DOI:
10.1039/D2QM00983H
(Research Article)
Mater. Chem. Front., 2023,
7, 65-71
LiRE(SO4)2 (RE = Y, Gd, Eu): noncentrosymmetric chiral rare-earth sulfates with very large band gaps†
Received
26th September 2022
, Accepted 21st October 2022
First published on 30th November 2022
Abstract
Three new ternary lithium rare earth metal sulfates, LiRE(SO4)2 (RE = Y, Gd, and Eu), have been synthesized via high temperature solid-state and solvothermal reactions. The reported materials crystallizing in the noncentrosymmetric (NCS) nonpolar tetragonal space group, P
n2 (no. 118) exhibit three-dimensional structures consisting of REO8, LiO4, and SO4 groups. Ultraviolet-visible diffuse reflectance spectra and density functional theory calculations indicate that the title materials exhibit very large band gaps of ca. 6.7 eV. The frameworks of the sulfates reveal high thermal stabilities of up to 873–1073 K depending on the constituting rare earth cations. Powder second-harmonic generation (SHG) measurements reveal that the sulfates with NCS structures have mild SHG responses of ca. 13 × α-SiO2 and are non-phase matchable. Photoluminescence experiments on LiEu(SO4)2 revealing a bright orange emission show multiple peaks originating from f–f transitions. Detailed synthetic procedures, crystal structures, and full characterization studies of the new sulfates are presented.
Introduction
Materials with noncentrosymmetric (NCS) structures are the most essential components for the second-order nonlinear optical (NLO) properties modulating and controlling frequency of solid-state lasers. Several crystalline materials such as KH2PO4,1 KTiOPO4,2 and β-BaB2O41 have been extensively utilized to generate second-harmonic lights with various wavelengths. However, discovering functional NLO materials that can be applied to a specific range, e.g., deep ultraviolet (DUV) area with shorter wavelengths of less than 200 nm, is an ongoing challenge. To generate a novel coherent DUV light, crystals must have wide band gaps (<200 nm of UV cutoff edge), moderate birefringence (Δn ≥ 0.053), and strong second harmonic generation (SHG) response. A few effective functional groups to fulfill the conditions originating from the structural anisotropy and electron density distribution include materials with well-aligned π-conjugated planar moieties such as BO33−, NO3−, and CO32−.3,4 A number of representative compounds consisting of π-conjugated planar groups with large birefringence and wide UV-cutoff window such as K2BeBO3F2,5 β-BaB2O4, LiB3O5,6 KSrCO3F,7 and Rb2Na(NO3)38 have been known thus far. Unfortunately, however, the materials are not widely utilized due to some critical drawbacks.9 For example, the benchmark DUV NLO material, K2BeBO3F2 not only exhibits a severe layering habit, but reveals an intrinsic toxicity attributable to beryllium.10 Meanwhile, crystals composed of non-π-conjugated tetrahedral groups such as PO43− and SO42− should be attractive potential DUV NLO materials because the constituting tetrahedra revealing lower interaction with other external groups can offer more stable framework structures with large band gaps.11–13 In addition, well-ordered tetrahedra also facilitate the crystallization of macroscopic NCS structures.14–20 With these ideas in mind, we have successfully synthesized pure polycrystalline samples and crystals of three ternary lithium rare-earth double sulfates, LiRE(SO4)2 (RE = Y, Eu, and Gd) by solid-state and hydrothermal reactions. In this paper, detailed characterizations on the novel NCS sulfate materials exhibiting very short UV-cutoff edges are presented along with their electronic structure calculations and photoluminescence properties.
Experimental section
Materials
LiNO3 (99%, Alfa Aesar), Li2SO4·H2O (99%, Alfa Aesar), Y2(SO4)3·8H2O (99.9%, Alfa Aesar), Eu2(SO4)3·8H2O (99.9%, Alfa Aesar), Gd2(SO4)3·8H2O (99.9%, Alfa Aesar), HNO3 (60%, Samchun), and H2SO4 (95%, Samchun) were used without further purification.
Synthesis
Polycrystalline samples were prepared via conventional solid-state reactions. Stoichiometric amounts of Li2SO4·H2O and RE2(SO4)3·8H2O (RE = Y, Gd, and Eu) were thoroughly mixed using an agate mortar and pestle. The well-ground reaction mixtures were heated at 600 °C for 12 h and cooled to room temperature by turning off the furnaces. For LiGd(SO4)2, an additional 12 h heating and intermediate regrinding were required to synthesize the pure product. Large single crystals of the title compounds were grown by hydrothermal reactions. 1 mmol of RE2(SO4)3·8H2O and 4 mmol of LiNO3 were placed in 18 mL Teflon liners with 0.2 mL of HNO3, 0.5 mL of H2SO4, and 0.5 mL of distilled water. After tightly sealing, the autoclaves were heated to 230 °C for 72 h and cooled to room temperature at 6 °C h−1. Millimeter-sized large crystals of LiY(SO4)2, LiGd(SO4)2, and LiEu(SO4)2 were isolated via vacuum filtration in 89.5%, 80.3% and 84.2% yields, respectively, based on RE2(SO4)3·8H2O.
