DOI:
10.1039/D2QI02366K
(Research Article)
Inorg. Chem. Front., 2023,
10, 1279-1285
Direct CO2 photoreduction from flue gas by synergistic catalysis of a nickel metal–organic framework and a ruthenium polypyridyl complex†
Received
8th November 2022
, Accepted 28th December 2022
First published on 30th December 2022
Abstract
Direct photoreduction of CO2 from flue gas is an energy-saving avenue to realize the carbon-neutral cycle but it is still in its infancy. Herein, we constructed a new anionic metal–organic framework based on trinuclear Ni clusters and thiophenecarboxylic acid for CO2 photoreduction of exhaust gas from a power plant. Under visible-light irradiation, the yield of CO was 17.4–26.3 mmol g−1 in diluted CO2 with a concentration of 5–20%. The apparent quantum yield (A.Q.Y.) under a 10% CO2 atmosphere was determined to be 2.1%, which ranks among the highest values of the reported photocatalysts under similar conditions. Importantly, in real flue gas containing 10% CO2, a selectivity of 90.4% was achieved and CO generation reached 18.2 mmol g−1. In situ transient photovoltage (TPV) and density functional theory (DFT) calculations showed that the formation of a CO2 bridged photocatalytic interface between Ni-MOF1 and a Ru complex ([Ru(bpy)3]Cl2/[Ru(bpy)2]Cl2) was a crucial factor for the efficient CO2-to-CO conversion in diluted CO2.
1. Introduction
With the gradual increase in the consumption of fossil fuels, the energy crisis and global warming resulting from heavy emission of CO2 are thorny issues facing human society.1–4 Reduction of CO2 into usable carbon-based fuel using solar light has been considered a promising avenue to solve these problems.5–9 Tremendous efforts have been made in this field and high reaction activity has been achieved but mainly focusing on the utilization of pure CO2.10–17 The purification and condensation of low concentration CO2
20 utilizing dropwise condensation or supersonic separation technologies21 is an energy-intensive and complicated process;22–24 however, the direct usage of CO2 from the exhaust gas of heavy industries, which contains 3–20% CO2,18,19 as the carbon source is an energy-saving, low-cost and sustainable way. However, direct photoreduction of CO2 from real flue gas is still in its initial stages.
Metal–organic frameworks (MOFs) have attracted tremendous attention in low-concentration CO2 photoreduction25–29 due to their advantages of high-density metal nodes and excellent CO2 adsorption capability.30–32 Besides, their well-defined crystalline structures can assist in understanding the structure–activity relationship.33,34 For example, Han et al.35 have demonstrated CO2 reduction activity of metal–organic monolayers (Ni MOLs) under 10% CO2 using [Ru(bpy)3]Cl2 as a photosensitizer. Similarly, Wang and co-workers36 have reported that Co-based MAF-X27-OH can convert CO2 into CO with [Ru(bpy)3]Cl2 as a photosensitizer and the yield from 10% CO2 is comparable to that from pure CO2. Although remarkable progress has been achieved towards the practical application of MOFs,37,38 still the catalytic efficiency at low CO2 concentration needs to be further improved. Since [Ru(bpy)3]Cl2 is a commonly used reagent in MOF systems, the development of efficient interface interaction between a MOF, Ru complex, and CO2 may be significant to enhance the catalytic efficiency, particularly in low-concentration CO2 reduction.39 Until now, there has been a lack of profound understanding of the MOF, Ru complex, and CO2 catalytic interface kinetics and it is still a big challenge to explore such systems.
Herein, a new MOF (Ni-MOF1) with an anionic framework based on thiophenecarboxylic acid and a trinuclear Ni cluster is synthesized for direct CO2 photoreduction from the flue gas of a power plant. The anionic framework could help to facilitate the collaboration between the MOF and [Ru(bpy)3]2+/[Ru(bpy)2]2+. Under visible-light irradiation, the yield of CO is 17.4–26.3 mmol g−1 in diluted CO2 with a concentration of 5–20%. And the apparent quantum yield (A.Q.Y.) is up to 2.1% which is better than that of most reported MOFs under similar conditions.11,18 Meanwhile, a selectivity of 90.4% is achieved and the production of CO is up to 18.2 mmol g−1 on Ni-MOF1 in flue gas containing 10% CO2. Mechanistic studies based on DFT calculations and TPV experiments reveal that CO2 bridged the photocatalytic interface between Ni-MOF1 and the Ru complex in the reduction process, which may account for the relatively high catalytic activity at low concentrations of CO2.
