DOI:
10.1039/D3QI00409K
(Research Article)
Inorg. Chem. Front., 2023,
10, 2618-2625
Carboxylate engineering for manipulating the optical and assembly properties of copper clusters†
Received
5th March 2023
, Accepted 22nd March 2023
First published on 28th March 2023
Abstract
Surface ligands are critical in the construction and stabilization of atomically precise metal nanoclusters (NCs) with diverse structures, and ligand engineering remains one of the most effective ways to tailor their properties. In this work, we report the synthesis, structure and surface engineering of novel copper nanoclusters co-protected by carboxylic and thioate ligands. The two clusters share the same formula Cu14(RCOO)6(AdmS)8 (RCOOH is benzoic acid or 2-[(2,6-dichlorophenyl) amino] benzeneacetic acid, and AdmSH is 1-adamantanethiol) and a similar molecular structure. What is surprising to us, however, is that the optical properties, stability and assembly structures of the two clusters are significantly different, thus strongly indicating the potential of engineering carboxylates for manipulating the physicochemical properties of atomically precise copper NCs. This work not only provides model clusters stabilized by carboxylic ligands for further study of the structure–property relationship, but also outlines the big picture of carboxylic-stabilized metal nanoclusters that will flourish in the near future.
Introduction
Owing to ultra-small sizes, well-defined structures, and unique electronic structures, atomically precise metal nanoclusters (NCs) have attracted widespread interest across the fields of bio-imaging, chemical sensing, catalysis, and nanomedicine.1–7 The clusters with precise structures are model platforms to explore the relationship between structures and properties at the atomic level, and thus direct the synthesis of functional nanomaterials for target applications in a reasonable way.8–16 In particular, the properties of metal NCs heavily rely on their compositions, charges and structures, in which the metal–ligand interface is crucial. It has been well documented that electronic structures, local coordination environments, and physicochemical properties are susceptible to delicate changes of ligand–metal interfacial structures.17–25 Therefore, it is of significance to deepen the understanding of the correlation between interfacial structures and physicochemical properties of metal NCs.19,22,23,26 In the past decades, great effort was made in engineering ligands for the construction and stabilization of atomically precise metal NCs with diverse interfacial structures, which are represented by metal-phosphine, metal-thiol, metal-alkynyl, metal-halide, and metal-N-heterocyclic carbene.6,11,15,19–21,23,24,27–45
Close examination of the literature reveals that although significant advancements have been made in illustrating the interfaces of metal NCs by introducing diverse ligands, much less explored in the community are carboxylates. Due to their great affordability, availability, and variety, carboxylate ligands have evolved to be some of the most common compounds that find wide application in the fields of organometallics, materials, catalysis, etc.18,46–59 Of note, the presence of carboxylate ligands on the surface of a handful of metal NCs is a pioneering implication for tailoring physicochemical properties of metal NCs by engineering carboxylate groups.60–62 Recently, several important studies have clearly demonstrated that carboxylates have emerged as a new class of ligands in the field of NCs. It has even been claimed from the viewpoint of theoretical calculations that carboxylates would enable great tunability of geometric structures, hence making them attractive ligands for endowing NCs with interesting catalytic, photocatalytic, and optical properties.63 Experimentally, the reports by Liu and co-workers for determining the crystal structure of all-carboxylate protected superatomic Ag8 for the first time, and by Wang and co-workers for preparing amino carboxylate-stabilized Ag47 with homochirality have clearly illustrated that carboxylate-stabilized NCs feature interesting optical properties, thus providing us a bright prospect for further functionalizations.64,65
Although carboxylate ligands have been introduced in the protection of metal NCs in previous reports, engineering carboxylate ligands for manipulating the optical and assembly properties of metal NCs such as copper, to the best of our knowledge, has not been claimed. Herein, we report the synthesis and structural determinations of two novel copper clusters co-stabilized by carboxylate and thiol ligands: [Cu14(BEN)6(AdmS)8] (Cu14-1) and [Cu14(diclofenac)6(AdmS)8] (Cu14-2) (BEN is benzoic acid, diclofenac is 2-[(2,6-dichlorophenyl) amino] benzeneacetic acid, and AdmSH is 1-adamantanethiol). Although the two Cu14 clusters share similar formulas, charges and structures, the use of different carboxylate ligands induces significant changes in their optical, stability and assembly properties, which therefore indicates the bright future of engineering carboxylate ligands for regulating the properties of NCs.
Results and discussion
The two Cu14 clusters were prepared by the hydrothermal reduction method reported by Melosh et al.66 Cu(RCOO)2 salts were reduced by ethylene glycol under thermal treatment in the presence of thiols to afford raw products (see the Experimental section for details). Bright yellow crystals suitable for single crystal X-ray diffraction analysis were obtained by vapor diffusion of ether into the cluster solution (Fig. S1 and S2†).
Structural analysis revealed that Cu14-1 crystallized in a triclinic system with a space group of P
, and Cu14-2 in a monoclinic unit cell in the P21c space group (Tables S1 and S2†). Due to the structural similarity of these two nanoclusters, we here present only the detailed structure of Cu14-1. The asymmetric unit in the structure of Cu14-1 contains 156 crystallographically independent sites, i.e., fourteen Cu, eight AdmSH ligands and six BEN ligands. In addition, no counterion was observed in the lattice, which indicates that it is a neutral molecule. Thus, the empirical formula of Cu14-1 was proposed to be [Cu14(BEN)6(Adms)8] (Fig. S3 and S4†). On the basis of its formula, all the 14 copper atoms are in the +1 oxidation state, which suggests the effective reduction of Cu2+ by ethylene glycol under heating conditions.