Measurements
Single crystal X-ray diffraction (SCXRD) data were collected at room temperature using a Bruker D8 Quest diffractometer with a graphite monochromated radiation source (Mo Kα, λ = 0.71073 Å) at the Advanced Bio-interface Core Research Facility, Sogang University. A colorless prism-shaped crystal for LiY(SO4)2 (0.038 mm × 0.127 mm × 0.206 mm), a colorless prism-shaped crystal for LiGd(SO4)2 (0.058 mm × 0.070 mm × 0.103 mm), and a pale red prism-shaped crystal for LiEu(SO4)2 (0.072 mm × 0.180 mm × 0.189 mm) were used for the data collections. After integrating the collected data using the SAINT program,21 absorption corrections were applied using the SADABS program.22 The structures were solved using SHELXS-201323 and refined using SHELXL-201324 software implemented in the WinGX-2014 program.25
Powder X-ray diffraction (PXRD) patterns were obtained on a Rigaku Miniflex 600 (Cu Kα, λ = 1.54056 Å) at room temperature. Well-ground polycrystalline samples mounted on sample holders were scanned in the 2θ range of 5–70° with a scan speed of 20° min−1 and a scan step width of 0.02°.
Ultraviolet-visible (UV-vis) diffuse reflectance spectra were obtained from 185 to 900 nm with 1 nm intervals using a PerkinElmer Lambda 1050 spectrophotometer.
Infrared (IR) spectra for the title compounds were obtained using a Thermo Scientific Nicolet iS50 FT-IR spectrometer with an attenuated total reflection accessory in the range of 400–4000 cm−1.
Thermogravimetric analysis (TGA) data were collected using a SCINCO TGA-N 1000 thermal analyzer. Polycrystalline samples were loaded in alumina crucibles and heated from 30 °C to 900 °C at a rate of 10 °C min−1 under the flowing argon gas.
Density functional theory (DFT) calculations were performed using a Quantum Espresso program package.26 The ultrasoft pseudopotential and Perdew–Burke–Ernzerhof (PBE) functional27 with the nonlinear core correction28 were used to calculate electronic structures of lithium rare-earth metal double sulfates. The kinetic energy and charge density cutoff were set to 46.65 and 419.85 Ry, respectively, for LiY(SO4)2, 122.08 and 1098.76 Ry for LiEu(SO4)2, and 116.606 and 1049.45 Ry for LiGd(SO4)2. The self-consistent function convergence thresholds for the reported compounds were set to about 10−6 Ry. The 4 × 4 × 6 k-point grids of the Brillouin zone were used for all compounds.
Powder SHG measurements were performed by using a modified Kurtz NLO system.29 Ground polycrystalline samples were sieved into the particle size range of <20, 20–45, 45–63, 63–75, 75–90, 90–125, 125–150, 150–200, and 200–250 μm to investigate the correlation between SHG responses and particle sizes. The sieved samples were packed into the respective capillaries (i.d. = 1.8 mm and o.d. = 3.00 mm) and irradiated through a DAWA Q-switched Nd:YAG laser (1064 nm). The SHG light (532 nm) was collected to a Hamamatsu photomultiplier tube and detected using a Tektronix TDS-2012C oscilloscope.