2. Experimental methods
2.1. Preparation of (C2H6NH2)+[Ni3(μ3-OH)(H2O)3(DMTDC)3](C2H5OH)(DMA)2 (Ni-MOF1)
Ni(NO3)2·6H2O (0.058 g, 0.2 mmol) was added to a mixture of DMA (8 mL) and absolute ethanol (2 mL) containing H2DMTDC (Shanghai Tensus Bio-tech Co., Ltd) (0.05 g, 0.02 mmol) and KOH (0.0056 g, 0.1 mmol). Then the mixture was placed in a Teflon reactor and heated at 150 °C for 72 h. Brown and long strip crystals were obtained after the reaction system was cooled to room temperature slowly. The product was filtered and washed with DMA. The yield was 82% based on H2DMTDC. The final empirical formula was determined by combining crystallographic data, and elemental and thermogravimetric analysis data. Elemental analysis calcd: C, 39.33; H, 4.95; N, 3.27. Found: C, 39.21; H, 4.98; N, 3.28. IR data (KBr pellet cm−1): 3286 (w), 3007 (w), 2934 (w), 2858 (w), 2464 (w), 1663 (w), 1614 (m), 1534 (w), 1497 (m), 1420 (w), 1391 (m), 1362 (s), 1334 (m), 1249 (w), 1183 (w), 1012 (m), 969 (w), 895 (w), 797 (w), 768 (m), 678 (w), 652 (w), 598 (w). (C2H6NH2)+[Ni3(μ3-OH)(H2O)3(DMTDC)3](C2H5OH)(DMA)2 (Ni-MOF1, DMTDC2− = 3,4-dimethylthieno[2,3-b]thiophene-2,5-dicarboxylate).
2.2. CO2 photoreduction
The procedure for photoreduction of CO2 was carried out in a sealed quartz reactor (50 mL). A circulating cooling water system was used to maintain the reaction temperature at around 20 °C. In a typical reduction reaction, Ni-MOF1 (1 mg), [Ru(bpy)3]Cl2 (Shanghai Bidepharmatech Co., Ltd) (bpy = 2′,2-bipyridine, 7 mg), water (1 mL), acetonitrile (MeCN, 4 mL) and triethanolamine (TEOA, 1 mL) were placed in a quartz reactor. Then the quartz reactor was filled with a low concentration of CO2 (CO2/Ar v/v). The system was irradiated using a 300 W Xe lamp with a cut-off filter (λ > 420 nm). To detect the composition of gas products, 0.5 mL of gas products were sampled and injected into an FID. The yield of CO was calibrated with standard gas mixtures and their identity was determined using their retention time (1.9 min for CO). The injector and detector temperatures were set to 60 °C. To detect the content of H2, 1 mL of the gas products were injected into a TCD. Subsequently, standard gas mixtures and retention time (0.7 min for H2) were used to determine the identity and calibrate the yield of H2.
To explore the source of CO produced by photocatalytic reduction of CO2, gas chromatography-mass spectrometry (GC-MS) was used to detect the products after an isotopic experiment. In this isotopic experiment, the reaction conditions were the same as described above except that 12CO2 was replaced by 13CO2.
3. Results and discussion
3.1. Characterization of the structure
Single-crystal X-ray structural analysis revealed that Ni-MOF1 crystallizes in the P63/mmc space group. The asymmetric unit consists of one Ni(II) ion, one coordinated water molecule, one third μ3-OH−, and one DMTDC2− ligand. Every Ni(II) ion is six-coordinated with four oxygen atoms from four different DMTDC2− ligands, one coordinated water molecule, and one μ3-OH−. The structure of the crystal consists of Ni3(μ3-OH) cores and DMTDC2− ligands, which is similar to the structure of MIL-88B (Fig. 1a).40 Five Ni3(μ3-OH) cores and six DMTDC2− ligands form a trigonal bipyramid-type cage (Fig. 1b). From a topological perspective, the whole structure of Ni-MOF1 can be described as a 6-connected acs network (Fig. 1c). Running along the c axis, there are hexagonal honeycomb porous channels with a pore size of about 10.1 Å (Fig. 1d). The infrared (IR) spectrum was used to elucidate the connection between the metal and the ligand in Ni-MOF1 (Fig. 2a). It displays saturated hydrocarbon stretching vibrations at 2934 cm−1 and 2858 cm−1, and carbonyl vibration at 1663 cm−1. Meanwhile, the peaks at 1497 cm−1 and 1420 cm−1 are attributed to the skeletal vibrations of the aromatic ring. The flexural vibrational peak of saturated hydrocarbons is observed at 1391 cm−1. No vibrational peak of the oxygen–hydrogen (hydroxyl) bond in the carboxyl group was observed, indicating that the Ni(II) atom of Ni-MOF1 is coordinated with the carbonyl oxygen of the carboxyl group in the ligand. Powder X-ray diffraction (PXRD) was used to determine the phase purity of Ni-MOF1. A good agreement between peak positions of the simulated prototype and the synthesized material proved the crystallinity and high purity of Ni-MOF1 (Fig. 2b). The difference between them may be due to the various crystal orientations of the powder samples.41–43
