimic properties. Compared with natural catalysts and other nanozymes, Fe-SANzyme exerted a high affinity with H2O2, which further revealed its remarkable antioxidant activity. Additionally, in the mice model, the vascular malfunction was successfully alleviated by Fe-SANzyme treatment without affecting the normalization of vascularity. Based on the H & E staining result, Fe-SANzyme was suggested as a secure and efficient antioxidant medication of ocular retinopathy.89
As the systemic inflammatory response syndrome, sepsis is one of the oxidation-related inflammatory diseases brought on by microbial infections. Yang et al. designed Cu-SAzyme, which demonstrated excellent SOD-mimic properties for O2˙− elimination as well as desirable stability over a broad range of pH and temperature. Additionally, in the infected mice model, Cu-SAzyme effectively relieved multiple organ dysfunctions by diminishing extensive inflammatory response, exhibiting prolonged life expectancy of mice.90
4.2 Nanozyme in cardiovascular diseases
Cardiovascular disease (CVD) is considered to be the leading cause of death and disability globally.91 In recent years, in spite of the advances in the management and administration of CVD, some disorders still remain inadequately regulated.92 Current medication therapies for CVD still suffer from considerable drawbacks, including low drug potency, side effects, and tolerable doses of drugs.93 With the flourishing progress of nanomaterial medicines, CVDs based on ROS out-of-balance gamble, such as atherosclerosis,94–96 myocardial ischemia-related diseases96–99 and hypertension,100–102 have been confirmed to benefit from the elaborate nanoplatforms.
4.2.1 Nanozyme-based atherosclerosis therapy.
Antioxidant therapies are considered promising for the management of atherosclerosis due to the key role of reactive oxygen species (ROS) in the pathogenesis of atherosclerosis, but currently available antioxidants show rather limited clinical results.95 During atherogenesis, the primary process of inflammation is accompanied by a secondary event of oxidative stress, which is thought to be an oxidative response to inflammation.103 Although regulation of oxygen homeostasis and cell signaling requires low levels of reactive oxygen species (ROS),104 high levels of ROS can induce oxidative stress that is closely related to the pathogenesis of atherosclerosis. In addition, ROS can lead to NFκB activation, apoptosis, protein modification, and oxidative damage to other biomolecules.105–107 ROS also disrupts redox-dependent signaling in the vessel wall, thereby promoting the progression of atherosclerosis.108–110 Involving signal transduction pathways,111 regulatory genes associated with vascular function,110 atherogenic inflammatory component of sclerosis,112 and macrophage clearance of apoptotic cells.113
On the basis of oxygen homeostasis wobble, to enhance the inhibitory effect of antioxidant therapy on atherosclerosis, Wang et al. formulated broad-spectrum ROS-scavenging nanoparticles with anti-oxidative stress activity and high targeting potential.98 Tempol serves as the SOD mimetic agent and a hydrogen-peroxide-eliminating compound of phenylboronic acid pinacol ester together covalently attached to the cyclic polysaccharide β-cyclodextrin (referred to as TPCD). Tempol is responsible for the rapidity and catalytic nature of the reaction by shuttling between the nitroxide radical, the reduced hydroxylamine, and the oxidized ammonium oxide cation forms in a 1 and 2 electron transfer reaction, a conformational change known as “boat and chair”. Tempol interacts with the superoxide anion to form hydrogen peroxide, which accounts for its “superoxide dismutase (SOD) mimicry”.114 TPCD NPs significantly attenuated ROS-induced inflammation and apoptosis of macrophages. Meanwhile, TPCD NPs effectively inhibited the formation of foam cells in macrophages and VSMCs by reducing the internalization of oxidized low-density lipoproteins. Comprehensively, TPCD NPs achieved atherosclerosis relief by reducing systemic and local oxidative stress and inflammation as well as attenuating inflammatory cell infiltration in plaques. The conclusion drawn from the blood biochemistry analysis and pathology result was that the dose of TPCD NP administered intravenously was safe.
In search of therapeutics for atherosclerosis with tremendous potential for clinical translation, Zhang et al. employed a bionic Prussian blue (PB) analogue, the porous manganese substituted Prussian blue (PMPB) nanocube (NC),94 to diminish atherosclerotic plaque and treat atherosclerosis. The mimetic PMPB NCs have been proved to scavenge excess ROS (including free radicals and H2O2), eliminate oxidative stress, and reduce inflammation as enzymatic catalysts as well as drug-vehicles. Loaded with simvastatin (Sim), PMPB NCs platform also remarkably inhibited oxidative LDL internalization and foam cell formation to counteract atherosclerosis. Additionally, no obvious damage was found in the histologic results of major organs, suggesting the clinical translation potential of PMPB NCs.
Simvastatin@PMPB NC outperformed either simvastatin alone or PMPB NC alone in the treatment of AS. The most notable reduction in oxidative stress was seen as the PMPB NC reduced the release of pro-inflammatory cytokines. Additionally, there was a significant reduction in the internalization of oxidized LDL and the development of foam cells. Simvastatin@PMPB NC was therefore anticipated to have an anti-atherosclerotic effect due to the thinner fibrous cap in the AS plaques.159
4.2.2 Nanozyme-related myocardial ischemia therapy.
Although mechanisms of ischemia-reperfusion (IR) injury have not been fully revealed, excessive ROS, especially O2˙−, is implicated as the essential factor in its progression98. By degrading O2˙− into O2 and H2O2, SOD exerts great potential in disease therapy; however, natural SOD often suffers from easy deactivation and poor targeting, paving the way for developing nanozymes with SOD-mimic property. Zhang et al. created a Mn-based nanozyme integrated with human-protein derived nanocage to target mitochondria and exhibit IR injury therapeutic capability. The recombination (Mito-Fenozyme) showed SOD-mimic and CAT-mimic properties without producing toxic OH˙− due to its low POD-like activity. In addition, this synthetic nanozyme offered effective ROS free radical breakdown into benign compounds, demonstrating its benefits in preventing mitochondrial oxidative damage. Due to the increased expression of the FTn receptor in ischemic tissues, the tailored nanozyme showed preferential accumulation and targeting to ischemic tissues and could penetrate into cardiac tissues when locally administered in conjunction with adhesive hydrogel cardiac patches, thus leading to enhanced recovery of cardiac function99 (Fig. 6).
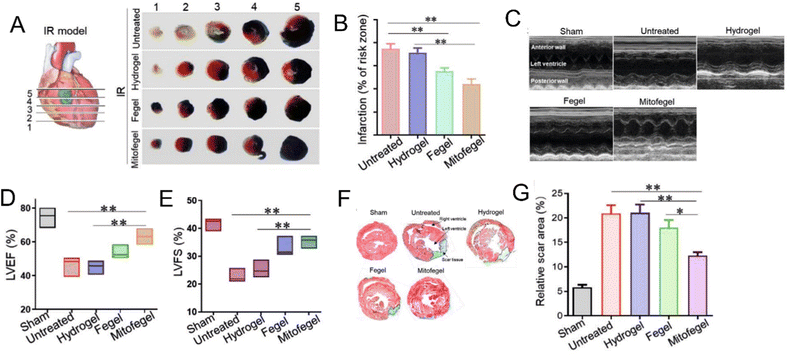 |
| Fig. 6 The impact of the adhesive Mitofegel on mouse IR models and heart function restoration. (A) The infarct size of heart sections after IR surgery by Evans Blue and TTC double staining. (B) The quantification results of infarct zone. (C) Echocardiography of left ventricular wall motion, LVEF and LVFS indexes, Masson staining of myocardium, healthy myocardial area, ATP level, and ATP5A expression in sham and IR cardiac tissues after various therapies. (D and E) The quantification analysis of LVEFs and LVFS in various groups according to echocardiography results. (F) The midpapillary regions of heart tissues by Masson staining. (G) quantification results according to (F). IR: ischemia reperfusion. LVEF: left ventricular ejection fractions. LVFS: left ventricular fractional shortening. Reprinted with permission.99 Copyright 2021 Wiley-VCH GmbH. | |
4.2.3 Nanozyme-based hypertension alleviation.
Cardiovascular diseases, such as chronic heart failure and hypertension, have proved to be closely interacted with Angiotensin II (AngII) originated O2˙−,101 thus requiring the demanding attention of effective antioxidants. From this standpoint, Savalia et al.102 constructed a nanozyme denoted as PLL50-PEG CuZnSOD, which exhilaratingly exerted SOD-like activity. In the C57BL/6 mice model particularly, the novel nanozyme indicated a remarkable alleviation of hypertension induced by AngII through reducing excessive O2˙−, demonstrating its potential role in cardiovascular system related therapy. Evidence also showed that the CuZnSOD nanozyme could deliver active CuZnSOD protein to central neurons in culture without causing severe damage.