The photoluminescence (PL) properties of LiEu(SO4)2 were investigated by a Jasco FP-8550 with a xenon lamp at room temperature. The excitation spectrum under 613 nm emission was collected in the range of 200–500 nm to obtain charge transfer bands. And the emission spectrum under 396 nm excitation was collected in the range of 550–750 nm. The photoluminescence quantum yield (PLQY) and lifetime were measured by using an integrating sphere with a 396 nm excitation wavelength. The decay curve was recorded for 613 nm and the single exponential function was used to calculate the lifetime.30
Results and discussion
Structural description
Although it was reported that LiEu(SO4)2 crystallized in the polar NCS orthorhombic space group, Pnn2 (no. 34),31 our SCXRD analyses indicate that the three title compounds with similar structures crystallize in the nonpolar NCS tetragonal space group, P
n2 (no. 118) (Table 1). Therefore, the structure of LiY(SO4)2 will be described here as a representative for the whole title compounds. The three-dimensional framework of LiY(SO4)2 consists of YO8 dodecahedra, SO4 tetrahedra, and LiO4 tetrahedra. In an asymmetric unit, one Li+ cation, one Y3+ cation, one S6+ cation, and two O2− anions are present (Fig. 1). Each Y3+ cation is coordinated by four O(1) and four O(2) atoms to form YO8 dodecahedra with the Y–O(1) and Y–O(2) distances of 2.2921(16) and 2.4449(15) Å, respectively. While S6+ cation is linked by two O(1) and two O(2) in SO4 tetrahedral environment with the S–O(1) and S–O(2) lengths of 1.4622(16) and 1.4839(14) Å, respectively, Li+ cation interacts with four O(2) with the Li–O(2) contact distance of 1.9172(15) Å in the LiO4 tetrahedral moiety (Fig. 1a and Table S1, ESI†). Fundamental building blocks (FBBs) of LiY(SO4)2 consist of one YO8 dodecahedron and six corner- and edge-sharing SO4 tetrahedra through oxygen atoms. The helical structure of FBBs can be easily identified by the corner-shared SO4 tetrahedra linked in the same positions along the b-axis. Further linkage of six sulfate groups in each chiral unit to another YO8 dodecahedron results in a pseudo-layered structure where YO8 and SO4 units are in an alternately parallel arrangement (Fig. 1b and c). Within the assembled pseudo-layered backbones, eight-membered rings (8-MRs) composed of four YO8 and SO4 are observed, in which the rings rotate in the clockwise direction and grow up helically along the c-axis. Li+ cations reside in channels constructed by the stacked rings along the c-direction (Fig. 2a). The structure can be also described as an anionic framework composed of LiO4 and SO4 tetrahedra with charge balancing Y3+ cations (Fig. 2b). The whole crystal constructs an isotropic and nonpolar structure because the helical structure does not make any net polarization, which is consistent with the compound's nonpolar space group. Although LiGd(SO4)2 and LiEu(SO4)2 reveal the same crystal structures as that of LiY(SO4)2, their unit cell volumes are 2.72% and 3.62% larger, respectively, than that of LiY(SO4)2. Bond valence sum (BVS) calculations performed on Li+, Y3+, Gd3+, Eu3+, S6+, and O2− for all three compounds result in values of 1.12–1.18, 3.13, 3.26, 3.24, 5.99–6.01, and 2.02–2.07, respectively.
Table 1 Crystallographic data for LiRE(SO4)2 (RE = Y, Gd, and Eu)
R(Fo) = ∑‖Fo| − |Fc‖/∑|Fo|.
R
w(Fo2) = {∑w[(Fo)2 − (Fc)2]2/∑w[(Fo)2]2 }1/2.
|
Compound |
LiY(SO4)2 |
LiGd(SO4)2 |
LiEu(SO4)2 |
fw |
287.97 |
356.31 |
351.02 |
Space group |
P n2 (no. 118) |
P n2 (no. 118) |
P n2 (no. 118) |
a = b (Å) |
7.57390(10) |
7.6335(3) |
7.6490(2) |
c (Å) |
5.4854(2) |
5.5514(3) |