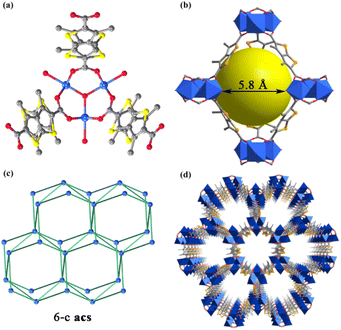 |
| Fig. 1 The structure of Ni-MOF1. (a) The coordination environment of inorganic SBUs. (b) The cage structure. (c) The 6-connected topology network. (d) View of the 3D structures along the c axis (blue balls: Ni; red balls: O; yellow balls: S; and grey balls: C). | |
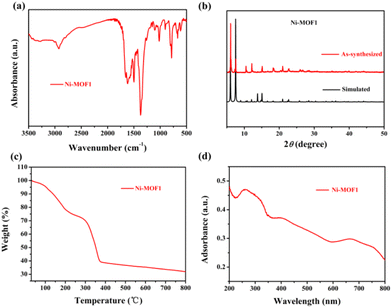 |
| Fig. 2 Basic characterization of Ni-MOF1. (a) Infrared (IR) spectra. (b) Powder X-ray diffraction (PXRD) (black curve: single-crystal simulation and red curve: experimental synthesis). (c) TGA curves. (d) UV-vis diffuse reflectance spectra. | |
To investigate the thermal stability of Ni-MOF1, thermogravimetric analysis (TGA) was conducted from 35 °C to 800 °C in N2 (Fig. 2c). The framework remains intact up to 294 °C, and above that temperature the structure starts to decompose. To understand the light-absorption ability of Ni-MOF1, UV-vis diffuse reflectance was tested. It showed that Ni-MOF1 has broad absorption in the visible light region (380–700 nm) (Fig. 2d).
3.2. CO2 photoreduction
Considering that the CO2 concentration in the exhaust emission of the heavy industry sector is between 3 and 20%, the reactivity of Ni-MOF1 in low CO2 concentration was first investigated by performing reduction reactions in nitrogen diluted CO2 with 5%, 10%, and 20% CO2 concentrations under the model conditions of acetonitrile, H2O, [Ru(bpy)3]Cl2 and TEOA (Fig. 3a). When 5% CO2 was used, the CO yield could reach 17.4 mmol g−1 while the selectivity was only 72.8% after reacting for three hours, which may be attributed to inadequate CO2 and the unused reductive sites in the production of H2. When 10% CO2 and 20% CO2 were used, the yields of CO could be increased to 23.1 mmol g−1 and 26.3 mmol g−1 with the selectivities of 84.3% and 89.2%, respectively. Meanwhile, an apparent quantum yield (A.Q.Y.) of approximately 2.1% at 420 nm and 10% CO2 concentration was identified, which is higher than the A.Q.Y. of most reported MOFs at the same concentration of CO2 (Table S2†).35,36,44,45 To trace the source of the generated CO, an isotopic experiment was performed with 13CO2 as the substrate under similar conditions of CO2 photoreduction and chromatography-mass spectrometry (GC-MS) was used to examine the products. The strong m/z peak signal at 29 was assigned to 13CO, which clearly indicated that the produced CO originates from photoreduction of CO2 instead of other species in the reaction system (Fig. S1, ESI†).
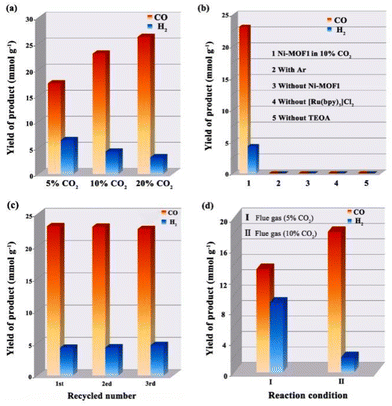 |
| Fig. 3 CO2 photoreduction performance and recycling. (a) Ni-MOF1 under different CO2 concentrations. (b) Ni-MOF1 under various reaction conditions. (c) Cycling tests under 3 h of irradiation in a 10% CO2 atmosphere over Ni-MOF1. (d) The yield of CO and H2 on Ni-MOF1 in real flue gas. | |
To elucidate the effect of each component of the system on CO2 photoreduction, four sets of control experiments were performed (Fig. 3b). No CO and H2 production was observed in the presence of Ar, indicating that the products did not come from the degradation of organic matter in the reaction system. In the absence of Ni-MOF1 or [Ru(bpy)3]Cl2, no CO and H2 were produced, demonstrating that Ni-MOF1 and [Ru(bpy)3]Cl2 are indispensable. And no products were detected in the absence of TEOA, indicating that TEOA as a sacrificial reagent is crucial for CO2 photoreduction. Cycling experiments revealed that the stability of Ni-MOF1 at 10% CO2 concentration was relatively good although a slight decline of CO production in the third reaction run was observed. The slight decline was probably owing to a little weight loss of Ni-MOF1 when cleaning and transferring (Fig. 3c).