Heart failure and possibly death can result from the hazardous condition known as pulmonary arterial hypertension (PAH). It is acknowledged that the substantial rise of NO may account for increasing oxidative stress in sick areas, leading to even worse circumstances. Furthermore, right ventricular (RV) hypertrophy, a representative sign, would start as a result of long-term hypertension. Nanozymes have been used to treat these issues and prevent the aberrant development of the right ventricle. Ceria nanoparticles demonstrated a brilliant ability to decrease unwelcome tissue growth in a monocrotaline-induced (MCT) rat model by lowering oxidative stress and cardiomyocyte death. The related results of the nanozyme treatment group had no significant changes compared to the beginning for the measurements of pulmonary arterial pressure (PA Pr), mean pulmonary arterial diameter (MPA diameter), mean pulmonary arterial area (MPA area), and right ventricular outflow tract diameter (RVOT), whereas the control group showed a range of higher parameters. Additionally, nanozymes clearly reduced RV anterior free wall thickness and interventricular septum diameter.160
4.3 Nanozyme in nervous system
The overwhelming ROS and/or reactive nitrogen species (RNS) production, in particular, is believed as the leading cause of brain damage that may induce ischemic stroke.115 Recently, the contribution of nanozyme in shielding nerve cells from oxidative damage has been revealed, highlighting its role in alleviating neurological disorders. Zhang et al. engineered smart hollow PB nanozymes (HPBZs) to achieve multi-enzyme mimicking functions by inhibiting ONOO–, H2O2, O2˙−, and ˙OH up to 72%, 88%, 79%, and 62%, respectively. With further mouse models of middle cerebral artery occlusion (MCAO) surgery, they found that the HPBZs treatment group significantly improved neurological scores, demonstrating its inspirational effect in alleviating ischemic stroke. In addition, HPBZs were demonstrated to successfully guard neurons against hypoxic/ischemic injury both in vitro and in vivo, mainly through antioxidative, anti-inflammatory, and anti-apoptotic activities with little perceptible side effects (Fig. 7).116 Similarly, Liu et al.115 established the Co–Fe3O4 nanozyme with prominent catalytic properties. Fe3O4, as the extensively applied nanozyme, has demonstrated its extraordinary role in catalase-mimic, peroxidase-mimic and decreasing reactive oxygen and nitrogen species (RONS) capabilities.116,118 Combined with Co, the Co–Fe3O4 nanozyme could exert superior affinity to scavenge RONS induced ischemia injury with subsequent neuroprotection both in vitro and in vivo. Furthermore, Co–Fe3O4 nanozyme was proved to act effectively toward neuroinflammatory reactions, while in short- and long-term investigations of healthy mice, it would not induce inflammatory response or damage in peripheral organs.117
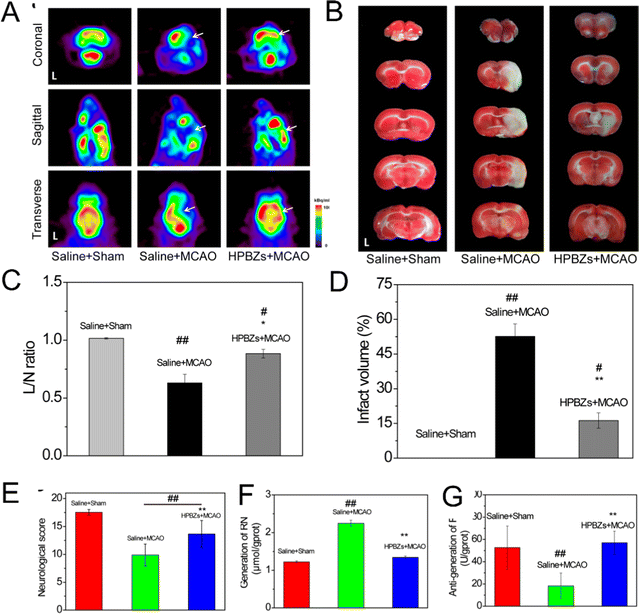 |
| Fig. 7 A rat model of cerebral ischemia/reperfusion injury, pretreatment with HPBZs reduced cerebral metabolic impairment, infarct volume, mitigated neurological impairments, and suppressed the production of RONS. (A) Cerebral 18F-FDG PET images and (C) semiquantitative analysis of cerebral glucose metabolism in different groups. (B) TTC-stained coronal brain images and (D) quantitative results of infarct region in different groups. (E) Pretreatment with HPBZs dramatically decreased brain impairment following ischemia/reperfusion injury, according to neurological testing. (F and G) ROS/RNS generation in brain tissues of Saline + Sham, Saline + MCAO, and HPBZs + MCAO groups. MCAO: middle cerebral artery occlusion. RNS: reactive nitrogen species. ROS: reactive oxygen species. Reprinted with permission.116 Copyright 2019 American Chemical Society. | |
After cardiovascular illnesses, strokes are the second biggest cause of death worldwide. The most common type of stroke is an ischemic stroke, which is primarily caused by an abrupt stoppage of the blood flow to the brain together with an explosive rise in ROS. Neural apoptosis also results in ischemia reperfusion injury to the brain.161 A multi-enzyme cascade antioxidant system was just recently created to reduce intracellular ROS and prevent neuronal apoptosis during cerebral ischemic reperfusion injury (CIRI) by Liu and colleagues. They were able to successfully create the Fe2NC@Se nanozyme, which had good cytoprotective properties and three different types of antioxidant activities. Fe2NC@Se nanozyme prevented MCAO rats from dying by inhibiting the ASK1/JNK signaling pathway in the MCAO paradigm of SD rats. The antioxidant properties of Fe2NC@Se nanozyme prevented neuronal apoptosis brought on by oxidative stress, significantly reduced infarct volume, and restored neurological impairments.162
It has been acknowledged that oxidative disequilibrium accounts for the progression of Alzheimer's disease (AD)-related β-amyloid (Aβ) plaques and tau protein-derived neurofibrillary tangles (NFTs).119,120 Although ROS elimination-targeted drugs have been developed and applied, it is still reluctant to achieve the desirable treating efficiency in AD due to the blood–brain barrier (BBB).121,122 Nanozymes are thus adopted for overcoming the drawback with the delicate-designed structure and multi enzyme-like activities. For instance, octahedral palladium nanoparticles (Pd NPs) possessing peroxidase- and superoxide-mimic properties were chosen for synthesis with PEG and traditional Chinese medicine (i.e., Bor) to construct Pd@PEG@Bor, which achieved potent BBB crossing, significant ROS decreasing, favorable Aβ suppression, and remarkable cognitive damage mitigation.122 Similarly, CuxO@EM-K, another novel nanozyme, was synthesized to enhance Aβ targeting capability based on KLVFF peptide and erythrocyte membrane, along with CuxO to realize various antioxidant enzyme-like functions. The AD mice model further verified that CuxO@EM-K was capable of Aβ degradation as well as oxidative damage mitigation, which could subsequently alleviate brain impairment induced by Aβ in AD with negligible toxicity.123 Not only for AD, the unstable homeostasis of oxidation is also the main cause of Parkinson's disease (PD). The interaction between ROS and nucleotide-binding domain and leucine-rich repeat family pyrin domain containing 3 (NLRP3) has been enlightened in PD treatment.124–126 By exploiting PB as nanozyme to realize catalytic properties in scavenging ROS, Ma et al. developed PBzyme to suppress NLRP3 inflammasome activity and pyroptosis both in vitro and in vivo, providing a promising strategy for PD therapy. Additionally, even though studies indicated that PBzyme did not cause any adverse effects, its pharmacokinetics and long-term toxicity still need to be further defined.127 The varied and complex causes of PD make therapy challenging, and the existing therapeutic medications have problematic side effects that lead to problems. There is proof that ROS induces neuronal malfunction because of oxidation brought on by mitochondrial dysfunction, offering a creative cure for neurodegenerative conditions like PD.163 However, physiological circumstances can affect natural antioxidants. Researchers have therefore been paying closer attention to the creation of nanozymes for PD therapy, especially those that have a stronger and wider range of antioxidant characteristics. A popular antioxidant nanozyme is CeO2. Due to its poor BBB permeability and ROS-catalytic characteristics, CeO2 has limits in the treatment of PD. Li et al. created Mn3O4 nanozymes with multi-enzymatic capabilities that were noticeably better than the other available nanozymes, including Fe3O4, CeO2, and V2O5. The created Mn3O4 nanoparticles showed considerable potential in the prevention of ROS-mediated PD in a disease model by playing a favorable protective activity in MPP + -induced nerve cell damage through its redox modulatory impact.164
The complex, multifaceted disease, i.e., Huntington's disease that also includes oxidative stress and epigenetic variables, is a representative ailment. To repair neuronal processes and prevent oxidative damages to the brain, Chen and colleagues created selenium nanoparticles. They performed behavioral dysfunction by inducing Huntington's illness in Caenorhabditis elegans, an animal model.165
Traumatic brain injury (TBI) often induces excessive RONS leading to severe damage to the nervous system.128 To reverse the oxidative imbalance, single-atom catalyst featured with high scavenging and catalytic efficiency among various nanozymes has aroused extensive attention.129,130 Yan et al., for example, prepared a single-atom Pt/CeO2-combined nanozyme that not only exhibited improved multi-enzyme mimicry but also higher RONS scavenging efficiency, providing a complete defense of neurons in TBI. Meanwhile, the approach offered a different noninvasive therapy option for TBI without any negative effects.131 CeO2 nanozyme could mimic enzymatic activities similar to SOD and CAT, but its GPx-mimetic properties have not been clearly stated,132 while the proportion of Ce3+/Ce4+ is believed to significantly influence the scavenge efficiency of RONS.133 To remedy this deficiency, Zhang et al. constructed a Cr/CeO2 nanozyme to deal with TBI. Encouragingly, Cr in this novel structure was harnessed to convert Ce3+ to Ce4+, achieving not only satisfactory catalytic properties of SOD, CAT, and GPx, but also an impressive clearance of RONS. Furthermore, this nanozyme-oriented therapeutic approach was demonstrated to be effective in terms of oxidative stress degradation and neuroprotective capacity by the further study of a mouse model of brain injury. Finally, via the observation of mice training behavior, its effective neurocognitive recovery was convinced. With minimal side effects, noninvasiveness and good biocompatibility, the research provided a TBI treatment strategy through the nanozyme patch (Fig. 8).132
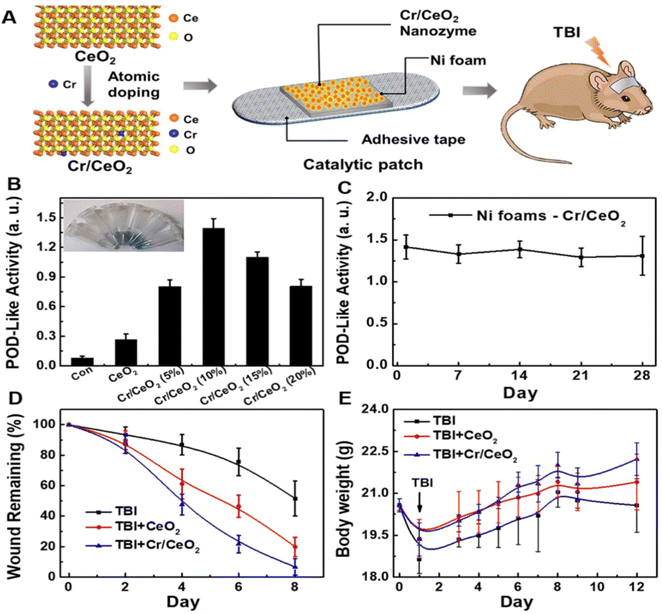 |
| Fig. 8 (A) Mechanism diagram of Cr/CeO2 nanozyme. (B) POD-mimic property of Cr/CeO2 nanozyme in various scale. (C) The stability characteristic of Cr/CeO2. (D) Mouse wound length following TBI with or without nanozyme patch therapy. (E) Body weight results of mice after TBI with or without nanozyme patch therapy. TBI: traumatic brain injury. Reprinted with permission.132 Copyright 2021 Ivyspring International Publisher. | |
Few medications are available because large nanoparticles are easily captured by the reticuloendothelial system. Thus, non-invasive medicines and ultrasmall, tailored nanozymes are being developed. He et al., for instance, proposed ultrasmall nanoparticles that gathered in ROS-rich environments. According to research, the nanozyme has nearly nine-fold greater absorption than other nanozymes and prefers to assemble in the presence of ROS in the mitochondria, offering it considerable promise for reducing ROS damage in TBI.166 Similarly, Mu and coworkers created a carbogenic small-sized nanozyme to effectively remove RONS in TBI. Due to its high enzymatic activity and selective RONS elimination, the nanozyme was utilized successfully in both in vitro research involving TBI mice.167
5. Conclusion and outlook
Compared with natural enzymes, as enzyme-like nanomaterials, nanozymes are characterized by flexible design, high stability, simple preparation, and tunable catalysis. Based on these unique features, considerable exploration has been carried out on nanomaterials for clinical practice. In this review, we discussed the versatile applications of nanozymes related to tumor therapy (including TME-oriented tumor therapy, synergistic therapy with PDT/PTT/SDT, and anti-tumor immunotherapy) and non-oncological related anti-inflammatory therapy, cardiovascular disease therapy, and nervous system disease therapy. By demonstrating the interactions between nanozyme and other catalyst/drug/nanomaterial, we illuminated various enzymatic features, biosafety, and recent advances in therapeutic modalities of different nanozymes, hoping to enlighten more advances and breakthroughs in the field of nanozyme-oriented disease treatment.