5.57730(10) |
V (Å3) |
314.664(14) |
323.48(3) |
326.312(18) |
Z
|
2 |
2 |
2 |
D
calc. (g cm−3) |
3.039 |
3.656 |
3.573 |
λ (Å) |
0.71073 |
0.71073 |
0.71073 |
T (K) |
298.0(2) |
298.0(2) |
298.0(2) |
R(Fo)a |
0.0096 |
0.0072 |
0.0072 |
R
w(Fo2)b |
0.0273 |
0.0178 |
0.0188 |
Flack x |
0.001(5) |
0.032(12) |
0.004(10) |
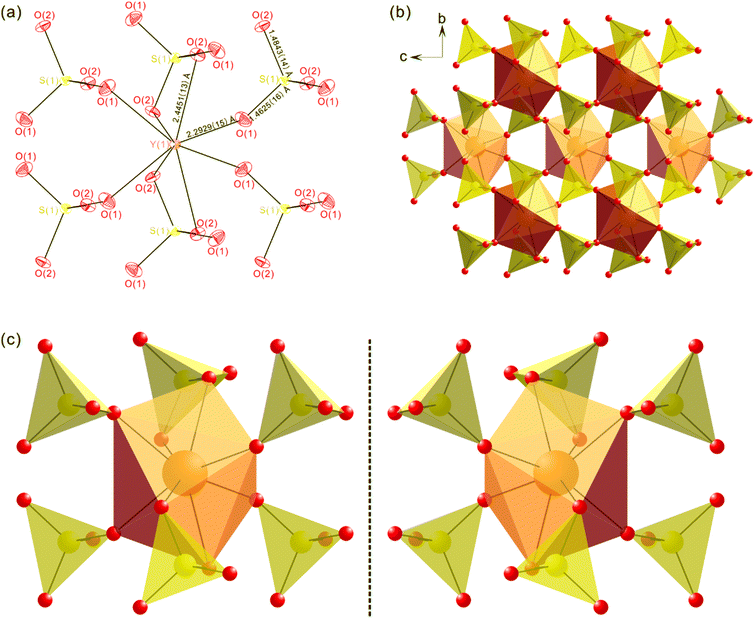 |
| Fig. 1 (a) ORTEP (50% probability ellipsoids) drawing for each polyhedra, YO8 and SO4 in the FBB of LiY(SO4)2. (b) Polyhedral representation of an alternately repeating pseudo-layer with parallel YO8 dodecahedra and SO4 tetrahedra of LiY(SO4)2 in the bc-plane (orange, Y; yellow, S; and red, O). (c) Each chiral FBB of LiY(SO4)2 is presented. | |
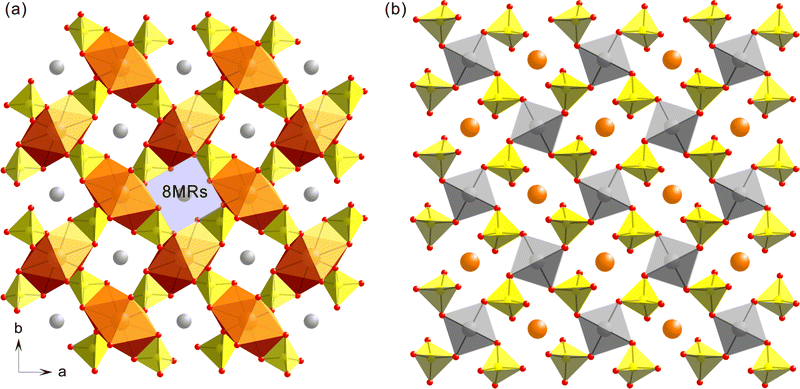 |
| Fig. 2 (a) Polyhedral representation of LiY(SO4)2 in the ab-plane (grey, Li; orange, Y; yellow, S; and red, O). Eight-membered rings (8MRs) composed of four YO8 and SO4 are observed. (b) A structural representation of LiY(SO4)2 with an anionic framework composed of LiO4 and SO4 tetrahedra with charge balancing Y3+ cations. | |
UV-Vis diffuse reflectance spectroscopy
UV-Vis diffuse reflectance spectra of all three title compounds reveal reflectance at ca. 230–245 nm. While LiY(SO4)2 and LiGd(SO4)2 reflect more than 60% of light from 900 nm to 185 nm, LiEu(SO4)2 absorbs ca. 83% of light at 230 nm (Fig. 3). The large band gaps of the compounds may be attributed to the combination of the nonconjugated SO4 tetrahedra, alkali metal containing LiO4 tetrahedra, and the polyhedra of rare earth metal cations. The observed red-shift of the UV cutoff edge for LiEu(SO4)2 might be originating from the charge transfer between O 2p orbitals and partially filled Eu3+ 4f orbitals. Half-filled f7 orbitals of trivalent gadolinium cations are much more stable than those of Eu3+ with incompletely filled f6 orbitals.32
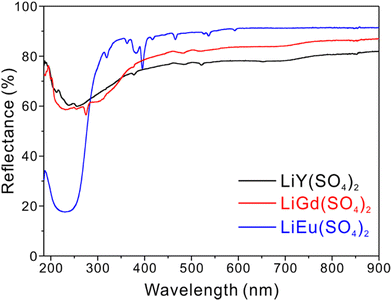 |
| Fig. 3 UV-Vis reflectance spectra of LiRE(SO4)2 (RE = Y, Gd, and Eu). | |
IR spectroscopy
Three isostructural title compounds exhibit similar IR absorption bands arising from the corresponding bonds (Fig. S2, ESI†). Bands observed at 1000–1150 cm−1 are assigned to S–O bonds of sulfate tetrahedra. And absorption peaks at 650–700 cm−1, 400–500 cm−1, and 600–650 cm−1 may be originating from the RE–O and Li–O bonds, respectively. The assignment of the observed vibrations are consistent with the reported data.33
TGA
TGA indicates that all three title compounds exhibit no significant weight loss up to ca. 800 °C (Fig. S3, ESI†). However, PXRD data for samples measured after heating to high temperature indicate that LiY(SO4)2 and LiRE(SO4)2 (RE = Gd and Eu) start decomposing at 650 and 820 °C, respectively. For LiGd(SO4)2 and LiEu(SO4)2, thermally decomposed main residues turned out to be Gd2O2SO4 and Eu2O2SO4, respectively, along with some Li2SO4 based on PXRD (Fig. S4, ESI†).34 Based on the similar PXRD pattern, the major thermal decomposition products of LiY(SO4)2 are assumed to be Y2O2SO4 and Li2SO4 although the diffraction pattern of Y2O2SO4 has not been reported. Higher decomposition temperatures of LiGd(SO4)2 and LiEu(SO4)2 might be attributable to the stronger corresponding RE–O bonds. Also, the title compounds are assumed to decompose by losing the volatile Li slowly at high temperature. For example, LiY(SO4)2 starts decomposing at 650 °C; however, the framework of the material is still observed if the material is heated at 1000 °C for 2 h. Only after heating the material at 1000 °C for 14 h, a complete decomposition occurs based on the PXRD patterns (Fig. S5, ESI†).