The metal skeleton of Cu14-1 was simultaneously protected by different types of donors of hard Lewis groups of O and the soft Lewis ones of S (Fig. 1a). The structure features a Cu12S8 core which could be viewed as two S atoms tetrahedrally embedded in the Cu12 framework (Fig. 1b). Considering the monovalent valence state of copper ions, the metal framework of Cu14-1 has an excessively positive charge. In order to make the structure stable, the core of Cu14-1 was further passivated by six BEN ligands that are less sterically demanding to form isolated neutral clusters. The metal framework of the cluster can be regarded as a malformed prismatic structure consisting of four prongs, and the thiol ligands act as bridging units to connect the four prongs to each other (Fig. 1c). Besides, the two Cu atoms at the end positions of each prong are capped by BEN ligands with Cu–O bond lengths of 1.863–2.044 Å (Fig. 1d), which is consistent with the literature reports.67
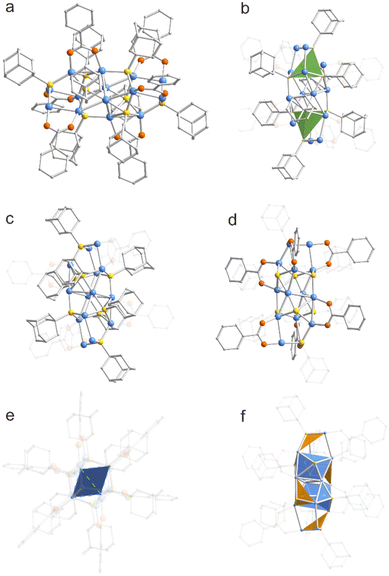 |
| Fig. 1 (a) The total structure of the [Cu14(BEN)6(AdmS)8] cluster. (b) The tetrahedral S4 unit in the core. (c and d) The binding pattern between the Cu atoms and ligands in the Cu14 metal core (one of them is highlighted). (e) Cu4 plane at the center of the cluster. (f) Triangular conical Cu3S (orange) and tetragonal conical Cu4S (blue) fragment units. Color codes for atoms: blue spheres, Cu; orange spheres, O; yellow spheres, S; grey spheres, C. All hydrogen atoms are omitted for clarity. | |
Compared with previously reported copper clusters capped by thiolate ligands, the introduction of BEN ligands not only makes the entire structure of Cu14-1 distinct, but also brings great significance for the construction of new connection patterns within the clusters. For example, the structure of Cu14-1 is highly different from that of Cu14(C2B10H10S2)6(CH3CN)8 (C2B10H10S2 is 1,2-dithiolate-o-carborane) which features a cubic Cu14 core.68 Furthermore, in sharp contrast to [NEt4][Cu(AdmS)2] and [NEt4][Cu5(AdmS)6], the copper atoms in the center of Cu14-1 are interconnected to form a planar quadrilateral structure of Cu4 (Fig. 1e).56 Notably, Cu–Cu bonds (green) with 2.94 Å are found in the Cu4 plane (Fig. 2a). The value is slightly larger than the average Cu–Cu bond lengths (Table S3†). The Cu–S–Cu bond lengths are of a wide range as well, with values between 2.138 and 2.647 Å, which is related to the two bridging modes (μ3-S, μ4-S) within the cluster in triangular conical Cu3S (orange) and tetragonal conical Cu4S (blue) fragment units, respectively (Fig. 1f). Furthermore, the Cu–S–Cu bond angles thus are in a relatively large range of 66.99–129.11°.
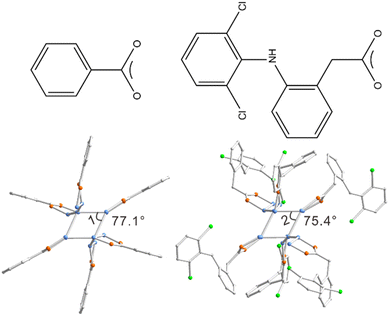 |
| Fig. 2 Structural comparisons of Cu14-1 and Cu14-2 clusters stabilized by different acids. The angles of the Cu4 plane are slightly different due to the use of different acids. Color codes for atoms: blue spheres, Cu; orange spheres, O; yellow spheres, S; grey spheres, C. All hydrogen atoms are omitted for clarity. | |
To confirm the formula determined by X-ray crystallographic analysis, high-resolution electrospray ionization time-of-flight mass spectroscopy (ESI-TOF-MS) of Cu14-1 was then conducted in the positive mode. As shown in Fig. S5,† the ESI-MS of Cu14-1 in the positive mode shows one prominent peak at ∼2470 and a small peak ∼3010 m/z. The two peaks can be assigned to be [Cu12(BEN)3(AdmS)8]+ and [Cu15(BEN)6(AdmS)8]+, respectively. The excellent match of the experimental and simulated isotope patterns verified the composition proposed by single crystal diffraction analysis (Fig. S5,† inset).
With Cu14-1 in hand, we thus in the following section wonder whether its surface structure can be regulated by introducing different carboxylic ligands. 2-[(2,6-Dichlorophenyl) amino] benzeneacetic acid (diclofenac) was selected here considering its large steric hindrance and wide use in the area of medicine (Fig. 2). [Cu14(diclofenac)6(AdmS)8] (Cu14-2) can be readily obtained by a similar synthetic approach to that of Cu14-1 by using diclofenac instead of BEN (Fig. S6 and S7†). It is noteworthy that Cu14-2, to the best of our knowledge, represents the first copper cluster stabilized by diclofenac. Structural comparisons reveal that the metal core and coordination mode of Cu14-2 are similar to those of Cu14-1, with the Cu–Cu bond lengths ranging from 2.609 to 2.842 Å, Cu–O from 1.876 to 2.026 Å and Cu–S from 2.149 to 2.573 Å, respectively. Careful comparison reveals that the average Cu–Cu bond lengths in Cu14-2 are slightly shorter than those of Cu14-1 (Table S3†). Notably, the angles of the Cu4 plane are highly different for the two clusters. The angle of quadrilateral in Cu14-1 is 77.1°, while it is only 75.4° for Cu14-2 (Fig. 2).