Due to the high catalytic activity of Ni-MOF1 at low CO2 concentrations, the performance of this system towards photoreduction of CO2 directly from flue gas (5% and 10% CO2 concentrations) was investigated. Of note, yields of CO of 13.4 and 18.2 mmol g−1 could still be obtained in the flue gas with 5% and 10% CO2 concentration, respectively (Fig. 3d). Peculiarly, a high selectivity of 90.4% was observed for the proposed catalyst in the flue gas with 10% CO2 concentration. Comparing the yields of CO in 5% CO2 (17.4 mmol g−1) and 10% CO2 (23.1 mmol g−1), the slight decrease in yield may be mainly attributed to the competitive adsorption and negative effect of other components such as H2S, SOx and NOx.46 These results indicated that Ni-MOF1 showed pretty good catalytic performance even under harsh reaction conditions, which would provide a valuable guideline to design efficient photocatalysts for practical applications of CO2 photoreduction.
3.3. Possible catalytic mechanism
To reveal the root reason behind the high reactivity of the system, an in situ TPV test was carried out in CO2 and N2-saturated ACN solutions. As shown in Fig. 4a, c, and d, the curves of CO2 and N2 obtained from Ni-MOF1 are almost the same while the intensity of the curves of CO2 obtained from the Ru complex and Ru complex/Ni-MOF1 is lower than that of N2. Compared with that in the Ru complex, a higher intensity curve of N2 (Fig. 4b) was identified in Ru complex/Ni-MOF1. The above results show that the Ni-MOF1/Ru complex is preponderant in the generation of photoelectrons and is more favorable as an active center for CO2 photoreduction. For the Ru complex and Ru complex/Ni-MOF1, the equalization (Q0 − Q1)/Q1 (the ratio of used number of electrons in CO2 reduction to the total number of photogenerated electrons) was calculated to be 66.83% and 74.37% (Fig. 4c and d), respectively. A slightly lower value for Ru complex/Ni-MOF1 (66.83%) than that for the Ru complex reveals that the bare Ru complex has a faster reaction rate for CO2 reduction.
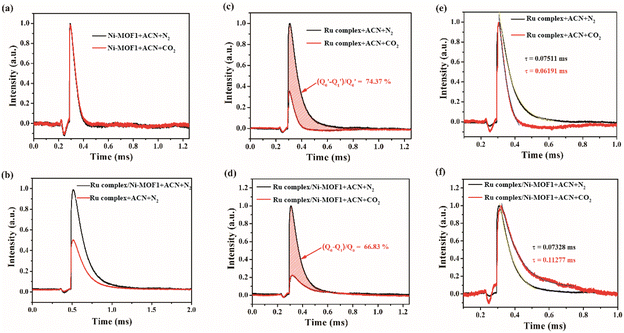 |
| Fig. 4 The in situ TPV patterns of different materials in N2-saturated ACN solution (black curve) and CO2-saturated ACN solution (red curve). (a) Ni-MOF1. (b) Ru complex/Ni-MOF1 (black curve) and Ru complex (red curve) in N2-saturated ACN solution. (c) Ru complex. (d) Ru complex/Ni-MOF1. Charge annihilation process of (e) the Ru complex and (f) Ru complex/Ni-MOF1. | |
Furthermore, a detailed analysis of the relaxation process using the TPV curves of Ru complex/Ni-MOF1 and Ru complex was conducted. According to the normalized curves of N2 and CO2 (Fig. 4e and f), the τ value, representing the time for electron and hole recombination, is also calculated. The τ of the Ru complex under N2 conditions (0.07511 ms) is larger than that under CO2 conditions (0.06191 ms) (Fig. 4e). This suggests that the Ru complex can transfer electrons directly to CO2 for the next reaction step. However, the τ of Ru complex/Ni-MOF1 under N2 conditions (0.07328 ms) is smaller than that under CO2 conditions (0.11277 ms) (Fig. 4f). These results indicate that a new photoelectric interface is generated in the CO2/Ru complex/Ni-MOF1 system. In other words, there is a new CO2 bridged photocatalytic interface between Ni-MOF1 and the Ru complex. Notably, this kind of CO2 bridged photocatalytic interface shows a less conductive characteristic than the Ru complex/Ni-MOF1 catalytic interface, but the CO2 bridged nature is beneficial for the photoreduction of low-concentration CO2. According to the reported study,47 the interface has some storage capacity for charge, and therefore it slows down the rate of electron-charge transport. A slower electron transfer process will ensure that the photoexcited charge on the sample surface is maintained for a longer period of time. The extended lifetime of the electrons is an important factor in facilitating the photocatalytic process.