DFT calculations
Calculations using the DFT method have been performed to study electronic structures and band gaps of the title compounds. By doing so, indirect band gaps of LiY(SO4)2, LiGd(SO4)2, and LiEu(SO4)2 were calculated to be 6.00 eV, 5.87 eV, and 4.52 eV, respectively (Fig. S6, ESI†), in which the corresponding UV cutoff edges are 206.8 nm, 211.4 nm, and 274.5 nm, respectively. The underestimation of calculated band gaps in the PBE functional compared to those of experimental values is well-documented.35 LiEu(SO4)2 has a significantly narrower band gap than those of LiY(SO4)2 and LiGd(SO4)2 because of the energy level difference in 4f orbitals for rare earth metal cations. In Eu3+ cations, partially filled 4f6 orbitals contribute to both valence band maximum (VBM) and conduction band minimum (CBM) and allow f–f transitions (Fig. S7, ESI†). On the other hand, the energy levels of half-filled 4f7 orbitals for Gd3+ are in the lower position than that of VBM and thus they are much more stable than those of 4f6 orbitals for Eu3+. The 2p orbitals of O2− mainly contribute to VBM in all three title compounds.
Powder SHG measurements
SHG properties of the title compounds have been investigated using the Kurtz–Perry powder method. The reported materials exhibit SHG responses of ca. 13 times that of α-SiO2 and are type-I nonphase-matchable at 1064 nm radiation (Fig. 4). It is not surprising that the title materials consisting of alternately aligned REO8 dodecahedra, SO4 tetrahedra, and LiO4 tetrahedra exhibit mild SHG efficiencies because the net moments effectively cancel in the nonpolar space group. The calculated birefringence of LiY(SO4)2 is ca. 0.028 at 532 nm, which also supports well the fact that the title compounds are nonphase-matchable at 1064 nm.
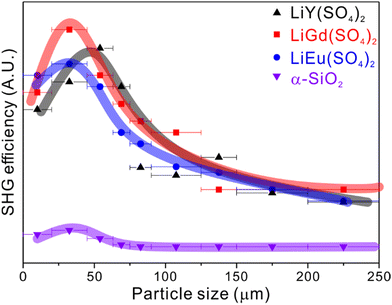 |
| Fig. 4 Plots of SHG versus particle size for LiRE(SO4)2 (RE = Y, Gd, and Eu). | |
PL spectroscopy
PL experiments of LiEu(SO4)2 reveal a bright orange emission, which corresponds to the strongest emission at 613 nm induced by 396 nm of excitation (Fig. 5). Specifically, the excitation spectrum emitting 613 nm shows peaks at ca. 287, 299, 320, 363, 382, 396, 417, and 466 nm, which can be assigned to f–f transitions of 7F0 → 5G5, 7F0 → 5G4, 7F0 → 5G3, 7F0 → 5D4, 7F0 → 5G2, 7F0 → 5L6, 7F0 → 5D3, and 7F0 → 5D2, respectively. The broad band occurring at ca. 260 nm is charge transfer arising from O 2p and Eu 4f orbitals (Fig. 5a). The emission spectrum induced by 396 nm exhibits peaks at ca. 592, 613, 658, and 699 nm, which is attributable to 5D0 → 7F1, 5D0 → 7F2, 5D0 → 7F3, and 5D0 → 7F4, respectively (Fig. 5b).33,36 LiEu(SO4)2 reveals a strong external PLQY of ca. 39.8% and long lifetime of ca. 3.6 ms once the material is irradiated by 396 nm radiation (Fig. 5c). The observed bright orange light can be depicted in the CIE 1931 diagram with the emissive coordinates of (0.65, 0.35) (Fig. 5d).