Despite the small structural deviations induced by using different carboxylic ligands, the physicochemical properties of the two Cu14 clusters are highly different. As shown in Fig. 3 (red trace), the room temperature ultraviolet-visible spectrum (UV-vis) of Cu14-1 manifests a unique electronic structure, with characteristic peaks at ∼235, ∼275 and ∼330 nm, respectively. To our surprise, the UV-Vis spectrum of Cu14-2 is extremely distinct from that of Cu14-1, which displays several prominent absorption bands at around 270, 350 and 500 nm, respectively (Fig. 3, green trace). We note that the heavy dependence of the optical properties on the surface ligands of Cu14 may facilitate their application in optics down to the nanometer scale.
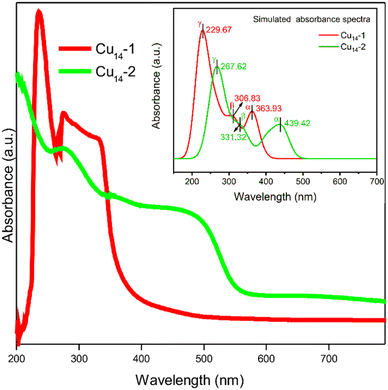 |
| Fig. 3 Experimental UV-Vis spectra of Cu14-1 and Cu14-2 clusters in dichloromethane. The inset shows the simulated absorbance spectra of Cu14-1 and Cu14-2 clusters. | |
To understand why the two clusters exhibit significantly different spectral properties, we have thus performed density functional theory (DFT) calculation to investigate their electronic structures. The experimental structures of the clusters were directly employed as the moieties for calculation (see technical details in the Experimental section). The simulated spectra of the two clusters, as shown in Fig. 3 (inset), exhibit similar profiles to the experimental ones. The calculated UV-Vis spectrum of Cu14-1 mainly displays three absorption band wavelengths at 364, 307 and 230 nm, respectively, which are attributed to the transition of α (HOMO to LUMO transition), β (HOMO−2 to LUMO) and γ (HOMO−5 to LUMO+2), respectively (Fig. 4a). As a comparison, the simulated UV spectrum of Cu14-2 exhibits an electronic transition from HOMO−2 to LUMO+2 with the minimum wavelength at 268 nm (Fig. 4b). It suggests that the energy levels for the electronic transitions of the two clusters are different, therefore rationalizing their distinct optical properties.
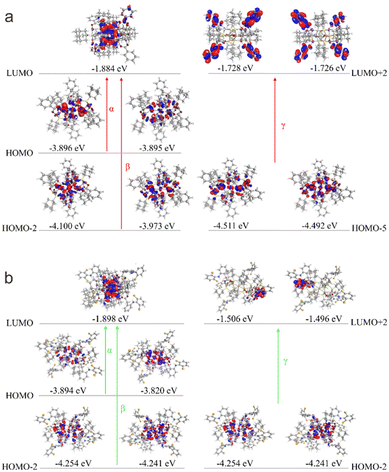 |
| Fig. 4 Orbitals involved in the α, β and γ transitions of Cu14-1 (a) and Cu14-2 (b). | |
Not only absorbance features of the two clusters have been tailored significantly by using different types of carboxylates, but also their stability and self-assembly modes. As suggested by the time-dependent UV-Vis spectra (Fig. S8†), while Cu14-1 exhibits high stability in the solution form, Cu14-2 is prone to decompose under similar conditions. Moreover, the two clusters are in practically different space groups (P
for Cu14-1 and P21c for Cu14-2). Shown in Fig. 5 is the comparison of the assembly structures of the two clusters. The packing structure of Cu14-1 is loosely arranged in the linear mode along the c-axis (Fig. 5a), while that of Cu14-2 is in a twisted style by closely touching with each other (Fig. 5b). The distinction observed above strongly indicates the efficiency of employing different carboxylates as protective shells for regulating assembly chemistry of metal clusters.
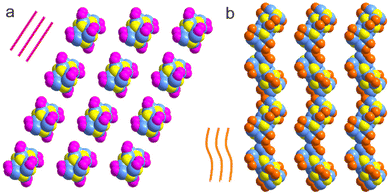 |
| Fig. 5 Assembly structures of Cu14-1 (a) and Cu14-2 (b) clusters along the c-axis. Color codes for atoms: blue spheres, Cu; magenta and orange spheres, O; yellow spheres, S. All other atoms are omitted for clarity. | |
Conclusions
In summary, we report the synthesis and structural determination of two novel copper nanoclusters stabilized by carboxylate ligands, which motivates the wide exploration of carboxylates as the next generation of surface ligands for the protection of metal nanoclusters. We have also introduced, for the first time, diclofenac as a ligand for the stabilization of copper clusters, implying their potential applications in medicine. Moreover, it has been demonstrated that the metal skeleton, surface structure, optical properties, stability, and assembly structures of carboxylic-protected metal nanoclusters can be tuned by employing different carboxylic groups. These Cu14 clusters reported herein not only have application prospects in the fields of optics and catalysis, but also can be regarded as a model system to study the fundamental issue of structure–activity relationship. More studies on preparing carboxylate-functionalized metal nanoclusters are ongoing in our laboratories to deepen the understanding of metal–carboxylic interfaces and the relationship between their structures and performances.