Density functional theory (DFT) calculations were performed to further understand the reaction mechanism of CO2 reduction to CO. In combination with the results of TPV, the free energy changes (ΔG) on the sites of bare Ni-MOF1, the Ru complex and the CO2 bridged interface of Ru complex/Ni-MOF1 for CO2 reduction was calculated. A key step in the reported mechanism is the activation of the classically inert CO2. In our calculations, ΔG values of COOH* formation on the Ru complex, bare Ni-MOF1 and the Ru complex/Ni-MOF1 interface were 2.15, 0.57 and 0.44 eV, respectively, indicating that the COOH* formed on the Ru complex/Ni-MOF1 interface is more stable (Fig. 5a). In the subsequent step, COOH* will be hydrogenated to CO* by reacting with another proton–electron pair. The ΔG for COOH* hydrogenation to form CO* + H2O is −0.46 and −0.25 eV, respectively. Besides, due to the weak adsorption of CO on the Ru complex, CO can directly desorb from the substrate with a ΔG of −1.52 eV. The final step is the CO desorption from Ni-MOF1 and the Ru complex/Ni-MOF1 interface after overcoming the larger thermodynamic barrier. Compared with bare Ni-MOF1 as catalyst sites, the synergistic catalytic process of the Ru complex and Ni-MOF1 results in lower energy (ΔGmax = 0.44 eV) and more stable intermediates. Thus the CO2 photoreduction is prone to occur on the CO2 bridged Ru complex/Ni-MOF1 interface (Fig. 5b). The high catalytic yield at low CO2 concentrations may be ascribed to this synergistic catalytic process.
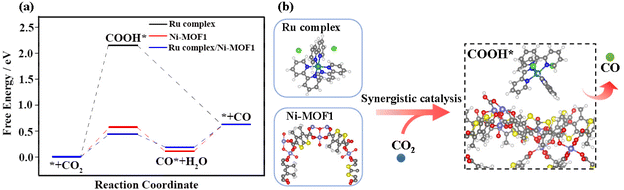 |
| Fig. 5 The DFT results and possible mechanism. (a) Free energy profile for the CO2RR on Ni-MOF1 and Ru complex/Ni-MOF1 (the free energy zero is set as that of a CO2 molecule in the gas phase and the catalyst). (b) Synergistic effect of a CO2 bridged photocatalytic interface of the Ru complex and Ni-MOF1. | |
4. Conclusions
In summary, a new kind of anionic MOF (Ni-MOF1) based on a trinuclear nickel cluster and DMTDC2− ligand was constructed for direct CO2 photoreduction from flue gas. Under visible light, a CO yield of 23.1 mmol g−1 and an A.Q.Y. of 2.1% were achieved with the assistance of the Ru complex in diluted CO2 with a concentration of 10%. The A.Q.Y. obtained in this work is comparable to the highest values of all reported photocatalysts under similar conditions. More importantly, in the flue gas containing 10% CO2 concentration, CO generation was still 18.2 mmol g−1 with a selectivity of 90.4%. TPV experiments and DFT calculations indicated that the synergistic catalysis of a CO2 bridged photocatalytic interface of the Ru complex and Ni-MOF1 played an important role in CO2 photoreduction, which might be the reason for the high reactivity in low concentration of CO2. This work provides new insights not only for the construction of a catalytic interface in CO2 photoreduction but also for the development of efficient photocatalysts for CO2 reduction directly from flue gas.
Author contributions
M. D. and Y. T. contributed equally to this paper. M. D. and C. Y. S. conceived the ideas. M. D. and X. H. W. designed the experiments, synthesized the sample, measured the photocatalytic performance and wrote the paper. L. X. W. assisted in performing photocatalysis experiments. Y. T. was responsible for the theoretical calculation part. B. S. H. was responsible for crystal analysis. J. W. was responsible for TPV analysis. A. Y. was responsible for article polishing. C. Y. S. and Z. H. K. supervised the project, analyzed the data and revised the paper. J. X. G. helped analyze data and modified the article. X. L. W. and Z. M. S. supervised the project and analyzed the data.
Conflicts of interest
There are no conflicts to declare.
Acknowledgements
This research was made possible as a result of a generous grant from the National Natural Science Foundation of China (21971032 and 22175033) and the Education Department of Jilin Province (JJKH20221153KJ).
References
- H. Dong, X. Zhang, Y. Lu, Y. Yang, Y.-P. Zhang, H.-L. Tang, F.-M. Zhang, Z.-D. Yang, X. Sun and Y. Feng, Regulation of metal ions in smart metal-cluster nodes of metal-organic frameworks with open metal sites for improved photocatalytic CO2 reduction reaction, Appl. Catal., B, 2020, 276, 119173–119195 CrossRef CAS.
- X. Chen, Q. Li, M. Zhang, J. Li, S. Cai, J. Chen and H. Jia, MOF-Templated Preparation of Highly Dispersed Co/Al2O3 Composite as the Photothermal Catalyst with High Solar-to-Fuel Efficiency for CO2 Methanation, ACS Appl. Mater. Interfaces, 2020, 12, 39304–39317 CrossRef CAS PubMed.