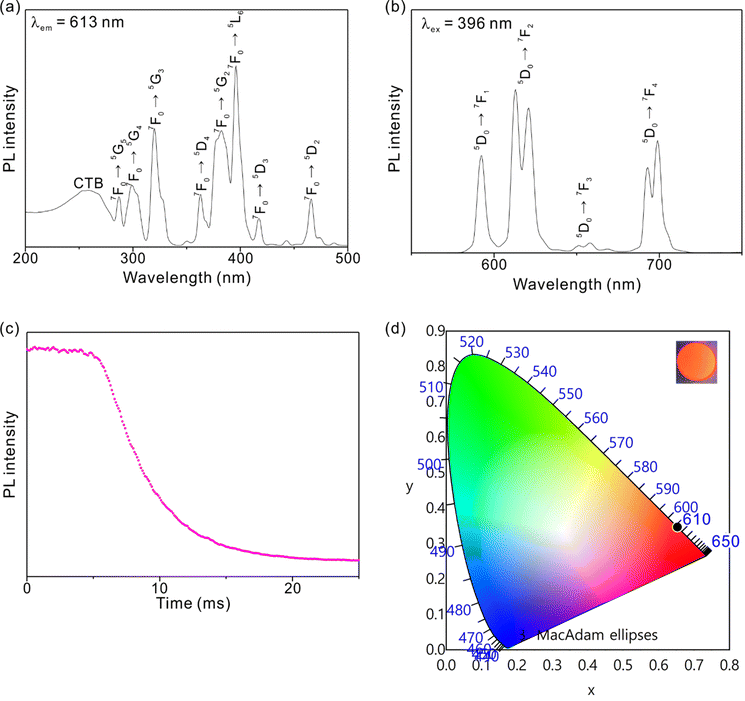 |
| Fig. 5 Photoluminescence (a) excitation and (b) emission spectra, (c) PL decay curve, and (d) CIE diagram for LiEu(SO4)2. | |
Conclusions
Pure polycrystalline samples and large crystals of three ternary lithium rare-earth double sulfates, LiRE(SO4)2 (RE = Y, Gd, and Eu) have been synthesized by solid-state reactions and solvothermal methods. The reported isostructural materials crystallizing in the NCS nonpolar space group, P
n2, reveal 3D chiral frameworks with REO8, SO4, and LiO4 fundamental building units. LiRE(SO4)2 reveal very short UV cutoff windows owing to the rigid SO4 tetrahedra and REO8 dodecahedra. LiRE(SO4)2 exhibit SHG responses of ca. 13 times that of α-SiO2 and are type-I nonphase-matchable attributable to the constituting nonpolarizable rare-earth cations and non-π conjugated sulfate tetrahedra. The PL spectrum of LiEu(SO4)2 revealed a strong fluorescence excited by light with very short wavelengths under 300 nm due to its short UV cutoff edge.
Conflicts of interest
There are no conflicts to declare.
Acknowledgements
This research was supported by the National Research Foundation of Korea (NRF) funded by the Ministry of Science and ICT (Grant No. 2018R1A5A1025208 and 2019R1A2C3005530).
References
-
V. G. Dmitriev, G. G. Gurzadyan and D. N. Nikogosyan, Handbook of nonlinear optical crystals, Springer, 2013 Search PubMed
.
- J. D. Bierlein and H. Vanherzeele, Potassium titanyl phosphate: properties and new applications, J. Opt. Soc. Am. B, 1989, 6, 622–633 CrossRef CAS
.
- M. Mutailipu, F. Li, C. Jin, Z. Yang, K. R. Poeppelmeier and S. Pan, Strong Nonlinearity Induced by Coaxial Alignment of Polar Chain and Dense [BO3] Units in CaZn2(BO3)2, Angew. Chem., Int. Ed., 2022, 134, e202202096 CrossRef
.
- M. Mutailipu, M. Zhang, H. Wu, Z. Yang, Y. Shen, J. Sun and S. Pan, Ba3Mg3(BO3)3F3 polymorphs with reversible phase transition and high performances as ultraviolet nonlinear optical materials, Nat. Commun., 2018, 9, 1–10 CrossRef CAS
.