Experimental
Materials
Copper sulfate (CuSO4, 99%), 2-[(2,6-dichlorophenyl) amino] benzeneacetic acid (diclofenac, 98%), benzoic acid (C6H5COOH, 98%) and 1-adamantanethiol (AdmSH, 98%) were purchased from Bidepharm (Shanghai, China). Dichloromethane (CH2Cl2, A.R.), ethylene glycol ((CH2OH)2, A.R.), toluene (C7H8, A.R.) and ether (C4H10O, A.R.) were purchased from Sinopharm Chemical Reagent Co. Ltd (Shanghai, China). Water used in all experiments was ultrapure. All other reagents were used as received without further purification.
Synthesis of Cu(C6H5COO)2 and Cu(diclofenac)2
Cu(C6H5COO)2 was synthesized by dissolving 28.8 mg of C6H5COONa and 16 mg of CuSO4 in 10 mL of deionized water. After stirring for 2 h at room temperature, the reaction mixture was filtered, and the green precipitate was washed with water and dried in air. The resulting copper salts were obtained in good yield. Cu(diclofenac)2 was prepared using a similar procedure except for the use of 32 mg of Na(diclofenac) and 16 mg of CuSO4 as precursors.
Synthesis of Cu14
Cu14-1 was prepared by a modified procedure of copper-based metal–organic chalcogenides. Typically, Cu(C6H5COO)2 and AdmSH at a molar ratio of 1
:
1 were dissolved in a mixed solvent of ethylene glycol and toluene. The mixture was heated at 80 °C over a period of 48 h, resulting in a yellow solution. The solution was then carefully filtered and dried up. To the solid, dichloromethane was added, affording a yellow solution. Yellow block crystals were obtained by vapor diffusion of ether into the solution. Cu14-2 was prepared by a similar procedure of Cu14-1, except for the use of Cu(diclofenac)2 instead of Cu(C6H5COO)2.
Characterization studies
UV-Vis spectra.
UV-Vis spectra were collected using a Carry-5000 Spectrophotometer using a quartz cuvette of 1 cm path length. The scanning speed was 1000 nm min−1. The spectra were recorded in diluted solutions of dichloromethane and the signal of the blank solvent was subtracted.
ESI-MS.
Electrospray ionization mass spectra (ESI-MS) were recorded using an Agilent 6224 time-of-flight mass spectrometer in the positive mode. The samples dissolved in dichloromethane were filtered before the measurements. Then the sample was directly infused at a flow rate of 1.2 mL h−1 using a syringe pump. Typical parameters used for the measurements were as follows: capillary voltage: 4.0 kV; drying gas temp.: 150 °C; drying gas flow: 4 L min−1; nebulizer pressure: 20 psi.
Crystallography.
X-ray single-crystal analysis: the diffraction data of the single crystals of compounds Cu14-1 and Cu14-2 were collected using a Rigaku Oxford Diffraction system X-ray single-crystal diffractometer using Cu Kα (λ = 1.54184 Å) at 293 K. The data were processed using CrysAlisPro. The structure was solved and refined using full-matrix least-squares based on F2 using ShelXT,69 ShelXL70 in Olex2,71 and Shelxle.72 The thermal ellipsoids of the ORTEP diagram were generated at 50% probability. Detailed crystal data and structural refinements for the two compounds are given in Tables S1 and S2.† CCDC 2216708 and 2216705† contain the supplementary crystallographic data for this paper.
Computational details
As for the optical-absorption spectrum and orbital information, the density functional theory (DFT) calculations were implemented in the quantum chemistry program Turbomole V4.2.73 Geometry optimization of the two Cu14 clusters was carried out by DFT calculations with the TPSS (Tao, Perdew, Staroverov, and Scuseria) functional for electron exchange and correlation and def2-SV(P) for orbital and auxiliary basis sets.74 Of note, the def2-SV(P) basis sets were used for S, N, O, Cl, C and H, while effective core potentials which include scalar relativistic corrections were considered for Cu.75,76 Then, the time-dependent DFT computation of the optical absorption spectrum was performed at the PBE level with the def2-SV(P) basis sets. All transitions together with their oscillator strengths were then convoluted with a Gaussian line shape of 0.5 eV broadening to generate the optical-absorption spectrum.
Author contributions
The manuscript was written through contributions of all authors. H. S., Q. T. and Chaolumen supervised the project. J. S., F. S., J. T. and X. T. prepared the cluster samples and conducted the characterization studies. Q. W., R. H., A. H., Sachurilatu and X. S. were responsible for analyzing the data. All authors contributed to composing the manuscript.
Conflicts of interest
There are no conflicts to declare.
Acknowledgements
We are grateful for the financial support provided by the NSFC (2022161035), the Inner Mongolia Autonomous Region Fund for Natural Science (2021MS02001), and the Education Department of Inner Mongolia Autonomous Region (NJYT22089).
References
- R. Jin, C. Zeng, M. Zhou and Y. Chen, Atomically Precise Colloidal Metal Nanoclusters and Nanoparticles: Fundamentals and Opportunities, Chem. Rev., 2016, 116, 10346 CrossRef CAS PubMed.
- Y. Du, H. Sheng, D. Astruc and M. Zhu, Atomically Precise Noble Metal Nanoclusters as Efficient Catalysts: A Bridge between Structure and Properties, Chem. Rev., 2020, 120, 526 CrossRef CAS PubMed.
- X. Liu and D. Astruc, Atomically precise copper nanoclusters and their applications, Coord. Chem. Rev., 2018, 359, 112 CrossRef CAS.
- A. J. Jordan, G. Lalic and J. P. Sadighi, Coinage Metal Hydrides: Synthesis, Characterization, and Reactivity, Chem. Rev., 2016, 116, 8318 CrossRef CAS PubMed.
- Z. Gan, N. Xia and Z. Wu, Discovery, Mechanism, and Application of Antigalvanic Reaction, Acc. Chem. Res., 2018, 51, 2774 CrossRef CAS PubMed.