- X. Qi, R. Zhong, M. Chen, C. Sun, S. You, J. Gu, G. Shan, D. Cui, X. Wang and Z. Su, Single Metal–Organic Cage Decorated with an Ir(III) Complex for CO2 Photoreduction, ACS Catal., 2021, 11, 7241–7248 CrossRef CAS.
- X. Yu, C.-C. Zhao, J.-X. Gu, C.-Y. Sun, H.-Y. Zheng, L.-K. Yan, M. Sun, X.-L. Wang and Z.-M. Su, Transition-Metal-Modified Vanadoborate Clusters as Stable and Efficient Photocatalysts for CO2 Reduction, Inorg. Chem., 2021, 60, 7364–7371 CrossRef CAS PubMed.
- Z. Chen, Y. Hu, J. Wang, Q. Shen, Y. Zhang, C. Ding, Y. Bai, G. Jiang, Z. Li and N. Gaponik, Boosting Photocatalytic CO2 Reduction on CsPbBr3 Perovskite Nanocrystals by Immobilizing Metal Complexes, Chem. Mater., 2020, 32, 1517–1525 CrossRef CAS.
- F. Guo, S. Yang, Y. Liu, P. Wang, J. Huang and W.-Y. Sun, Size Engineering of Metal–Organic Framework MIL-101(Cr)–Ag Hybrids for Photocatalytic CO2 Reduction, ACS Catal., 2019, 9, 8464–8470 CrossRef CAS.
- X. Deng, J. Albero, L. Xu, H. García and Z. Li, Construction of a Stable Ru–Re Hybrid System Based on Multifunctional MOF-253 for Efficient Photocatalytic CO2 Reduction, Inorg. Chem., 2018, 57, 8276–8286 CrossRef CAS PubMed.
- W. Zhu, C. Zhang, Q. Li, L. Xiong, R. Chen, X. Wan, Z. Wang, W. Chen, Z. Deng and Y. Peng, Selective reduction of CO2 by conductive MOF nanosheets as an efficient co-catalyst under visible light illumination, Appl. Catal., B, 2018, 238, 339–345 CrossRef CAS.
- I. I. Alkhatib, C. Garlisi, M. Pagliaro, K. Al-Ali and G. Palmisano, Metal-organic frameworks for photocatalytic CO2 reduction under visible radiation: A review of strategies and applications, Catal. Today, 2020, 340, 209–224 CrossRef CAS.
- M. Lu, J. Liu, Q. Li, M. Zhang, M. Liu, J.-L. Wang, D.-Q. Yuan and Y.-Q. Lan, Rational Design of Crystalline Covalent Organic Frameworks for Efficient CO2 Photoreduction with H2O, Angew. Chem., Int. Ed., 2019, 58, 12392–12397 CrossRef CAS PubMed.
- A. Crake, K. C. Christoforidis, A. Gregg, B. Moss, A. Kafizas and C. Petit, The Effect of Materials Architecture in TiO2/MOF Composites on CO2 Photoreduction and Charge Transfer, Small, 2019, 15, 1805473–1805484 CrossRef PubMed.
- X.-Y. Dao, J.-H. Guo, Y.-P. Wei, F. Guo, Y. Liu and W.-Y. Sun, Solvent-Free Photoreduction of CO2 to CO Catalyzed by Fe-MOFs with Superior Selectivity, Inorg. Chem., 2019, 58, 8517–8524 CrossRef CAS PubMed.
- C. Gao, Q. Meng, K. Zhao, H. Yin, D. Wang, J. Guo, S. Zhao, L. Chang, M. He, Q. Li, H. Zhao, X. Huang, Y. Gao and Z. Tang, Co3O4 Hexagonal Platelets with Controllable Facets Enabling Highly Efficient Visible-Light Photocatalytic Reduction of CO2, Adv. Mater., 2016, 28, 6485–6490 CrossRef CAS PubMed.
- M. Liu, Y.-F. Mu, S. Yao, S. Guo, X.-W. Guo, Z.-M. Zhang and T.-B. Lu, Photosensitizing single-site metal–organic framework enabling visible-light-driven CO2 reduction for syngas production, Appl. Catal., B, 2019, 245, 496–501 CrossRef CAS.
- H.-Q. Xu, J. Hu, D. Wang, Z. Li, Q. Zhang, Y. Luo, S.-H. Yu and H.-L. Jiang, Visible-Light Photoreduction of CO2 in a Metal–Organic Framework: Boosting Electron–Hole Separation via Electron Trap States, J. Am. Chem. Soc., 2015, 137, 13440–13443 CrossRef CAS PubMed.
- D. Li, M. Kassymova, X. Cai, S.-Q. Zang and H.-L. Jiang, Photocatalytic CO2 reduction over metal-organic framework-based materials, Coord. Chem. Rev., 2020, 412, 213262 CrossRef CAS.