- C. Chen, G. Wang, X. Wang and Z. Xu, Deep-UV nonlinear optical crystal KBe2BO3F2—discovery, growth, optical properties and applications, Appl. Phys. B: Lasers Opt., 2009, 97, 9–25 CrossRef CAS
.
- C. Chen, Y. Wu, A. Jiang, B. Wu, G. You, R. Li and S. Lin, New nonlinear-optical crystal: LiB3O5, J. Opt. Soc. Am. B, 1989, 6, 616–621 CrossRef CAS
.
- G. Zou, N. Ye, L. Huang and X. Lin, Alkaline-alkaline earth fluoride carbonate crystals ABCO3F (A = K, Rb, Cs; B = Ca, Sr, Ba) as nonlinear optical materials, J. Am. Chem. Soc., 2011, 133, 20001–20007 CrossRef CAS
.
- G. Zou, C. Lin, H. G. Kim, H. Jo and K. M. Ok, Rb2Na(NO3)3: A congruently melting UV-NLO crystal with a very strong second-harmonic generation response, Crystals, 2016, 6, 42 CrossRef
.
- L. Xiong, L.-M. Wu and L. Chen, A General Principle for DUV NLO Materials: π-Conjugated Confinement Enlarges Band Gap, Angew. Chem., Int. Ed., 2021, 60, 25063–25067 CrossRef CAS
.
- C. Chen, Z. Lin and Z. Wang, Deep-UV Nonlinear Optical Crystal KBe2BO3F2-Discovery, Growth, Optical Properties and Applications, Appl. Phys. B: Lasers Opt., 2005, 80, 1 CrossRef CAS
.
- L. Kang, F. Liang, P. Gong, Z. Lin, F. Liu and B. Huang, Two Novel Deep-Ultraviolet Nonlinear Optical Crystals with Shorter Phase-Matching Second Harmonic Generation than KBe2BO3F2: A First-Principles Prediction, Phys. Status Solidi RRL, 2018, 1800276 CrossRef
.
- M.-R. Li, W. Liu, M.-H. Ge, H.-H. Chen, X.-X. Yang and J.-T. Zhao, NH4[BPO4F]: A novel open-framework ammonium fluorinated borophosphate with a zeolite-like structure related to gismondine topology, Chem. Commun., 2004, 1272–1273 RSC
.
- X. Shi, A. Tudi, M. Cheng, F. Zhang, Z. Yang, S. Han and S. Pan, Noncentrosymmetric Rare-Earth Borate Fluoride La2B5O9F3: A New Ultraviolet Nonlinear Optical Crystal with Enhanced Linear and Nonlinear Performance, ACS Appl. Mater. Interfaces, 2022, 14, 18704–18712 CrossRef CAS
.
- H. Wu, B. Zhang, H. Yu, Z. Hu, J. Wang, Y. Wu and P. S. Halasyamani, Designing Silicates as Deep-UV Nonlinear Optical (NLO) Materials using Edge-Sharing Tetrahedra, Angew. Chem., Int. Ed., 2020, 59, 8922–8926 CrossRef CAS PubMed
.
- X. Dong, L. Huang, H. Zeng, Z. Lin, K. M. Ok and G. Zou, High-Performance Sulfate Optical Materials Exhibiting Giant Second Harmonic Generation and Large Birefringence, Angew. Chem., Int. Ed., 2022, 134, e202116790 Search PubMed
.
- C. Wu, X. Jiang, Y. Hu, C. Jiang, T. Wu, Z. Lin, Z. Huang, M. G. Humphrey and C. Zhang, A Lanthanum Ammonium Sulfate Double Salt with a Strong SHG Response and Wide Deep-UV Transparency, Angew. Chem., Int. Ed., 2022, 61, e202115855 CAS
.
- Y. Li, S. Zhao, P. Shan, X. Li, Q. Ding, S. Liu, Z. Wu, S. Wang, L. Li and J. Luo, Li8NaRb3(SO4)6·2H2O as a new sulfate deep-ultraviolet nonlinear optical material, J. Mater. Chem. C, 2018, 6, 12240–12244 RSC
.
- Q. Ding, X. Liu, S. Zhao, Y. Wang, Y. Li, L. Li, S. Liu, Z. Lin, M. Hong and J. Luo, Designing a deep-UV nonlinear optical fluorooxosilicophosphate, J. Am. Chem. Soc., 2020, 142, 6472–6476 CrossRef CAS PubMed
.
- Y. C. Yang, X. Liu, J. Lu, L. M. Wu and L. Chen, [Ag(NH3)2]2SO4: A strategy for the coordination of cationic moieties to design nonlinear optical materials, Angew. Chem., Int. Ed., 2021, 60, 21216–21220 CrossRef CAS PubMed
.