- K. Konishi, M. Iwasaki and Y. Shichibu, Phosphine-Ligated Gold Clusters with Core + exo Geometries: Unique Properties and Interactions at the Ligand-Cluster Interface, Acc. Chem. Res., 2018, 51, 3125 CrossRef CAS PubMed.
- S. Sharma, K. K. Chakrahari, J. Saillard and C. W. Liu, Structurally Precise Dichalcogenolate-Protected Copper and Silver Superatomic Nanoclusters and Their Alloys, Acc. Chem. Res., 2018, 51, 2475 CrossRef CAS PubMed.
- R. S. Dhayal, W. E. van Zyl and C. W. Liu, Polyhydrido Copper Clusters: Synthetic Advances, Structural Diversity, and Nanocluster-to-Nanoparticle Conversion, Acc. Chem. Res., 2016, 49, 86 CrossRef CAS PubMed.
- A. Ghosh, O. F. Mohammed and O. M. Bakr, Atomic-Level Doping of Metal Clusters, Acc. Chem. Res., 2018, 51, 3094 CrossRef CAS PubMed.
- R. Jin, Y. Pei and T. Tsukuda, Controlling Nanoparticles with Atomic Precision, Acc. Chem. Res., 2019, 52, 1 CrossRef CAS PubMed.
- Z. Lei, X. K. Wan, S. F. Yuan, Z. J. Guan and Q. M. Wang, Alkynyl Approach toward the Protection of Metal Nanoclusters, Acc. Chem. Res., 2018, 51, 2465 CrossRef CAS PubMed.
- Q. Yao, T. Chen, X. Yuan and J. Xie, Toward Total Synthesis of Thiolate-Protected Metal Nanoclusters, Acc. Chem. Res., 2018, 51, 1338 CrossRef CAS PubMed.
- A. Baghdasaryan and T. Bürgi, Copper nanoclusters: designed synthesis, structural diversity, and multiplatform applications, Nanoscale, 2021, 13, 6283 RSC.
- H. Häkkinen, The gold–sulfur interface at the nanoscale, Nat. Chem., 2012, 4, 443 CrossRef PubMed.
- P. D. Jadzinsky, G. Calero, C. J. Ackerson, D. A. Bushnell and R. D. Kornberg, Structure of a Thiol Monolayer-Protected Gold Nanoparticle at 1.1 Å Resolution, Science, 2007, 318, 430 CrossRef CAS PubMed.
- C. Zeng, Y. Chen, K. Kirschbaum, K. J. Lambright and R. Jin, Emergence of hierarchical structural complexities in nanoparticles and their assembly, Science, 2016, 354, 1580 CrossRef CAS PubMed.
- J. Yan, B. K. Teo and N. F. Zheng, Surface Chemistry of Atomically Precise Coinage-Metal Nanoclusters: From Structural Control to Surface Reactivity and Catalysis, Acc. Chem. Res., 2018, 51, 3084 CrossRef CAS PubMed.
- S. Bera, B. Xue, P. Rehak, G. Jacoby, W. Ji, L. J. W. Shimon, R. Beck, P. Kral, Y. Cao and E. Gazit, Self-Assembly of Aromatic Amino Acid Enantiomers into Supramolecular Materials of High Rigidity, ACS Nano, 2020, 14, 1694 CrossRef CAS PubMed.
- P. N. Gunawardene, J. Martin, J. M. Wong, Z. Ding, J. F. Corrigan and M. S. Workentin, Controlling the Structure, Properties and Surface Reactivity of Clickable Azide-Functionalized Au25(SR)18 Nanocluster Platforms Through Regioisomeric Ligand Modifications, Angew. Chem., Int. Ed., 2022, 61, e202205194 CrossRef CAS PubMed.
- H. Shen, G. Deng, S. Kaappa, T. Tan, Y. Z. Han, S. Malola, S. C. Lin, B. K. Teo, H. Häkkinen and N. F. Zheng, Highly Robust but Surface-Active: An N-Heterocyclic Carbene-Stabilized Au25 Nanocluster, Angew. Chem., Int. Ed., 2019, 58, 17731 CrossRef CAS PubMed.
- H. Shen, Z. Xu, M. S. A. Hazer, Q. Wu, J. Peng, R. Qin, S. Malola, B. K. Teo, H. Häkkinen and N. F. Zheng, Surface Coordination of Multiple Ligands Endows N-Heterocyclic Carbene-Stabilized Gold Nanoclusters with High Robustness and Surface Reactivity, Angew. Chem., Int. Ed., 2021, 60, 3752 CrossRef CAS PubMed.
- X. Du and R. Jin, Atomic-precision engineering of metal nanoclusters, Dalton Trans., 2020, 49, 10701 RSC.
- L. Xu, Q. Li, T. Li, J. Chai, S. Yang and M. Zhu, Construction of a new Au27Cd1(SAdm)14(DPPF)Cl nanocluster by surface engineering and insight into its structure–property correlation, Inorg. Chem. Front., 2021, 8, 4820 RSC.
- C. Dong, R. Huang, C. Chen, J. Chen, S. Nematulloev, X. Guo, A. Ghosh, B. Alamer, M. N. Hedhili, T. T. Isimjan, Y. Han, O. F. Mohammed and O. M. Bakr, [Cu36H10(PET)24(PPh3)6Cl2] Reveals Surface Vacancy Defects in Ligand-Stabilized Metal Nanoclusters, J. Am. Chem. Soc., 2021, 143, 11026 CrossRef CAS PubMed.
- P. Liu, R. Qin, G. Fu and N. F. Zheng, Surface Coordination Chemistry of Metal Nanomaterials, J. Am. Chem. Soc., 2017, 139, 2122 CrossRef CAS PubMed.
- X. Lin, C. Liu, K. Sun, R. Wu, X. Fu and J. Huang, Structural isomer and high-yield of Pt1Ag28 nanocluster via one-pot chemical wet method, Nano Res., 2019, 12, 309 CrossRef CAS.