- J. Li, H. Huang, W. Xue, K. Sun, X. Song, C. Wu, L. Nie, Y. Li, C. Liu, Y. Pan, H.-L. Jiang, D. Mei and C. Zhong, Self-adaptive dual-metal-site pairs in metal-organic frameworks for selective CO2 photoreduction to CH4, Nat. Catal., 2021, 4, 719–729 CrossRef CAS.
- G. V. Last and M. T. Schmick, A review of major non-power-related carbon dioxide stream compositions, Environ. Earth Sci., 2015, 74, 1189–1198 CrossRef CAS.
- A. Goeppert, M. Czaun, J.-P. Jones, G. K. Surya Prakash and G. A. Olah, Recycling of carbon dioxide to methanol and derived products–closing the loop, Chem. Soc. Rev., 2014, 43, 7995–8048 RSC.
- P.-Q. Liao, X.-W. Chen, S.-Y. Liu, X.-Y. Li, Y.-T. Xu, M. Tang, Z. Rui, H. Ji, J.-P. Zhang and X.-M. Chen, Putting an ultrahigh concentration of amine groups into a metal–organic framework for CO2 capture at low pressures, Chem. Sci., 2016, 7, 6528–6533 RSC.
- W. Sun, X. Cao, W. Yang and X. Jin, Numerical simulation of CO2 condensation process from CH4-CO2 binary gas mixture in supersonic nozzles, Sep. Purif. Technol., 2017, 188, 238–249 CrossRef CAS.
- G. Jiang, Q. Huang, S. D. Kenarsari, X. Hu, A. G. Russell, M. Fan and X. Shen, A new mesoporous amine-TiO2 based pre-combustion CO2 capture technology, Appl. Energy, 2015, 147, 214–223 CrossRef CAS.
- A. Hart and N. Gnanendran, Cryogenic CO2 capture in natural gas, Energy Procedia, 2009, 1, 697–706 CrossRef CAS.
- A. L. Khan, X. Li, A. Ilyas, M. T. Raza and I. F. J. Vankelecom, Novel sulfonated and fluorinated PEEK membranes for CO2 separation, Sep. Purif. Technol., 2016, 167, 1–5 CrossRef CAS.
- J.-D. Xiao and H.-L. Jiang, Metal–Organic Frameworks for Photocatalysis and Photothermal Catalysis, Acc. Chem. Res., 2019, 52, 356–366 CrossRef CAS PubMed.
- R. Li, W. Zhang and K. Zhou, Metal–Organic-Framework-Based Catalysts for Photoreduction of CO2, Adv. Mater., 2018, 30, 1705512–1705542 CrossRef PubMed.
- A. Dhakshinamoorthy, A. M. Asiri and H. García, Metal–Organic Framework (MOF) Compounds: Photocatalysts for Redox Reactions and Solar Fuel Production, Angew. Chem., Int. Ed., 2016, 55, 5414–5445 CrossRef CAS PubMed.
- X. Feng, Y. Pi, Y. Song, C. Brzezinski, Z. Xu, Z. Li and W. Lin, Metal–Organic Frameworks Significantly Enhance Photocatalytic Hydrogen Evolution and CO2 Reduction with Earth-Abundant Copper Photosensitizers, J. Am. Chem. Soc., 2020, 142, 690–695 CrossRef CAS PubMed.
- S. N. Habisreutinger, L. Schmidt-Mende and J. K. Stolarczyk, Photocatalytic Reduction of CO2 on TiO2 and Other Semiconductors, Angew. Chem., Int. Ed., 2013, 52, 7372–7408 CrossRef CAS PubMed.
- T. Kajiwara, M. Fujii, M. Tsujimoto, K. Kobayashi, M. Higuchi, K. Tanaka and S. Kitagawa, Photochemical Reduction of Low Concentrations of CO2 in a Porous Coordination Polymer with a Ruthenium(II)–CO Complex, Angew. Chem., Int. Ed., 2016, 55, 2697–2700 CrossRef CAS PubMed.
- D. Wang, R. Huang, W. Liu, D. Sun and Z. Li, Fe-Based MOFs for Photocatalytic CO2 Reduction: Role of Coordination Unsaturated Sites and Dual Excitation Pathways, ACS Catal., 2014, 4, 4254–4260 CrossRef CAS.
- H.-X. Zhang, Q.-L. Hong, J. Li, F. Wang, X. Huang, S. Chen, W. Tu, D. Yu, R. Xu, T. Zhou and J. Zhang, Isolated Square-Planar Copper Center in Boron Imidazolate Nanocages for Photocatalytic Reduction of CO2 to CO, Angew. Chem., Int. Ed., 2019, 58, 11752–11756 CrossRef CAS PubMed.
- W. Yang, H.-J. Wang, R.-R. Liu, J.-W. Wang, C. Zhang, C. Li, D.-C. Zhong and T.-B. Lu, Tailoring Crystal Facets of Metal–Organic Layers to Enhance Photocatalytic Activity for CO2 Reduction, Angew. Chem., Int. Ed., 2021, 60, 409–414 CrossRef CAS PubMed.