With the rapid pace of advances in nanozyme-oriented cross-over fields, novel strategies for disease therapy will increasingly be enlightened. Undeniably, though nanozymes have compensated for a large number of deficiencies of traditional pro-oxidants and antioxidants, there still remain some challenges to be addressed.
(1) In light of the great potential of nanozymes in therapeutic research that has been revealed, the biosafety and biocompatibility of nanozymes still own prior attention in further practice. In essence, nanozymes are mostly inorganic nanoparticles that enter and interact with lysosomes, mitochondria, or nuclei, leading to irreversible intracellular damage that may be unavoidable. Therefore, it is essential to minimize the toxicity of the nanozymes themselves by optimization and modification. In addition, nanozymes may also act as a foreign substance to induce an immune response in the system. Unfortunately, most of the recent in vivo studies have focused on mice, leaving the deep interaction between nanozymes and the human system challenging. Overall, in the long term, it is necessary to emphasize on metabolic pattern-related studies to facilitate further clinical translation.
(2) Compared with natural enzymes and organocatalysts, the catalytic activity or efficiency of nanozymes still remains at a relatively low level at present.134 From this perspective, measures to tune sizes and modify surfaces should be taken to achieve greater efficiency. For instance, the molecularly imprinted polymers can be employed to encapsulate the nanozyme to significantly improve its catalytic selectivity;135 or nanozymes and natural enzymes could be combined simultaneously to achieve specific recognition and catalysis of target molecules.136 A recent study reported by Fan et al. showed that although Fe3O4 nanozyme exhibited a similar catalytic efficiency to HRP, its substrate-binding affinity was substantially lower. Therein, they modified the surface of Fe3O4 with histidine residues so as to boost the affinity with H2O2 binding sites.137 Furthermore, challenges still remain for the regulation between multiple enzymatic activities. The modulation of ROS level is a critical mechanism for the therapeutic basis of nanozymes;138 however, since a nanozyme often possesses more than one enzyme-mimicking activity, how to balance the antioxidant or pro-oxidant properties, or how to precisely control its optimal catalytic activity are issues that still require further discussion.
(3) As a novel therapeutic strategy, many research studies associated with nanozymes for treatment have been discovered and have become the focus of attention. However, a long way lies ahead for the clinical translation of these studies. The reasons are provided as follows: firstly, the mechanisms of many nanozymes in treatment are still obscure, which requires further efforts in this field. Secondly, given those specific criteria for nanozyme-oriented therapies are not yet well established or defined, this may not be sufficient to guide their eventual clinical application. Therefore, an urgent demand exists for more in-depth studies to elucidate their standardization and performance in order to enlighten further clinical practice in the future.
(4) To elevate the treatment efficiency, combined or synergistic therapy is usually encouraged.139–158 Inspired by this, nanozymes can be integrated with immunotherapy, chemotherapy, and other ROS-highlighted therapy to magnify the treatment efficiency. This direction will also arouse more interests, propelling clinical translation especially when imparting these functions into one platform and realizing the all-in-one strategy.
In conclusion, accompanied by the mature progress in the nanozyme development in high safety, excellent stability, ideal enzymatic activity, and high specificity, the further explorations of the catalytic mechanism relevant to nanozymes and the expansion of corresponding indications will also be the highlights in therapies, which will bring more inspiring research to meet the future scope of nanozymes.
Conflicts of interest
There are no conflicts to declare.
Acknowledgements
This work was supported by National Natural Science Foundation of China (Grant Number: 82022033), Shanghai Rising-Star Program (Grant No. 19QA1406800), Shanghai Talent Development Fund (Grant No. 2019040), the Fundamental Research Funds for the Central Universities (22120210561) and the program for Shanghai Young Top-Notch Talent.
References
- G. V. Mireia, J. Gao, K. Martin and T. Donald, How enzymes work: analysis by modern rate theory and computer simulations, Science, 2004, 303, 186–195 CrossRef PubMed.
- M. Wei, J. Lee, F. Xia, P. Lin, X. Hu, F. Li and D. Ling, Chemical design of nanozymes for biomedical applications, Acta Biomater., 2021, 126, 15–30 CrossRef CAS PubMed.
- Y. Lin, J. Ren and X. Qu, Catalytically active nanomaterials: a promising candidate for artificial enzymes, Acc. Chem. Res., 2014, 47, 1097–1105 CrossRef CAS PubMed.
- H. Wei, L. Gao, K. Fan, J. Liu, J. He, X. Qu, S. Dong, E. Wang and X. Yan, Nanozymes: A clear definition with fuzzy edges, Nano Today, 2021, 40, 101269 CrossRef CAS.
- M. Liang and X. Yan, Nanozymes: from new concepts, mechanisms, and standards to applications, Acc. Chem. Res., 2019, 52, 2190–2200 CrossRef CAS PubMed.
- L. Gao, J. Zhuang, L. Nie, J. Zhang, Y. Zhang, N. Gu, T. Wang, J. Feng, D. Yang, S. Perrett and X. Yan, Intrinsic peroxidase-like activity of ferromagnetic nanoparticles, Nat. Nanotechnol., 2007, 2, 577–583 CrossRef CAS.
- R. Zhang, X. Yan and K. Fan, Nanozymes inspired by natural enzymes, Acc. Mater. Res., 2021, 2, 534–547 CrossRef CAS.
- Z. Zhang, X. Zhang, B. Liu and J. Liu, Molecular imprinting on inorganic nanozymes for hundred-fold enzyme specificity, J. Am. Chem. Soc., 2017, 139, 5412–5419 CrossRef CAS PubMed.
- G. Tang, J. He, J. Liu, X. Yan and K. Fan, Nanozyme for tumor therapy: surface modification matters, Exploration, 2021, 1, 75–89 CrossRef.
- Y. Huang, J. Ren and X. Qu, Nanozymes: classification, matalytic mechanisms, activity regulation, and applications, Chem. Rev., 2019, 119, 4357–4412 CrossRef CAS PubMed.
- X. Wang, X. J. Gao, L. Qin, C. Wang, L. Song, Y. N. Zhou, G. Zhu, W. Cao, S. Lin, L. Zhou, K. Wang, H. Zhang, J. Zhong, P. Wang, X. Gao and H. Wei, e(g) occupancy as an effective descriptor for the catalytic activity of perovskite oxide-based peroxidase mimics, Nat. Commun., 2019, 10, 704 CrossRef CAS PubMed.
- J. Wu, X. Wang, Q. Wang Q, Z. Lou, Y. Zhu, L. Qin and H. Wei, Nanomaterials with enzyme-like characteristics (nanozymes): next-generation artificial enzymes (II), Chem. Soc. Rev., 2019, 48, 1004–1076 RSC.
- Y. Shang, F. Liu, Y. Wang, N. Li and B. Ding, Enzyme Mimic Nanomaterials and Their Biomedical Applications, ChemBioChem, 2020, 17, 2408–2418 CrossRef.
- N. J. Durr, T. Larson, D. K. Smith, B. A. Korgel, K. Sokolov and A. Ben-Yakar, Two-photon luminescence imaging of cancer cells using molecularly targeted gold nanorods, Nano Lett., 2007, 7, 941e5 CrossRef.
- W. He, S. Hou, X. Mao, X. Wu, Y. Ji, J. Liu, X. Hu, K. Zhang, C. Wang, Y. Yang and Q. Wang, Peptide-tailored assembling of Au nanorods, Chem. Commun., 2011, 47, 5482–5484 RSC.
- J. Li, L. Zou, D. Hartono, C. Ong, B. H. Bay and L. Yung, Gold Nanoparticles Induce Oxidative Damage in Lung Fibroblasts In Vitro, Adv. Mater., 2008, 1, 138–142 CrossRef.