- C. Sun, N. Mammen, S. Kaappa, P. Yuan, G. Deng, C. Zhao, J. Yan, S. Malola, K. Honkala, H. Häkkinen, B. K. Teo and N. F. Zheng, Atomically Precise, Thiolated Copper–Hydride Nanoclusters as Single-Site Hydrogenation Catalysts for Ketones in Mild Conditions, ACS Nano, 2019, 13, 5975 CrossRef CAS PubMed.
- Y. Cao, V. Fung, Q. Yao, T. Chen, S. Q. Zang, D. Jiang and J. Xie, Control of single-ligand chemistry on thiolated Au25 nanoclusters, Nat. Commun., 2020, 11, 5498 CrossRef CAS PubMed.
- H. Yang, Y. Wang, H. Huang, L. Gell, L. Lehtovaara, S. Malola, H. Hakkinen and N. F. Zheng, All-thiol-stabilized Ag44 and Au12Ag32 nanoparticles with single-crystal structures, Nat. Commun., 2013, 4, 2422 CrossRef PubMed.
- H. Shen and T. Mizuta, An Atomically Precise Alkynyl-Protected PtAg42 Superatom Nanocluster and Its Structural Implications, Chem. – Asian J., 2017, 12, 2904 CrossRef CAS PubMed.
- L. Jin, D. S. Weinberger, M. Melaimi, C. E. Moore, A. L. Rheingold and G. Bertrand, Trinuclear gold clusters supported by cyclic (alkyl)(amino)carbene ligands: mimics for gold heterogeneous catalysts, Angew. Chem., Int. Ed., 2014, 53, 9059 CrossRef CAS PubMed.
- H. Shen, Q. Wu, M. Asre Hazer, X. Tang, Y. Han, R. Qin, C. Ma, S. Malola, B. K. Teo, H. Häkkinen and N. F. Zheng, Regioselective hydrogenation of alkenes over atomically dispersed Pd sites on NHC-stabilized bimetallic nanoclusters, Chem, 2022, 8, 2380 CAS.
- V. K. Kulkarni, B. N. Khiarak, S. Takano, S. Malola, E. L. Albright, T. I. Levchenko, M. D. Aloisio, C. T. Dinh, T. Tsukuda, H. Häkkinen and C. M. Crudden, N-Heterocyclic, Carbene-Stabilized Hydrido Au24 Nanoclusters: Synthesis, Structure, and Electrocatalytic Reduction of CO2, J. Am. Chem. Soc., 2022, 144, 9000 CrossRef CAS PubMed.
- M. R. Narouz, S. Takano, P. A. Lummis, T. I. Levchenko, A. Nazemi, S. Kaappa, S. Malola, G. Yousefalizadeh, L. A. Calhoun, K. G. Stamplecoskie, H. Hakkinen, T. Tsukuda and C. M. Crudden, Robust, Highly Luminescent Au13 Superatoms Protected by N-Heterocyclic Carbenes, J. Am. Chem. Soc., 2019, 141, 14997 CrossRef CAS PubMed.
- H. Shen, Q. Wu, S. Malola, Y. Han, Z. Xu, R. Qin, X. Tang, Y. Chen, B. K. Teo, H. Häkkinen and N. F. Zheng, N-Heterocyclic, Carbene-Stabilized Gold Nanoclusters with Organometallic Motifs for Promoting Catalysis, J. Am. Chem. Soc., 2022, 144, 10844 CrossRef CAS PubMed.
- H. Shen, L. Wang, O. López-Estrada, C. Hu, Q. Wu, D. Cao, S. Malola, B. K. Teo, H. Häkkinen and N. F. Zheng, Copper-hydride nanoclusters with enhanced stability by N-heterocyclic carbenes, Nano Res., 2021, 14, 3303 CrossRef CAS.
- H. Shen, S. Xiang, Z. Xu, C. Liu, X. Li, C. Sun, S. Lin, B. K. Teo and N. F. Zheng, Superatomic Au13 clusters ligated by different N-heterocyclic carbenes and their ligand-dependent catalysis, photoluminescence, and proton sensitivity, Nano Res., 2020, 13, 1908 CrossRef CAS.
- M. R. Narouz, K. M. Osten, P. J. Unsworth, R. W. Y. Man, K. Salorinne, S. Takano, R. Tomihara, S. Kaappa, S. Malola, C. T. Dinh, J. D. Padmos, K. Ayoo, P. J. Garrett, M. Nambo, J. H. Horton, E. H. Sargent, H. Häkkinen, T. Tsukuda and C. M. Crudden, N-heterocyclic carbene-functionalized magic-number gold nanoclusters, Nat. Chem., 2019, 11, 419 CrossRef CAS PubMed.
- H. Shen, Y. Han, Q. Wu, J. Peng, B. K. Teo and N. F. Zheng, Simple and Selective Synthesis of Copper–Containing Metal Nanoclusters Using (PPh3)2CuBH4 as Reducing Agent, Small Methods, 2020, 5, 2000603 CrossRef PubMed.
- C. Liu, S. F. Yuan, S. Wang, Z. J. Guan, D. Jiang and Q. M. Wang, Structural transformation and catalytic hydrogenation activity of amidinate-protected copper hydride clusters, Nat. Commun., 2022, 13, 2082 CrossRef CAS PubMed.
- T. Chiu, J. Liao, F. Gam, Y. Wu, X. Wang, S. Kahlal, J. Saillard and C. W. Liu, Hydride-Containing Eight-Electron Pt/Ag Superatoms: Structure, Bonding, and Multi-NMR Studies, J. Am. Chem. Soc., 2022, 144, 10599 CrossRef CAS PubMed.