- B. Hou, C. Qin, C. Sun, X. Wang and Z. Su, Stepwise Construction of Multivariate Metal–Organic Frameworks from a Predesigned Zr16 Cluster, CCS Chem., 2021, 3, 287–293 CrossRef CAS.
- B. Han, X. Ou, Z. Deng, Y. Song, C. Tian, H. Deng, Y.-J. Xu and Z. Lin, Nickel Metal–Organic Framework Monolayers for Photoreduction of Diluted CO2: Metal-Node-Dependent Activity and Selectivity, Angew. Chem., Int. Ed., 2018, 57, 16811–16815 CrossRef CAS PubMed.
- Y. Wang, N.-Y. Huang, J.-Q. Shen, P.-Q. Liao, X.-M. Chen and J.-P. Zhang, Hydroxide Ligands Cooperate with Catalytic Centers in Metal–Organic Frameworks for Efficient Photocatalytic CO2 Reduction, J. Am. Chem. Soc., 2018, 140, 38–41 CrossRef CAS PubMed.
- M. Dong, J.-X. Gu, C.-Y. Sun, X.-L. Wang and Z.-M. Su, Photocatalytic reduction of low-concentration CO2 by metal–organic frameworks, Chem. Commun., 2022, 58, 10114–10126 RSC.
- H. Fei, M. D. Sampson, Y. Lee, C. P. Kubiak and S. M. Cohen, Photocatalytic CO2 Reduction to Formate Using a Mn(I) Molecular Catalyst in a Robust Metal–Organic Framework, Inorg. Chem., 2015, 54, 6821–6828 CrossRef CAS PubMed.
- J. Qin, S. Wang and X. Wang, Visible-light reduction CO2 with dodecahedral zeolitic imidazolate framework ZIF-67 as an efficient co-catalyst, Appl. Catal., B, 2017, 209, 476–482 CrossRef CAS.
- H. H. Thorp, Bond valence sum analysis of metal-ligand bond lengths in metalloenzymes and model complexes, Inorg. Chem., 1992, 31, 1585–1588 CrossRef CAS.
- B. Han, J. Song, S. Liang, W. Chen, H. Deng, X. Ou, Y.-J. Xu and Z. Lin, Hierarchical NiCo2O4 hollow nanocages for photoreduction of diluted CO2: Adsorption and active sites engineering, Appl. Catal., B, 2020, 260, 118208 CrossRef CAS.
- Y. Jiang, J. Sun, X. Yang, J. Shen, Y. Fu, Y. Fan, J. Xu and L. Wang, Cd-MOF@PVDF Mixed-Matrix Membrane with Good Catalytic Activity and Recyclability for the Production of Benzimidazole and Amino Acid Derivatives, Inorg. Chem., 2021, 60, 2087–2096 CrossRef CAS PubMed.
- Y. Jiang, J. Song, Z. Xiu, L. Huang, F. Gao, S. Jiao and Y. Bi, Solvent-free syntheses of two pcu topological indium phosphite-oxalates with a novel butterfly motif and proton conductivity, Microporous Mesoporous Mater., 2019, 289, 109643 CrossRef CAS.
- T.-T. Zhang, G. Li and X.-B. Cui, Three New Polyoxoniobates Functioning as Different Oxidation Catalysts, Cryst. Growth Des., 2021, 21, 3191–3201 CrossRef CAS.
- W. Zhong, R. Sa, L. Li, Y. He, L. Li, J. Bi, Z. Zhuang, Y. Yu and Z. Zou, A Covalent Organic Framework Bearing Single Ni Sites as a Synergistic Photocatalyst for Selective Photoreduction of CO2 to CO, J. Am. Chem. Soc., 2019, 141, 7615–7621 CrossRef CAS PubMed.
- S.-H. Guo, X.-J. Qi, H.-M. Zhou, J. Zhou, X.-H. Wang, M. Dong, X. Zhao, C.-Y. Sun, X.-L. Wang and Z.-M. Su, A bimetallic-MOF catalyst for efficient CO2 photoreduction from simulated flue gas to value-added formate, J. Mater. Chem. A, 2020, 8, 11712–11718 RSC.
- M. Dong, J. Zhou, J. Zhong, H. T. Li, C. Y. Sun, Y. D. Han, J. N. Kou, Z. H. Kang, X. L. Wang and Z. M. Su, CO2 Dominated Bifunctional Catalytic Sites for Efficient Industrial Exhaust Conversion, Adv. Funct. Mater., 2021, 2110136 Search PubMed.
Footnotes |
† Electronic supplementary information (ESI) available. CCDC 2061508. For ESI and crystallographic data in CIF or other electronic format see DOI: https://doi.org/10.1039/d2qi02366k |
‡ These authors contributed equally. |
|
This journal is © the Partner Organisations 2023 |
Click here to see how this site uses Cookies. View our privacy policy here.