- Y. Pan, S. Neuss, A. Leifert, M. Fischler, F. Wen, U. Simon, G. Schmid, W. Brandau and W. Jahnen-Dechent, Size-dependent cytotoxicity of gold nanoparticles, Small, 2007, 11, 1941–1949 CrossRef.
- X. Niu, N. Cheng, X. Ruan, D. Du and Y. Lin, Nanozyme-Based Immunosensors and Immunoassays: Recent Developments and Future Trends, J. Electrochem. Soc., 2020, 167, 037508 CrossRef CAS.
- W. He, Y. Zhou, G. Wamer, D. Boudreau and J. Yin, Mechanisms of the pH dependent generation of hydroxyl radicals and oxygen induced by Ag nanoparticles, Biomaterials, 2012, 30, 7547–7555 CrossRef.
- L. Long, R. Cai, J. Liu and X. Wu, A Novel Nanoprobe Based on Core–Shell Au@Pt@Mesoporous SiO2 Nanozyme With Enhanced Activity and Stability for Mumps Virus Diagnosis, Front Chem., 2020, 8, 463 CrossRef CAS PubMed.
- C. Ge, G. Fang, X. Shen, Y. Chong, G. Wamer, X. Gao, Z. Chai, C. Chen and J. Yin, Facet Energy versus Enzyme-like Activities: The Unexpected Protection of Palladium Nanocrystals against Oxidative Damage, ACS Nano, 2016, 11, 10436–10445 CrossRef PubMed.
- L. Ran, H. Huang, Y. Li, L. Song and Y. Xiong, 10. Palladium-Based Nanomaterials: A Platform to Produce Reactive Oxygen Species for Catalyzing Oxidation Reactions, Adv. Mater., 2015, 44, 7025–7042 Search PubMed.
- E. Antolini, Iridium as Catalyst and Cocatalyst for Oxygen Evolution/Reduction in Acidic Polymer Electrolyte Membrane Electrolyzers and Fuel Cells, ACS Catal., 2014, 4, 1426–1440 CrossRef CAS.
- H. Kobayashi, M. Yamauchi and H. Kitagawa, Finding HydrogenStorage Capability in Iridium Induced by the Nanosize Effect, J. Am. Chem. Soc., 2012, 134, 6893–6895 CrossRef CAS.
- W. Hui and E. Wang, Fe3O4 magnetic nanoparticles as peroxidase mimetics and their applications in H2O2 and glucose detection, Anal. Chem., 2008, 80, 2250–2254 CrossRef.
- Z. Zhang, X. Wang and X. Yang, A sensitive choline biosensor using Fe3O4 magnetic nanoparticles as peroxidase mimics, Analyst, 2011, 23, 4960–4965 RSC.
- N. Puvvada, K. Panigrahi, D. Mandal and A. Pathak, Shape dependent peroxidase mimetic activity towards oxidation of pyrogallol by H2O2, RSC Adv., 2012, 8, 3270–3273 RSC.
- K. Fan, H. Wang, J. Xi, Q. Liu, X. Meng, D. Duan, L. Gao and X. Yan, Optimization of Fe3O4 nanozyme activity via single amino acid modification mimicking an enzyme active site, Chem Commun., 2016, 53, 424–427 RSC.
- Y. Yang, S. Luo, H. Li, S. Dong and X. Yang, Alendronate as a robust anchor for ceria nanoparticle surface coating: facile binding and improved biological properties, RSC Adv., 2014, 104, 59965–59969 RSC.
- V. Baldim, F. Bedioui, N. Mignet, I. Margaill and J. F. Berret, Nanoscale, 2018, 10, 6971–6980 RSC.
- M. Soh, D. W. Kang, H. G. Jeong, D. Kim, D. Y. Kim, W. Yang, C. Song, S. Baik, I. Y. Choi, S. K. Ki, H. J. Kwon, T. Kim, C. K. Kim, S. H. Lee and T. Hyeon, Angew. Chem., Int. Ed., 2017, 56, 11399–11403 CrossRef CAS PubMed.
- M. Hijaz, S. Das, I. Mert, A. Gupta, Z. Al-Wahab, C. Tebbe, S. Dar, J. Chhina, S. Giri, A. Munkarah, S. Seal and R. Rattan, Folic acid tagged nanoceria as a novel therapeutic agent in ovarian cancer, BMC Cancer, 2016, 16, 220 CrossRef.
- S. Saraf, C. Neal and S. Das, Understanding the Adsorption Interface of Polyelectrolyte Coating on Redox Active Nanoparticles Using Soft Particle Electrokinetics and Its Biological Activity, ACS Appl. Mater. Interfaces, 2014, 8, 5472–5482 CrossRef.
- Q. Sun, F. He, C. Sun, X. Wang, C. Li, J. Xu, D. Yang, H. Bi, S. Gai and P. Yang, Honeycomb-Satellite Structured pH/HO-Responsive Degradable Nanoplatform for Efficient Photodynamic Therapy and Multimodal Imaging, ACS Appl. Mater. Interfaces, 2018, 40, 33901–33912 CrossRef PubMed.
- Y. Chen, D. Ye, M. Wu, H. Chen, L. Zhang, J. Shi and L. Wang, Break-up of two-dimensional MnO2 nanosheets promotes ultrasensitive pH-triggered theranostics of cancer, Adv. Mater., 2014, 41, 7019–7026 CrossRef.
- Y. Zhu, D. Jin, M. Liu, Y. Dai, L. Li, X. Zheng, L. Wang, A. Shen, J. Yu, S. Wu, Y. Wu, K. Zhong, J. Cheng and Y. Liu, Oxygen Self-Supply Engineering-Ferritin for the Relief of Hypoxia in Tumors and the Enhancement of Photodynamic Therapy Efficacy, Small, 2022, 15, e2200116 CrossRef.
- A. P. Periasamy, P. Roy, W. P. Wu, Y. H. Huang and H. T. Chang, Glucose oxidase and horseradish peroxidase like activities of cuprous oxide/polypyrrole composites, Electrochimica Acta., 2016, 215, 253–260 CrossRef CAS.
- W. Zhang, S. Hu, J. Yin, W. He, W. Lu, M. Ma, N. Gu and Y. Zhang, Prussian blue nanoparticles as multienzyme mimetics and reactive oxygen species scavengers, J. Am. Chem. Soc., 2016, 138, 5860–5865 CrossRef CAS PubMed.
- H. Dong, Y. Fan, W. Zhang, N. Gu and Y. Zhang, Catalytic Mechanisms of Nanozymes and Their Applications in Biomedicine, Bioconjug Chem, 2019, 5, 1273–1296 CrossRef PubMed.
- L. Jiao, W. Yang, G. Wan, R. Zhang, X. Zheng, H. Zhou, S. Yu and H. Jiang, Single-atom electrocatalysts from multivariate metal-organic frameworks for highly selective reduction of co(2)at low pressures, Angew. Chem., 2020, 46, 20589–20595 CrossRef PubMed.
- Y. Zheng, J. Lin, L. Xie, H. Tang, K. Wang and J. Liu, One-step preparation of nitrogen-doped graphene quantum dots with anodic electrochemiluminescence for sensitive detection of hydrogen peroxide and glucose, Front. Chem., 2021, 9, 688358 CrossRef CAS PubMed.
- J. Gong, Z. Zhang, Z. Zeng, W. Wang, L. Kong, J. Liu and P. Chen, Graphene quantum dots assisted exfoliation of atomically-thin 2D materials and as-formed 0D/2D van der Waals heterojunction for HER, Carbon, 2021, 184, 554–561 CrossRef CAS.
- G. Qiu, Y. Han, X. Zhu, J. Gong, T. Luo, C. Zhao, J. Liu and X. Li, Sensitive detection of sulfide ion based on fluorescent ionic liquid-graphene quantum dots nanocomposite, Front. Chem., 2021, 9, 658045 CrossRef CAS PubMed.
- L. Lu, L. Zhou, J. Chen, F. Yan, J. Liu, X. Dong, F. Xi and P. Chen, Nanochannel-confined graphene quantum dots for ultrasensitive electrochemical analysis of complex samples, ACS Nano, 2018, 12, 12673–12681 CrossRef CAS PubMed.
- F. Bray, J. Ferlay, I. Soerjomataram, R. L. Siegel, L. A. Torre and A. Jemal, Global cancer statistics 2018: GLOBOCAN estimates of incidence and mortality worldwide for 36 cancers in 185 countries, CA Cancer J. Clin., 2018, 68, 394–424 CrossRef.
- Y. Wang, H. Li, L. Guo, Q. Jiang and F. Liu, A cobalt-doped iron oxide nanozyme as a highly active peroxidase for renal tumor catalytic therapy, RSC Adv., 2019, 9, 18815–18822 RSC.
- S. Ali, S. Sikdar, S. Basak, B. Rajbanshi, M. Mondal, D. Roy, A. Dutta, A. Kumar, V. K. Dakua, R. Chakrabarty, A. Roy, A. Barman, A. Datta, P. K. Roy, B. Chakraborty and M. N. Roy, β-cyclodextrin-stabilized biosynthesis nanozyme for dual enzyme mimicking and Fenton reaction with a high potential anticancer agent, ACS Omega, 2022, 7, 4457–4470 CrossRef CAS PubMed.
- S. Yao, X. Zhao, X. Wang, T. Huang, Y. Ding, J. Zhang, Z. Zhang, Z. L. Wang and L. Li, Bioinspired electron polarization of nanozymes with a human self-generated electric field for cancer catalytic therapy, Adv. Mater., 2022, 34, 2109568 CrossRef CAS PubMed.
- R. Yang, S. Fu, R. Li, L. Zhang, Z. Xu, Y. Cao, H. Cui, Y. Kang and P. Xue, Facile engineering of silk fibroin capped AuPt bimetallic nanozyme responsive to tumor microenvironmental factors for enhanced nanocatalytic therapy, Theranostics, 2021, 11, 107–116 CrossRef CAS PubMed.
- Y. Hu, K. Wang and C. Ye, “Four-in-one” nanozyme and natural enzyme symbiotic system of Cu2-x Se-GOx for cervical cancer therapy, Chem. – Eur. J., 2022, 28, e202102885 CAS.