- L. G. AbdulHalim, M. S. Bootharaju, Q. Tang, S. Del Gobbo, R. G. AbdulHalim, M. Eddaoudi, D. Jiang and O. M. Bakr, Ag29(BDT)12(TPP)4: A Tetravalent Nanocluster, J. Am. Chem. Soc., 2015, 137, 11970 CrossRef CAS PubMed.
- R. S. Dhayal, J. Liao, S. Kahlal, X. Wang, Y. Liu, M. Chiang, W. E. van Zyl, J. Saillard and C. W. Liu, [Cu32(H)20{S2P(OiPr)2}12]: The Largest Number of Hydrides Recorded in a Molecular Nanocluster by Neutron Diffraction, Chem. – Eur. J., 2015, 21, 8369 CrossRef CAS PubMed.
- Z. Wang, H. Su, L. Zhang, J. Dou, C. H. Tung, D. Sun and L. Zheng, Stepwise Assembly of Ag42 Nanocalices Based on a MoVI-Anchored Thiacalix[4]arene Metalloligand, ACS Nano, 2022, 16, 4500 CrossRef CAS PubMed.
- A. Ghosh, R. W. Huang, B. Alamer, E. Abou-Hamad, M. N. Hedhili, O. F. Mohammed and O. M. Bakr, [Cu61(StBu)26S6Cl6H14]+: A Core–Shell Superatom Nanocluster with a Quasi-J36 Cu19 Core and an “18-Crown-6” Metal-Sulfide-like Stabilizing Belt, ACS Mater. Lett., 2019, 1, 297 CrossRef CAS.
- C. Li, J. Zhao, L. Xie, J. Wu, Q. Ren, Y. Wang and G. Li, Surface-Adsorbed Carboxylate Ligands on Layered Double Hydroxides/Metal-Organic Frameworks Promote the Electrocatalytic Oxygen Evolution Reaction, Angew. Chem., Int. Ed., 2021, 60, 18129 CrossRef CAS PubMed.
- Y. Liu, X. Xi, C. Ye, T. Gong, Z. Yang and Y. Cui, Chiral metal-organic frameworks bearing free carboxylic acids for organocatalyst encapsulation, Angew. Chem., Int. Ed., 2014, 53, 13821 CrossRef CAS PubMed.
- C. A. Salazar, J. J. Gair, K. N. Flesch, I. A. Guzei, J. C. Lewis and S. S. Stahl, Catalytic Behavior of Mono-N-Protected Amino-Acid Ligands in Ligand-Accelerated C-H Activation by Palladium(II), Angew. Chem., Int. Ed., 2020, 59, 10873 CrossRef CAS PubMed.
- O. Karagiaridi, N. A. Vermeulen, R. C. Klet, T. C. Wang, P. Z. Moghadam, S. S. Al-Juaid, J. F. Stoddart, J. T. Hupp and O. K. Farha, Functionalized defects through solvent-assisted linker exchange: synthesis, characterization, and partial postsynthesis elaboration of a metal-organic framework containing free carboxylic acid moieties, Inorg. Chem., 2015, 54, 1785 CrossRef CAS PubMed.
- X. Lv, L. Feng, L. Xie, T. He, W. Wu, K. Wang, G. Si, B. Wang, J. Li and H. Zhou, Linker Desymmetrization: Access to a Series of Rare-Earth Tetracarboxylate Frameworks with Eight-Connected Hexanuclear Nodes, J. Am. Chem. Soc., 2021, 143, 2784 CrossRef CAS PubMed.
- T. Zhou, P. F. Qian, J. Y. Li, Y. B. Zhou, H. C. Li, H. Y. Chen and B. F. Shi, Efficient Synthesis of Sulfur-Stereogenic Sulfoximines via Ru(II)-Catalyzed Enantioselective C-H Functionalization Enabled by Chiral Carboxylic Acid, J. Am. Chem. Soc., 2021, 143, 6810 CrossRef CAS PubMed.
- Z. Xue, K. Liu, Q. Liu, Y. Li, M. Li, C. Su, N. Ogiwara, H. Kobayashi, H. Kitagawa, M. Liu and G. Li, Missing-linker metal-organic frameworks for oxygen evolution reaction, Nat. Commun., 2019, 10, 5048 CrossRef PubMed.
- J. Zhang, L. D. Ellis, B. Wang, M. J. Dzara, C. Sievers, S. Pylypenko, E. Nikolla and J. W. Medlin, Control of interfacial acid–metal catalysis with organic monolayers, Nat. Catal., 2018, 1, 148 CrossRef CAS.
- R. E. Plata, D. E. Hill, B. E. Haines, D. G. Musaev, L. Chu, D. P. Hickey, M. S. Sigman, J. Q. Yu and D. G. Blackmond, A Role for Pd(IV) in Catalytic Enantioselective C-H Functionalization with Monoprotected Amino Acid Ligands under Mild Conditions, J. Am. Chem. Soc., 2017, 139, 9238 CrossRef CAS PubMed.
- P. Su, Z. Zhu, Q. Fan, J. Cao, Y. Wang, X. Yang, B. Cheng, W. Liu and Y. Tang, Surface ligand coordination induced self-assembly of a nanohybrid for efficient photodynamic therapy and imaging, Inorg. Chem. Front., 2018, 5, 2620 RSC.
- F. Kiyoshi, I. Sadako, K. Nobumasa and M. Yoshihiko, Preparation, Spectroscopic Characterization, and Molecular Structure of Copper(I) Aliphatic Thiolate Complexes, Inorg. Chem., 1998, 37, 168 CrossRef.
- J. Wang, H. Liu, Z. Tong and C. Ha, Fluorescent/luminescent detection of natural amino acids by organometallic systems, Coord. Chem. Rev., 2015, 303, 139 CrossRef CAS.