- Y. Shen, X. Xue, W. Tu, Z. Liu, R. Yan, H. Zhang and J. Jia, Synthesis, crystal structure, and characterization of a noncentrosymmetric sulfate Cs2Ca2(SO4)3, Eur. J. Inorg. Chem., 2020, 854–858 CrossRef CAS
.
-
R. Saint, 4.05, Siemens Energy and Automation Inc; Madison, WI, 53719, 1996.
- R. H. Blessing, An empirical correction for absorption anisotropy, Acta Crystallogr., Sect. A: Found. Crystallogr., 1995, 51, 33–38 CrossRef PubMed
.
-
G. Sheldrick, SHELXS-2013/1, program for the solution of crystal structures, Germany: University of Göttingen, 2013 Search PubMed
.
- G. M. Sheldrick, Crystal structure refinement with SHELXL, Acta Crystallogr., Sect. C: Struct., Chem., 2015, 71, 3–8 Search PubMed
.
- L. J. Farrugia, WinGX and ORTEP for Windows: an update, J. Appl. Crystallogr., 2012, 45, 849–854 CrossRef CAS
.
- P. Giannozzi, S. Baroni, N. Bonini, M. Calandra, R. Car, C. Cavazzoni, D. Ceresoli, G. L. Chiarotti, M. Cococcioni and I. Dabo, QUANTUM ESPRESSO: a modular and open-source software project for quantum simulations of materials, J. Phys.: Condens. Matter, 2009, 21, 395502 CrossRef PubMed
.
- J. P. Perdew, K. Burke and M. Ernzerhof, Generalized Gradient Approximation Made Simple, Phys. Rev. Lett., 1996, 77, 3865 CrossRef CAS PubMed
.
- D. Vanderbilt, Soft self-consistent pseudopotentials in a generalized eigenvalue formalism, Phys. Rev. B: Condens. Matter Mater. Phys., 1990, 41, 7892 CrossRef PubMed
.
- S. K. Kurtz and T. T. Perry, A powder technique for evaluation of nonlinear optical materials, J. Appl. Phys., 1968, 39, 3798 CrossRef CAS
.
- X. Liu, X. Chen, Y. Yu, W. Xie, Y. Zhao, S. Luo, G. Mei and J. Lin, Broad-band excited and tunable luminescence of CaTbAl3O7: Re3+ (Re3+= Ce3+ and/or Eu3+) nanocrystalline phosphors for near-UV wleds, Inorg. Chem., 2020, 59, 12348–12361 CrossRef CAS
.
- S. Sirotinkin, V. Efremov, L. Kovba and A. Pokrovskij, Crystal structure of double lithium and europium sulfate, Kristallografiya, 1977, 22, 966–970 CAS
.
- P. Dorenbos, Lanthanide charge transfer energies and related luminescence, charge carrier trapping, and redox phenomena, J. Alloys Compd., 2009, 488, 568–573 CrossRef CAS
.
- S.-J. Oh, H.-G. Kim, H. Jo, T. G. Lim, J. S. Yoo and K. M. Ok, Photoconversion Mechanisms and the Origin of Second-Harmonic Generation in Metal Iodates with Wide Transparency, NaLn(IO3)4 (Ln = La, Ce, Sm, and Eu) and NaLa(IO3)4:Ln3+ (Ln = Sm and Eu), Inorg. Chem., 2017, 56, 6973–6981 CrossRef CAS PubMed
.
- M. Machida, K. Kawamura, K. Ito and K. Ikeue, Large-Capacity Oxygen Storage by Lanthanide Oxysulfate/Oxysulfide Systems, Chem. Mater., 2005, 17, 1487–1492 CrossRef CAS
.
- J. P. Perdew, Density functional theory and the band gap problem, Int. J. Quantum Chem., 1985, 28, 497–523 CrossRef
.
- K. Kim, M. H. Lee and K. M. Ok, Structural Origin of Very Large Second-Harmonic Generation of a Layered Perovskite, Na0.5Bi2.5Nb2O9, Chem. Mater., 2021, 33, 6564–6571 CrossRef CAS
.
Footnote |
† Electronic supplementary information (ESI) available: Detailed crystallographic data, experimental and calculated PXRD data, SEM-EDX data, IR spectra, TGA diagrams, band structures, and DOS diagrams. CCDC 2209391–2209393. For ESI and crystallographic data in CIF or other electronic format see DOI: https://doi.org/10.1039/d2qm00983h |
|
This journal is © the Partner Organisations 2023 |
Click here to see how this site uses Cookies. View our privacy policy here.