- C. Wei, Y. Liu, X. Zhu, X. Chen, Y. Zhou, G. Yuan, Y. Gong and J. Liu, Iridium/ruthenium nanozyme reactors with cascade catalytic ability for synergistic oxidation therapy and starvation therapy in the treatment of breast cancer, Biomaterials, 2020, 238, 119848 CrossRef CAS.
- Y. Yao, Z. Wang, Q. Cao, H. Li, S. Ge, J. Liu, P. Sun, Z. Liu, Y. Wu, W. Wang and J. Liu, Degradable tumor-responsive iron-doped phosphate-based glass nanozyme for H2O2 self-supplying cancer therapy, ACS Appl. Mater. Interfaces, 2022, 14, 17153–17163 CrossRef CAS PubMed.
- M. Hu, K. Korschelt, P. Daniel, K. Landfester, W. Tremel and M. B. Bannwarth, Fibrous nanozyme dressings with catalase-like activity for H2O2 reduction to promote wound healing, ACS Appl. Mater. Interfaces, 2017, 9, 38024–38031 CrossRef CAS PubMed.
- Y. Duo, M. Suo, D. Zhu, Z. Li, Z. Zheng and B. Z. Tang, AIEgen-based bionic nanozymes for the interventional photodynamic therapy-based treatment of orthotopic Colon cancer, ACS Appl. Mater. Interfaces, 2022, 14, 26394–26403 CrossRef CAS PubMed.
- Y. Hao, Y. Chen, X. He, Y. Yu, R. Han, Y. Li, C. Yang, D. Hu and Z. Qian, Polymeric nanoparticles with ROS-responsive prodrug and platinum nanozyme for enhanced chemophotodynamic therapy of Colon cancer, Adv. Sci., 2020, 7, 2001853 CrossRef CAS.
- Y. Zhu, D. Jin, M. Liu, Y. Dai, L. Li, X. Zheng, L. Wang, A. Shen, J. Yu, S. Wu, Y. Wu, K. Zhong, J. Cheng and Y. Liu, Oxygen self-supply engineering-ferritin for the relief of hypoxia in tumors and the enhancement of photodynamic therapy efficacy, Small, 2022, 18, 2200116 CrossRef CAS PubMed.
- Y. Zhu, Z. Wang, R. Zhao, Y. Zhou, L. Feng, S. Gai and P. Yang, Pt decorated Ti3C2Tx MXene with NIR-II light amplified nanozyme catalytic activity for efficient phototheranostics, ACS Nano, 2022, 16, 3105–3118 CrossRef CAS PubMed.
- F. Liu, L. Lin, Y. Zhang, S. Sheng, Y. Wang, C. Xu, H. Tian and X. Chen, Two-dimensional nanosheets with high curcumin loading content for multimodal imaging-guided combined chemo-photothermal therapy, Biomaterials, 2019, 223, 119470 CrossRef CAS PubMed.
- H. Xiang, H. Lin, L. Yu and Y. Chen, Hypoxia-irrelevant photonic thermodynamic cancer nanomedicine, ACS Nano, 2019, 13, 2223–2235 CAS.
- S. Dong, Y. Dong, T. Jia, S. Liu, J. Liu, D. Yang, F. He, S. Gai, P. Yang and J. Lin, GSH-depleted nanozymes with hyperthermia-enhanced dual enzyme-mimic activities for tumor nanocatalytic therapy, Adv. Mater., 2020, 32, 2002439 CrossRef CAS PubMed.
- N. Feng, Q. Li, Q. Bai, S. Xu, J. Shi, B. Liu and J. Guo, Development of an Au-anchored Fe Single-atom nanozyme for biocatalysis and enhanced tumor photothermal therapy, J. Colloid Interface Sci., 2022, 618, 68–77 CrossRef CAS PubMed.
- P. Qi, J. Zhang, Z. Bao, Y. Liao, Z. Liu and J. Wang, A platelet-mimicking single-atom nanozyme for mitochondrial damage-mediated mild-temperature photothermal therapy, ACS Appl. Mater. Interfaces, 2022, 14, 19081–19090 CrossRef CAS PubMed.
- S. Liang, B. Liu, X. Xiao, M. Yuan, L. Yang, P. Ma, Z. Cheng and J. Lin, A robust narrow bandgap vanadium tetrasulfide sonosensitizer optimized by charge separation engineering for enhanced sonodynamic cancer therapy, Adv. Mater., 2021, 33, 2101467 CrossRef CAS PubMed.
- Z. Gong and Z. Dai, Design and challenges of sonodynamic therapy system for cancer theranostics: from equipment to sensitizers, Adv. Sci., 2021, 8, 2002178 CrossRef CAS PubMed.
- X. Qiao, L. Xue, H. Huang, X. Dai, Y. Chen and H. Ding, Engineering defected 2D Pd/H-TiO2 nanosonosensitizers for hypoxia alleviation and enhanced sono-chemodynamic cancer nanotherapy, J. Nanobiotechnol., 2022, 20, 186 CrossRef CAS PubMed.
- A. Yamasaki, K. Yanai and H. Onishi, Hypoxia and pancreatic ductal adenocarcinoma, Cancer Lett., 2020, 484, 9–15 CrossRef CAS PubMed.
- J. Chen, Y. Bao, Y. Song, C. Zhang, F. Qiu, Y. Sun, L. Xin, J. Cao, Y. Jiang, J. Luo, C. Zhang, G. Wang, Q. Li, Y. Liu, W. Tong and P. Huang, Hypoxia-alleviated nanoplatform to enhance chemosensitivity and sonodynamic effect in pancreatic cancer, Cancer Lett., 2021, 520, 100–108 CrossRef CAS PubMed.
- S. Liu, W. Zhang, Q. Chen, J. Hou, J. Wang, Y. Zhong, X. Wang, W. Jiang, H. Ran and D. Guo, Multifunctional nanozyme for multimodal imaging-guided enhanced sonodynamic therapy by regulating the tumor microenvironment, Nanoscale, 2021, 13, 14049–14066 RSC.
- X. Wang, X. Zhong, L. Bai, J. Xu, F. Gong, Z. Dong, Z. Yang, Z. Zeng, Z. Liu and L. Cheng, ultrafine titanium monoxide (TiO1 + x) nanorods for enhanced sonodynamic therapy, J. Am. Chem. Soc., 2020, 142, 6527–6537 CrossRef CAS PubMed.
- J. An, Y. G. Hu, K. Cheng, C. Li, X. L. Hou, G. L. Wang, X. S. Zhang, B. Liu, Y. D. Zhao and M. Z. Zhang, ROS-augmented and tumor-microenvironment responsive biodegradable nanoplatform for enhancing chemo-sonodynamic therapy, Biomaterials, 2020, 234, 119761 CrossRef CAS PubMed.
- L. Lei, S. S. Cai, Y. Zhang, L. Y. Yang, J. Deng, H. Mei, X. Q. Zhang, K. Zhang, B. He and J. Cao, Structure inversion-bridged sequential amino acid metabolism disturbance potentiates photodynamic-evoked immunotherapy, Adv. Funct. Mater., 2022, 32, 2103394 CrossRef CAS.
- J. Zhang, F. Li, Y. Yin, N. Liu, M. Zhu, H. Zhang, W. Liu, M. Yang, S. Qin, X. Fan, Y. Yang, K. Zhang and F. Yu, Alpha radionuclide-chelated radioimmunotherapy promoters enable local radiotherapy/chemodynamic therapy to discourage cancer progression, Biomater. Res., 2022, 26, 44 CrossRef CAS PubMed.
- K. Zhang, Y. Fang, Y. P. He, H. H. Yin, X. Guan, Y. Y. Pu, B. G. Zhou, W. W. Yue, W. W. Ren, D. Du, H. Y. Li, C. Liu, L. P. Sun, Y. Chen and H. X. Xu, Extravascular gelation shrinkage-derived internal stress enables tumor starvation therapy with suppressed metastasis and recurrence, Nat. Commun., 2019, 10, 5380 CrossRef.
- Q. Hou, K. Zhang, S. Chen, J. Chen, Y. Zhang, N. Gong, W. Guo, C. Fang, L. Wang, J. Jiang, J. Dou, X. Liang, J. Yu and P. Liang, Physical & chemical microwave ablation (MWA) enabled by nonionic MWA nanosensitizers repress incomplete MWA-arised liver tumor recurrence, ACS Nano, 2022, 16, 5704–5718 CrossRef CAS PubMed.
- H. He, Z. Fei, T. Guo, Y. Hou, D. Li, K. Wang, F. Ren, K. Fan, D. Zhou, C. Xie, C. Wang and X. Lu, Bioadhesive injectable hydrogel with phenolic carbon quantum dot supported Pd single atom nanozymes as a localized immunomodulation niche for cancer catalytic immunotherapy, Biomaterials, 2022, 280, 121272 CrossRef CAS PubMed.
- S. A. Richman, S. Nunez-Cruz, B. Moghimi, L. Z. Li, Z. T. Gershenson, Z. Mourelatos, D. M. Barrett, S. A. Grupp and M. C. Milone, High-sffinity GD2-specific CAR T cells induce fatal encephalitis in a preclinical neuroblastoma model, Cancer Immunol. Res., 2018, 6, 36–46 CrossRef CAS PubMed.
- L. Zhu, J. Liu, G. Zhou, T. M. Liu, Y. Dai, G. Nie and Q. Zhao, Remodeling of tumor microenvironment by tumor-targeting nanozymes enhances immune activation of CAR T cells for combination Therapy, Small, 2021, 17, 2102624 CrossRef CAS PubMed.
- B. Xu, Y. Cui, W. Wang, S. Li, C. Lyu, S. Wang, W. Bao, H. Wang, M. Qin, Z. Liu, W. Wei and H. Liu, Immunomodulation-enhanced nanozyme-based tumor catalytic therapy, Adv. Mater., 2020, 32, 2003563 CrossRef CAS PubMed.