- E. Shin, J. Yoo, G. Yoo, Y. Kim and Y. S. Kim, Eco-friendly cross-linked polymeric dielectric material based on natural tannic acid, Chem. Eng. J., 2019, 358, 170 CrossRef CAS.
- X. Zhao, E. T. Nguyen, A. N. Hong, P. Feng and X. Bu, Chiral Isocamphoric Acid: Founding a Large Family of Homochiral Porous Materials, Angew. Chem., Int. Ed., 2018, 57, 7101 CrossRef CAS PubMed.
- B. Han, Z. Liu, L. Feng, Z. Wang, R. K. Gupta, C. M. Aikens, C. H. Tung and D. Sun, Polymorphism in Atomically Precise Cu23 Nanocluster Incorporating Tetrahedral [Cu4]0 Kernel, J. Am. Chem. Soc., 2020, 142, 5834 CrossRef CAS PubMed.
- A. W. Cook, Z. R. Jones, G. Wu, S. L. Scott and T. W. Hayton, An Organometallic Cu20 Nanocluster: Synthesis, Characterization, Immobilization on Silica, and “Click” Chemistry, J. Am. Chem. Soc., 2018, 140, 394 CrossRef CAS PubMed.
- X. M. Luo, C. H. Gong, X. Y. Dong, L. Zhang and S. Q. Zang, Evolution of all-carboxylate-protected superatomic Ag clusters confined in Ti-organic cages, Nano Res., 2021, 14, 2309 CrossRef CAS.
- T. Liu and D. Jiang, Understanding the interaction between carboxylates and coinage metals from first principles, J. Chem. Phys., 2021, 155, 034301 CrossRef CAS PubMed.
- K. G. Liu, X. M. Gao, T. Liu, M. L. Hu and D. E. Jiang, All-Carboxylate-Protected Superatomic Silver Nanocluster with an Unprecedented Rhombohedral Ag8 Core, J. Am. Chem. Soc., 2020, 142, 16905 CrossRef CAS PubMed.
- W. D. Liu, J. Q. Wang, S. F. Yuan, X. Chen and Q. M. Wang, Chiral Superatomic Nanoclusters Ag47 Induced by the Ligation of Amino Acids, Angew. Chem., Int. Ed., 2021, 60, 11430 CrossRef CAS PubMed.
- H. Yan, J. N. Hohman, F. H. Li, C. Jia, D. Solis-Ibarra, B. Wu, J. E. Dahl, R. M. Carlson, B. A. Tkachenko, A. A. Fokin, P. R. Schreiner, A. Vailionis, T. R. Kim, T. P. Devereaux, Z. X. Shen and N. A. Melosh, Hybrid metal-organic chalcogenide nanowires with electrically conductive inorganic core through diamondoid-directed assembly, Nat. Mater., 2017, 16, 349 CrossRef CAS PubMed.
- L. Asgharnejad, A. Abbasi, M. Najafi and J. Janczak, One-, two- and three-dimensional coordination polymers based on copper paddle-wheel SBUs as selective catalysts for benzyl alcohol oxidation, J. Solid State Chem., 2019, 277, 187 CrossRef CAS.
- Y. L. Li, J. Wang, P. Luo, X. H. Ma, X. Y. Dong, Z. Y. Wang, C. X. Du, S. Q. Zang and T. C. W. Mak, Cu14 Cluster with Partial Cu(0) Character: Difference in Electronic Structure from Isostructural Silver Analog, Adv. Sci., 2019, 6, 1900833 CrossRef CAS PubMed.
- G. M. Sheldrick, SHELXT-integrated space-group and crystal-structure determination, Acta Crystallogr., Sect. A: Found. Adv., 2015, 71, 3 CrossRef PubMed.
- G. M. Sheldrick, A short history of SHELX, Acta Crystallogr., Sect. A: Found. Crystallogr., 2008, 64, 112 CrossRef CAS PubMed.
- O. V. Dolomanov, L. J. Bourhis, R. J. Gildea, J. A. K. Howard and H. Puschmann, OLEX2: a complete structure solution, refinement and analysis program, J. Appl. Crystallogr., 2009, 42, 339 CrossRef CAS.
- C. B. Hubschle, G. M. Sheldrick and B. Dittrich, ShelXle: a Qt graphical user interface for SHELXL, J. Appl. Crystallogr., 2011, 44, 1281 CrossRef PubMed.
- R. Ahlrichs, M. Bär, M. Häser, H. Horn and C. Kölmel, Electronic structure calculations on workstation computers: The program system turbomole, Chem. Phys. Lett., 1989, 162, 165 CrossRef CAS.
- J. Tao, J. P. Perdew, V. N. Staroverov and G. E. Scuseria, Climbing the Density Functional Ladder: Nonempirical Meta–Generalized Gradient Approximation Designed for Molecules and Solids, Phys. Rev. Lett., 2003, 91, 146401 CrossRef PubMed.
- D. Andrae, U. Häußermann, M. Dolg, H. Stoll and H. Preuß, Energy-adjustedab initio pseudopotentials for the second and third row transition elements, Theor. Chim. Acta, 1990, 77, 123 CrossRef CAS.
- D. Andrae, U. Häußermann, M. Dolg, H. Stoll and H. Preuß, Energy-adjustedab initio pseudopotentials for the second and third row transition elements: Molecular test for M2 (M=Ag, Au) and MH (M=Ru, Os), Theor. Chim. Acta, 1991, 78, 247 CrossRef CAS.
Footnotes |
† Electronic supplementary information (ESI) available. CCDC 2216708 and 2216705. For ESI and crystallographic data in CIF or other electronic format see DOI: https://doi.org/10.1039/d3qi00409k |
‡ These authors contributed equally to this work. |
|
This journal is © the Partner Organisations 2023 |
Click here to see how this site uses Cookies. View our privacy policy here.