- Y. Zhao, X. Xiao, M. Zou, B. Ding, H. Xiao, M. Wang, F. Jiang, Z. Cheng, P. Ma and J. Lin, Nanozyme-initiated in situ cascade reactions for self-amplified biocatalytic immunotherapy, Adv. Mater., 2021, 33, 2006363 CrossRef CAS PubMed.
- I. Dalle-Donne, G. Aldini, M. Carini, R. Colombo, R. Rossi and A. Milzani, Protein carbonylation, cellular dysfunction, and disease progression, J. Cell. Mol. Med., 2006, 10, 389–406 CrossRef CAS PubMed.
- Y. W. Kim, X. Z. West and T. Byzova, Inflammation and oxidative stress in angiogenesis and vascular disease, J. Mol. Med., 2013, 91, 323–328 CrossRef CAS PubMed.
- S. Reuter, S. C. Gupta, M. M. Chaturvedi and B. B. Aggarwal, Oxidative stress, inflammation, and cancer: how are they linked?, Free Radic. Biol. Med., 2010, 49, 1603–1616 CrossRef CAS PubMed.
- Q. Li, Y. Liu, X. Dai, W. Jiang and H. Zhao, Nanozymes regulate redox homeostasis in ROS-related inflammation, Front. Chem., 2021, 9, 740607 CrossRef PubMed.
- Q. Wang, C. Cheng, S. Zhao, Q. Liu, Y. Zhang, W. Liu, X. Zhao, H. Zhang, J. Pu, S. Zhang, H. Zhang, Y. Du and H. Wei, A valence-engineered self-cascading antioxidant nanozyme for the therapy of inflammatory bowel disease, Angew. Chem., Int. Ed., 2022, 61, e202201101 CAS.
- R. Yuan, Y. Li, S. Han, X. Chen, J. Chen, J. He, H. Gao, Y. Yang, S. Yang and Y. Yang, Fe-curcumin nanozyme-mediated reactive oxygen species scavenging and anti-inflammation for acute lung injury, ACS Cent. Sci., 2022, 8, 10–21 CrossRef CAS PubMed.
- H. Eric, K. Ajay, S. Sudipta and S. William, The role of cerium redox state in the SOD mimetic activity of nanoceria, Biomaterials, 2008, 29, 2705–2709 CrossRef PubMed.
- M. M. T. Jansman, X. Liu, P. Kempen, G. Clergeaud, T. L. Andresen, P. W. Thulstrup and L. Hosta-Rigau, Hemoglobin-based oxygen carriers incorporating nanozymes for the depletion of reactive oxygen species, ACS Appl. Mater. Interfaces, 2020, 12, 50275–50286 CrossRef CAS PubMed.
- J. Kim, G. Hong, L. Mazaleuskaya, J. C. Hsu, D. N. Rosario-Berrios, T. Grosser, P. F. Cho-Park and D. P. Cormode, Ultrasmall antioxidant cerium oxide nanoparticles for regulation of acute inflammation, ACS Appl. Mater. Interfaces, 2021, 13, 60852–60864 CrossRef CAS PubMed.
- R. Zhang, B. Xue, Y. Tao, H. Zhao, Z. Zhang, X. Wang, X. Zhou, B. Jiang, Z. Yang, X. Yan and K. Fan, Edge-site engineering of defective Fe–N4 Nanozymes with boosted catalase-like performance for retinal vasculopathies, Adv. Mater., 2022, 34, 2205324 CrossRef CAS PubMed.
- J. Yang, R. Zhang, H. Zhao, H. Qi, J. Li, J. F. Li, X. Zhou, A. Wang, K. Fan, X. Yan and T. Zhang, Bioinspired copper single-atom nanozyme as a superoxide dismutase-like antioxidant for sepsis treatment, Exploration, 2022, 2, 20210267 CrossRef.
- E. B. Peters and M. R. Kibbe, Nanomaterials to resolve atherosclerosis, ACS Biomater. Sci. Eng., 2020, 6, 3693–3712 CrossRef CAS PubMed.
- N. Manners, V. Priya, A. K. Mehata, M. Rawat, S. Mohan, H. A. Makeen, M. Albratty, A. Albarrati, A. M. Meraya and M. S. Muthu, Theranostic nanomedicines for the treatment of cardiovascular and related diseases: current strategies and future perspectives, Pharmaceuticals, 2022, 15, 441 CrossRef CAS PubMed.
- N. A. Mohamed, I. Marei, S. Crovella and H. Abou-Saleh, Recent developments in nanomaterials-based drug delivery and upgrading treatment of cardiovascular diseases, Int. J. Mol. Sci., 2022, 23, 1404 CrossRef CAS PubMed.
- Y. Zhang, Y. Yin, W. Zhang, H. Li, T. Wang, H. Yin, L. Sun, C. Su, K. Zhang and H. Xu, Reactive oxygen species scavenging and inflammation mitigation enabled by biomimetic prussian blue analogues boycott atherosclerosis, J. Nanobiotechnol., 2021, 19, 161 CrossRef CAS PubMed.
- Y. Wang, L. Li, W. Zhao, Y. Dou, H. An, H. Tao, X. Xu, Y. Jia, S. Lu, J. Zhang and H. Hu, Targeted therapy of atherosclerosis by a broad-spectrum reactive oxygen species scavenging nanoparticle with intrinsic anti-inflammatory activity, ACS Nano, 2018, 12, 8943–8960 CrossRef CAS PubMed.
- S. Katsuki, T. Matoba, J. I. Koga, K. Nakano and K. Egashira, Anti-inflammatory nanomedicine for cardiovascular disease, Front. Cardiovasc. Med., 2017, 4, 87 CrossRef PubMed.
- M. P. Ferreira, V. Balasubramanian, J. Hirvonen, H. Ruskoaho and H. A. Santos, Advanced nanomedicines for the treatment and diagnosis of myocardial infarction and heart failure, Curr. Drug Targets, 2015, 16, 1682–1697 CrossRef CAS PubMed.
- G. Heusch, Myocardial ischaemia-reperfusion injury and cardioprotection in perspective, Nat. Rev. Cardiol., 2020, 17, 773–789 CrossRef PubMed.
- Y. Zhang, A. Khalique, X. Du, Z. Gao, J. Wu, X. Zhang, R. Zhang, Z. Sun, Q. Liu, Z. Xu, A. C. Midgley, L. Wang, X. Yan, J. Zhuang, D. Kong and X. Huang, Biomimetic design of mitochondria-targeted hybrid nanozymes as superoxide scavengers, Adv. Mater., 2021, 33, 2006570 CrossRef CAS PubMed.
- V. M. Martín Giménez, D. E. Kassuha and W. Manucha, Nanomedicine applied to cardiovascular diseases: latest developments, Ther. Adv. Cardiovasc. Dis., 2017, 11, 133–142 CrossRef PubMed.
- R. M. Touyz, Reactive oxygen species and angiotensin II signaling in vascular cells-implications in cardiovascular disease, Braz. J. Med. Biol. Res., 2004, 37, 1263–1273 CrossRef CAS PubMed.
- K. Savalia, D. S. Manickam, E. G. Rosenbaugh, J. Tian, I. M. Ahmad, A. V. Kabanov and M. C. Zimmerman, Neuronal uptake of nanoformulated superoxide dismutase and attenuation of angiotensin II-dependent hypertension after central administration, Free Radic. Biol. Med., 2014, 73, 299–307 CrossRef CAS PubMed.
- R. Stocker and J. F. Keaney Jr, Role of oxidative modifications in atherosclerosis, Physiol. Rev., 2004, 84, 1381–1478 CrossRef CAS PubMed.
- L. A. Sena and N. S. Chandel, Physiological roles of mitochondrial reactive oxygen species, Mol. Cell, 2012, 48, 158–167 CrossRef CAS PubMed.
- G. Gloire, S. Legrand-Poels and J. Piette, NF-kappaB activation by reactive oxygen species: fifteen years later, Biochem. Pharmacol., 2006, 72, 1493–1505 CrossRef CAS PubMed.
- M. Mittal, M. R. Siddiqui, K. Tran, S. P. Reddy and A. B. Malik, Reactive oxygen species in inflammation and tissue injury, Antioxid. Redox Signal., 2014, 20, 1126–1167 CrossRef CAS PubMed.
- P. A. Grimsrud, H. Xie, T. J. Griffin and D. A. Bernlohr, Oxidative stress and covalent modification of protein with bioactive aldehydes, J. Biol. Chem., 2008, 283, 21837–21841 CrossRef CAS PubMed.
- A. J. Kattoor, N. V. K. Pothineni, D. Palagiri and J. L. Mehta, Oxidative stress in atherosclerosis, Curr. Atheroscler. Rep., 2017, 19, 42 CrossRef PubMed.
- U. Forstermann, N. Xia and H. Li, Roles of vascular oxidative stress and nitric oxide in the pathogenesis of atherosclerosis, Circ. Res., 2017, 120, 713–735 CrossRef PubMed.
- T. Munzel, G. G. Camici, C. Maack, N. R. Bonetti, V. Fuster and J. C. Kovacic, Impact of oxidative stress on the heart and vasculature: part 2 of a 3-part series, J. Am. Coll. Cardiol., 2017, 70, 212–229 CrossRef PubMed.
- K. K. Griendling, D. Sorescu, B. Lassègue and M. Ushio-Fukai, Modulation of protein kinase activity and gene expression by reactive oxygen species and their role in vascular physiology and pathophysiology, Arterioscler., Thromb., Vasc. Biol., 2000, 20, 2175–2183 CrossRef CAS PubMed.
- H. Li, S. Horke and U. Forstermann, Vascular oxidative stress, nitric oxide and atherosclerosis, Atherosclerosis, 2014, 237, 208–219 CrossRef CAS PubMed.
- Y. Wang, M. Subramanian, A. Yurdagul, V. C. Barbosa-Lorenzi, B. Cai, J. de Juan-Sanz, T. A. Ryan, M. Nomura, F. R. Maxfield and I. Tabas, Mitochondrial fission promotes the continued clearance of apoptotic cells by macrophages, Cell, 2017, 171, 331–345 CrossRef CAS PubMed.
- C. S. Wilcox, Effects of tempol and redox-cycling nitroxides in models of oxidative stress, Pharmacol. Ther., 2010, 126, 119–145 CrossRef CAS PubMed.
- D. N. Granger and P. R. Kvietys, Reperfusion injury and reactive oxygen species: The evolution of a concept, Redox Biol., 2015, 6, 524–551 CrossRef CAS PubMed.
- K. Zhang, M. Tu, W. Gao, X. Cai, F. Song, Z. Chen, Q. Zhang, J. Wang, C. Jin, J. Shi, X. Yang, Y. Zhu, W. Gu, B. Hu, Y. Zheng, H. Zhang and M. Tian, Hollow prussian blue nanozymes drive Neuroprotection against ischemic stroke via attenuating oxidative stress, counteracting inflammation, and suppressing cell apoptosis, Nano Lett., 2019, 19, 2812–2823 CrossRef CAS PubMed.
- Y. Liu, X. Wang, X. Li, S. Qiao, G. Huang, D. M. Hermann, T. R. Doeppner, M. Zeng, W. Liu, G. Xu, L. Ren, Y. Zhang, W. Liu, E. Casals, W. Li and Y. C. Wang, A co-doped Fe3O4 nanozyme shows enhanced reactive oxygen and nitrogen species scavenging activity and ameliorates the deleterious effects of ischemic stroke, ACS Appl. Mater. Interfaces, 2021, 13, 46213–46224 CrossRef CAS PubMed.
- S. Guo and L. Guo, Unraveling the multi-enzyme-like activities of iron oxide nanozyme via a first-principles microkinetic study, J. Phys. Chem. C, 2019, 123, 30318–30334 CrossRef CAS.
- J. L. Cummings and G. Cole, Alzheimer disease, JAMA, 2002, 287, 2335–2338 CrossRef CAS PubMed.
- H. Liu, C. Qian, T. Yang, Y. Wang, J. Luo, C. Zhang, X. Wang, X. Wang and Z. Guo, Small molecule-mediated co-assembly of amyloid-β oligomers reduces neurotoxicity through promoting non-fibrillar aggregation, Chem. Sci., 2020, 11, 7158–7169 RSC.
- M. J. McManus, M. P. Murphy and J. L. Franklin, The mitochondria-targeted antioxidant MitoQ prevents loss of spatial memory retention and early neuropathology in a transgenic mouse model of Alzheimer's disease, J. Neurosci., 2011, 31, 15703–15715 CrossRef CAS PubMed.
- Z. Jia, X. Yuan, J. A. Wei, X. Guo, Y. Gong, J. Li, H. Zhou, L. Zhang and J. Liu, A functionalized octahedral palladium nanozyme as a radical scavenger for ameliorating Alzheimer's disease, ACS Appl. Mater. Interfaces, 2021, 13, 49602–49613 CrossRef CAS PubMed.
- M. Ma, Z. Liu, N. Gao, Z. Pi, X. Du, J. Ren and X. Qu, Self-protecting biomimetic nanozyme for selective and synergistic clearance of peripheral amyloid-β in an Alzheimer's disease Model, J. Am. Chem. Soc., 2020, 142, 21702–21711 CrossRef CAS PubMed.
- S. Wang, Y. H. Yuan, N. H. Chen and H. B. Wang, The mechanisms of NLRP3 inflammasome/pyroptosis activation and their role in Parkinson's disease, Int. Immunopharmacol., 2019, 67, 458–464 CrossRef CAS PubMed.
- N. Kelley, D. Jeltema, Y. Duan and Y. He, The NLRP3 Inflammasome: an overview of mechanisms of activation and regulation, Int. J. Mol. Sci., 2019, 20, 3328 CrossRef CAS PubMed.
- R. Que, J. Zheng, Z. Chang, W. Zhang, H. Li, Z. Xie, Z. Huang, H. T. Wang, J. Xu, D. Jin, W. Yang, E. K. Tan and Q. Wang, Dl-3-n-butylphthalide rescues dopaminergic neurons in Parkinson's disease models by inhibiting the NLRP3 inflammasome and ameliorating mitochondrial impairment, Front. Immunol., 2021, 12, 794770 CrossRef CAS PubMed.
- X. Ma, J. Hao, J. Wu, Y. Li, X. Cai and Y. Zheng, Prussian blue nanozyme as a pyroptosis inhibitor alleviates neurodegeneration, Adv. Mater., 2022, 34, 2106723 CrossRef CAS PubMed.
- K. Blennow, D. L. Brody, P. M. Kochanek, H. Levin, A. McKee, G. M. Ribbers, K. Yaffe and H. Zetterberg, Traumatic brain injuries, Nat. Rev. Dis. Primers., 2016, 2, 16084 CrossRef PubMed.
- L. Nie, D. Mei, H. Xiong, B. Peng, Z. Ren, X. I. P. Hernandez, A. DeLaRiva, M. Wang, M. H. Engelhard, L. Kovarik, A. K. Datye and Y. Wang, Activation of surface lattice oxygen in single-atom Pt/CeO2 for low-temperature CO oxidation, Science, 2017, 358, 1419–1423 CrossRef CAS PubMed.
- F. Dvořák, M. Farnesi Camellone, A. Tovt, N. D. Tran, F. R. Negreiros, M. Vorokhta, T. Skála, I. Matolínová, J. Mysliveček, V. Matolín and S. Fabris, Creating single-atom Pt-ceria catalysts by surface step decoration, Nat. Commun., 2016, 7, 10801 CrossRef PubMed.
- R. Yan, S. Sun, J. Yang, W. Long, J. Wang, X. Mu, Q. Li, W. Hao, S. Zhang, H. Liu, Y. Gao, L. Ouyang, J. Chen, S. Liu, X. D. Zhang and D. Ming, Nanozyme-based bandage with single-atom catalysis for brain trauma, ACS Nano, 2019, 13, 11552–11560 CrossRef CAS PubMed.
- S. Zhang, Y. Liu, S. Sun, J. Wang, Q. Li, R. Yan, Y. Gao, H. Liu, S. Liu, W. Hao, H. Dai, C. Liu, Y. Sun, W. Long, X. Mu and X. D. Zhang, Catalytic patch with redox Cr/CeO2 nanozyme of noninvasive intervention for brain trauma, Theranostics, 2021, 11, 2806–2821 CrossRef CAS PubMed.
- M. Soh, D. M. Kang, H. G. Jeong, D. Kim, D. Y. Kim, W. Yang, C. Song, S. Baik, I. Y. Choi, S. K. Ki, H. J. Kwon, T. Kim, C. K. Kim, S. H. Lee and T. Hyeon, Ceria-zirconia nanoparticles as an enhanced multi-antioxidant for sepsis treatment, Angew. Chem., Int. Ed., 2017, 56, 11399–11403 CrossRef CAS PubMed.
- M. Chen, H. Liao, Z. Bu, D. Wang, C. Fang, X. Liang, H. Li, J. Liu, K. Zhang and D. Su, Pyroptosis activation by photodynamic-boosted nanocatalytic medicine favors malignancy recession, Chem. Eng. J., 2022, 441, 136030 CrossRef CAS.
- Q. Liu, W. Zhang, R. Jiao, Z. Lv, X. Lin, Y. Xiao and K. Zhang, Rational nanomedicine design enhances clinically physical treatment-inspired or combined immunotherapy, Adv. Sci., 2022, 9, 2203921 CrossRef CAS PubMed.
- H. Wei and E. Wang, Fe3O4 magnetic nanoparticles as peroxidase mimetics and their applications in H2O2 and glucose detection, Anal. Chem., 2008, 80, 2250–2254 CrossRef CAS PubMed.
- K. Fan, H. Wang, J. Xi, Q. Liu, X. Meng, D. Duan, L. Gao and X. Yan, Optimization of Fe3O4 nanozyme activity via single amino acid modification mimicking an enzyme active site, Chem. Commun., 2017, 53, 424–427 RSC.
- H. Wang, K. Wan and X. Shi, Recent advances in nanozyme research, Adv.
Mater., 2019, 31, 1805368 CrossRef CAS PubMed.
- Y. Fang, H. Y. Li, H. H. Yin, S. H. Xu, W. W. Ren, S. S. Ding, W. Z. Tang, L. H. Xiang, R. Wu, X. Guan and K. Zhang, Radiofrequency-sensitive longitudinal relaxation tuning strategy enabling the visualization of radiofrequency ablation intensified by magnetic composite, ACS Appl. Mater. Interfaces, 2019, 11, 11251–11261 CrossRef CAS PubMed.
- L. Lu, T. Wang, C. Fang, L. Song, C. Qian, Z. Lv, Y. Fang, X. Liu, X. Yu, X. Xu, C. Su, F. Chen and K. Zhang, Oncolytic impediment/promotion balance disruption by sonosensitizer-free Nanoplatforms unfreezes autophagy-induced resistance to sonocatalytic therapy, ACS Appl. Mater. Interfaces, 2022, 14, 36462–36472 CrossRef CAS PubMed.
- X. Guan, H. H. Yin, X. H. Xu, G. Xu, Y. Zhang, B. G. Zhou, W. W. Yue, C. Liu, L. P. Sun, H. X. Xu and K. Zhang, Tumor metabolism-engineered composite nanoplatforms potentiate sonodynamic therapy via reshaping tumor microenvironment and facilitating electron-hole pairs' separation, Adv. Funct. Mater., 2020, 30, 2000326 CrossRef CAS.
- K. Zhang, H. Y. Li, J. Y. Lang, X. T. Li, W. W. Yue, Y. F. Yin, D. Du, Y. Fang, H. Wu, Y. X. Zhao and C. Xu, Quantum yield-engineered biocompatible probes illuminate lung tumor based on viscosity confinement-mediated antiaggregation, Adv. Funct. Mater., 2019, 29, 1905124 CrossRef CAS.
- M. Yang, Y. Zhang, C. Fang, L. Song, Y. Wang, L. Lu, R. X. Yang, Z. T. Bu, X. Y. Liang, K. Zhang and Q. Fu, Urine-microenvironment-initiated composite hydrogel patch reconfiguration propels scarless memory repair and reinvigoration of the urethra, Adv. Mater., 2022, 34, 2109522 CrossRef CAS PubMed.
- F. L. Kong, C. Fang, Y. Zhang, L. X. Duan, D. Du, G. Xu, X. L. Li, H. Y. Li, Y. F. Yin, H. X. Xu and K. Zhang, Abundance and metabolism disruptions of intratumoral microbiota by chemical and physical actions unfreeze tumor treatment resistance, Adv. Sci., 2022, 9, 2105523 CrossRef CAS PubMed.
- T. Luo, D. Wang, L. D. Liu, Y. Zhang, C. Y. Han, Y. Xie, Y. Liu, J. C. Liang, G. H. Qiu, H. X. Li, D. K. Su, J. J. Liu and K. Zhang, Switching reactive oxygen species into reactive nitrogen species by photocleaved O-2-released nanoplatforms favors hypoxic tumor repression, Adv. Sci., 2021, 8, 2101065 CrossRef CAS PubMed.
- H. Wu, H. Y. Li, Y. Q. Liu, J. C. Liang, Q. S. Liu, Z. G. Xu, Z. Z. Chen, X. Zhang, K. Zhang and C. A. Xu, Blockading a new NSCLC immunosuppressive target by pluripotent autologous tumor vaccines magnifies sequential immunotherapy, Bioact. Mater., 2022, 13, 223–238 CrossRef CAS PubMed.
- X. Liang, Y. Zhang, J. Zhou, Z. Bu, J. Liu and K. Zhang, Tumor microenvironment-triggered intratumoral in situ construction of theranostic supramolecular self-assembly, Coord. Chem. Rev., 2022, 473, 214824 CrossRef CAS.
- T. X. Wang, X. H. Xu and K. Zhang, Nanotechnology-enabled chemodynamic therapy and immunotherapy, Curr. Cancer Drug Targets, 2021, 21, 545–557 CrossRef CAS PubMed.
- Z. Zeng, H. L. Gao, C. X. Chen, L. B. Xiao and K. Zhang, Bioresponsive nanomaterials: recent advances in cancer multimodal imaging and imaging-guided therapy, Front. Chem., 2022, 10, 881812 CrossRef CAS PubMed.
- H. Mei, X. X. Zhang, S. S. Cai, X. Q. Zhang, Y. Zhang, Z. Y. Guo, W. X. Shi, R. R. Chu, K. Zhang, J. Cao and B. He, Fluorocarbon-driven photosensitizer assembly decodes energy conversion pathway for suppressing breast tumor, Nano Today, 2021, 41, 101305 CrossRef CAS.
- Y. F. Yin, X. W. Jiang, L. P. Sun, H. Y. Li, C. X. Su, Y. Zhang, G. Xu, X. L. Li, C. K. Zhao, Y. Chen, H. X. Xu and K. Zhang, Continuous inertial cavitation evokes massive ROS for reinforcing sonodynamic therapy and immunogenic cell death against breast carcinoma, Nano Today, 2021, 36, 101009 CrossRef CAS.
- H. Cao, L. X. Duan, Y. Zhang, J. Cao and K. Zhang, Current hydrogel advances in physicochemical and biological response-driven biomedical application diversity, Signal Transduct. Target. Ther., 2021, 6, 426 CrossRef CAS PubMed.
- Y. Zhang, L. H. Guo, F. L. Kong, L. X. Duan, H. Y. Li, C. Fang and K. Zhang, Nanobiotechnology-enabled energy utilization elevation for augmenting minimally-invasive and noninvasive oncology thermal ablation, Wiley Interdiscip. Rev.: Nanomed. Nanobiotechnol., 2021, 13, e1733 Search PubMed.
- Y. Zhang, D. Du, C. Fang, X. Yu, Y. Fang, X. Liu, D. Ou, H. Yin, H. Liu, T. Wang, L. Lu, X. Li and K. Zhang, Epigenetics disruptions enabled by porphyrin-derived metal-organic frameworks disarm resistances to sonocatalytic ROS anti-tumor actions, Fundam. Res., 2022 DOI:10.1016/j.fmre.2022.1006.1020.
- L. Song, L. Lu, Y. Y. Pu, H. H. Yin and K. Zhang, Nanomaterials-based tumor microenvironment modulation for magnifying sonodynamic therapy, Acc. Mater. Res., 2022, 3, 971–985 CrossRef CAS.
- D. Wang, M. Zhang, Y. Zhang, G. Qiu, J. Chen, X. Zhu, C. Kong, X. Lu, X. Liang, L. Duan, C. Fang, J. Liu, K. Zhang and T. Luo, Intraparticle double-scattering-decoded sonogenetics for augmenting immune checkpoint blockade and CAR-T therapy, Adv. Sci., 2022, 9, 2203106 CrossRef CAS PubMed.
- J. Chen, X. Yu, X. Liu, J. Ni, G. Yang and K. Zhang, Advances in nanobiotechnology-propelled multidrug resistance circumvention of cancer, Nanoscale, 2022, 14, 12984–12998 RSC.
- Y. Huang, L. Zhong, X. Li, P. Wu, J. He, C. Tang, Z. Tang, J. Su, Z. Feng, B. Wang, Y. Ma, H. Peng, Z. Bai, Y. Zhong, Y. Liang, W. Lu, R. Luo, J. Li, H. Li, Z. Deng, X. Lan, Z. Liu, K. Zhang and Y. Zhao, In situ silver-based electrochemical oncolytic bioreactor, Adv. Mater., 2022, 34, 2109973 CrossRef CAS PubMed.
- Y. Zhang, W. Yin, H. Zhang, T. Li, H. Wang, L. Yin, C. Sun, K. Su, H. Zhang and J. Xu, Nanobiotechnol., 2021, 19, 161 CrossRef CAS PubMed.
- B. Kolli, N. Manne, R. Para, K. Siva and E. Blough, Cerium oxide nanoparticles attenuate monocrotaline induced right ventricular hypertrophy following pulmonary arterial hypertension, Biomaterials, 2014, 37, 9951–9962 CrossRef PubMed.
- Y. Zhang, W. Liu, X. Wang, Y. Liu and H. Wei, Nanozyme-Enabled Treatment of Cardio- and Cerebrovascular Diseases, Small, 2022, 3, e2204809 CrossRef PubMed.
- R. Tian, H. Ma, W. Ye, Y. Li, S. Wang, Z. Zhang, S. Liu, M. Zang, J. Hou, J. Xu, Q. Luo, H. Sun, F. Bai, Y. Yang and J. Liu, Se-Containing MOF Coated Dual-Fe-Atom Nanozymes With Multi-Enzyme Cascade Activities Protect Against Cerebral Ischemic Reperfusion Injury, Adv. Funct. Mater., 2022, 32, 2204025 CrossRef CAS.
- J. Kwon, J. Kim, S. Park, G. Khang, P. Kang and D. Lee, Inflammation-responsive Antioxidant Nanoparticles Based on a Polymeric Prodrug of Vanillin, Biomacromolecules, 2013, 14, 1618–1626 CrossRef CAS PubMed.
- Y. Li, Y. Li, H. Wang and R. Liu, Yb3 +, Er3+ Codoped Cerium Oxide Upconversion Nanoparticles Enhanced the Enzymelike Catalytic Activity and Antioxidative Activity for Parkinson's Disease Treatment, ACS Appl. Mater. Inter., 2021, 13, 13968–13977 CrossRef CAS PubMed.
- W. Cong, R. Bai, Y. Li, L. Wang and C. Chen, Huntington's disease-cardiovascular nanozyme, ACS Appl. Mater. Interfaces, 2019, 11, 34725 CrossRef CAS PubMed.
- H. He, X. Shi, J. Wang, X. Wang, Q. Wang and D. Yu, Reactive Oxygen Species-Induced Aggregation of Nanozymes for Neuron Injury, ACS Appl. Mater. Inter., 2020, 12, 209–216 CrossRef CAS PubMed.
- X. Mu, H. He, J. Wang, W. Long, Q. Li, H. Li, Y. Gao, L. Ouyang, Q. Ren, S. Sun, J. Wang, J. Yang, Q. Liu, Y. Sun, C. Liu, X. Zhang and W. Hu, Carbogenic Nanozyme with Ultrahigh Reactive Nitrogen Species Selectivity for Traumatic Brain Injury, Nano Lett., 2019, 19, 4527–4534 CrossRef CAS PubMed.
- M. Li, Q. Zhao, X. Yi, X. Zhong, G. Song, Z. Chai, Z. Liu and K. Yang, Au@MnS@ZnS Core/Shell/Shell Nanoparticles for Magnetic Resonance Imaging and Enhanced Cancer Radiation Therapy, ACS Appl. Mater. Interfaces, 2016, 8, 9557–9564 CrossRef CAS PubMed.
- C. R. Gordijo, A. J. Shuhendler and X. Wu, Glucose-Responsive Bioinorganic Nanohybrid Membrane for Self-Regulated Insulin Release, Adv. Funct. Mater., 2010, 9, 1404–1412 CrossRef.
- P. Prasad, C. R. Gordijo, A. Z. Abbasi, A. Maed, A. Ip and A. M. Rauth, Multifunctional Albumin-MnO Nanoparticles Modulate Solid Tumor Microenvironment by Attenuating Hypoxia, Acidosis, Vascular Endothelial Growth Factor and Enhance Radiation Response, ACS Nano, 2014, 8, 3202–3212 CrossRef CAS PubMed.
- B. Hu, X. Xiao, P. Chen, J. Qian, G. Yuan, Y. Ye, L. Zeng, S. Zhong, X. Wang, X. Qin, Y. Yang, Y. Pan and Y. Zhang, Enhancing anti-tumor effect of ultrasensitive bimetallic RuCu nanoparticles as radiosensitizers with dual enzyme-like activities, Biomaterials, 2022, 290, 121811 CrossRef CAS PubMed.
Footnote |
† These authors contributed equally to this work. |
|
This journal is © the Partner Organisations 2023 |
Click here to see how this site uses Cookies. View our privacy policy here.