DOI:
10.1039/D1QI01122G
(Research Article)
Inorg. Chem. Front., 2022,
9, 60-69
Intramolecular π-conjugated channel expansion achieved by doping cross-linked dopants into carbon nitride frameworks for propelling photocatalytic hydrogen evolution and mechanism insight†
Received
5th September 2021
, Accepted 21st October 2021
First published on 22nd October 2021
Abstract
Developing modified carbon nitride (CN) with a multi-bonding mode remains a pivotal challenge for high-efficiency photocatalytic hydrogen evolution (PHE). Here, we fabricate a PYM-CN photocatalyst by doping pyrimethamine (PYM) in CN by a facile one-step polymerization method, which shows remarkably enhanced PHE performance in contrast with pure CN, because PYM as a cross-linked bridge in CN frameworks via a multi-bonding mode broadens the visible-light absorption range and expedites the transfer and separation of intramolecular photoproduced electron–hole pairs by π-conjugated channel expansion. Density functional theory (DFT) calculations reveal that this significant improvement is attributed to the orbital hybridization and delocalization effects resulting from the unique intramolecular cross-linked structure in PYM-CN. As expected, the PHE rate over the optimal PYM100-CN sample is about 11.6 times that of pure CN and the AQE value reaches up to 17.7% at 420 nm. Hence, this work provides a representative paradigm to exploit intramolecular cross-linked polymer photocatalysts with a multi-bonding mode for the high-efficiency PHE reaction or other photocatalytic application fields.
Introduction
With the gradual depletion of fossil fuels, finding a sustainably clean energy source is a top priority. Hydrogen as an energy carrier has attracted worldwide attention due to its high heat value of combustion as well as low environmental pollution.1–4 In the current reported methods, photocatalytic hydrogen evolution (PHE) from water splitting using solar energy is considered as a promising sustainably green technology for satisfying practical applications in the future.5–8 Up to now, how to optimize the separation efficiency of photogenic charge carriers and expand the visible-light absorption range of photocatalysts have been the most critical issues to enhance PHE activity. It is reported that unembellished photocatalysts always fail to achieve satisfactory results for practical application because they must suffer the worst separation capacity of photogenerated charge carriers and conversion efficiency of solar energy.9–12 Therefore, researchers have concentrated on developing a suitable modification strategy to improve the PHE activity remarkably in recently years.
Plenty of investigations show that high-efficiency PHE activity can be achieved by reasonably modifying carbon nitride (CN) polymer photocatalysts,13–16 especially for incorporation of organic small molecules into conjugated frameworks of CN polymer, and it has been deemed as a new effective strategy to adjust the energy band structure, accelerate charge carrier separation, extend visible light absorption and thus boost PHE performance.17–19 Significantly, the π–π stacking configurations between molecular frameworks and organic small molecules can provide more photogeneration electron transport channels to accelerate separation of electron–hole pairs, where small molecule dopants involve various bonding modes with conjugated CN frameworks.20,21 For instance, polycyclic aromatic compounds as dopants with different amounts of aromatic rings are introduced into the internal CN framework in the manner of a single bonding mode. All the as-prepared photocatalysts display narrowed bandgaps, conducive separation of photogenerated electron–hole pairs and improved PHE activity compared with pure CN through adjusting the π-conjugated electronic structure and strengthening the polarizability of π-electrons.22 Again, in the single bonding mode, our group grafts pyridine rings as the conjugated nitrogen heterocycle on the structural edge of CN and the optimal PHE performance is boosted dramatically, which is due to the dual synergy function of modulating electron hybridization structure, along with the directional transfer from the center to the structural edge of CN.23 Besides, there have been many reports on the double bonding mode of small molecule dopants in the polymer photocatalysts because of their more obvious advantages.24,25 The most common is the architecture of donor–acceptor (D–A) conjugated polymers owing to their unrestrained intramolecular charge transfer and reduced electrostatic attraction between charge carriers for the conducive separation of bulk/surface excitons.26,27 For instance, the CN-based D–A conjugated polymer with polyporous structure is facilely prepared by the double coordination mode of melamine formaldehyde resin, which improves the light utilization and charge carriers’ separation owing to the ameliorative electron-accepting ability, thus boosting the PHE activity.28 The above investigations indicate that adjusting the bonding modes of small molecule dopants may produce the interesting effects on PHE activity. More noteworthily, the conjugated polymer with a multi-bonding mode has a unique cross-linked structure, in which the augmented π-conjugation length favors delocalization and transport of charge carriers and the highly conjugated level and orderliness facilitate adequate utilization of redox active sites, with the inherent property of large surface area enhancing the interaction with water molecules and so on.29,30 Therefore, it is vital to design the appropriate cross-linked structure which is involved in more than two linked sites, delocalized π-conjugation nature, electron donor–acceptor capacity, etc.31,32 So it encourages us to prepare conjugated polymer photocatalysts such as CN with a multi-bonding mode to realize intramolecular π-conjugated channel expansion for high-efficiency synergistic PHE reaction.
Herein, we designed and prepared a novel cross-linked π-conjugated polymer via one-step polymerization of urea with pyrimethamine (PYM). The as-prepared PYM-CN samples exhibit a extended π-conjugated structure. As expected, the PYM-CN samples not only have a strong visible light absorption capacity and easily adjusted band gap by changing the amount of PYM, but also exhibit rapid carrier separation and transfer capacity. As a consequence, the PHE rate (5.418 mmol h−1 g−1) over the optimal PYM100-CN sample is approximately 11.6 times that of pure CN and the AQE value at 420 nm reaches to 17.7%.
Experimental
Materials
Urea (AR, ≥99%), H2PtCl6·H2O (AR, ≥99%) and ethanol (AR, ≥99%) were purchased from Sinopharm Chemical Reagent Co. Ltd. Pyrimethamine (PYM) (AR, ≥98%), triethanolamine (TEOA) (AR, ≥98%) and Na2SO4 (AR, ≥99%) were obtained from Aladdin Chemistry Co. Ltd. All chemicals were not further purified.
Synthesis of samples
The synthesis of PYM-CN was as follows: 80 g of dried urea and a certain amount of pyrimethamine was put in a mortar and ground evenly. After that, it was heated in air at a rate of 2.5 °C min−1 to 550 °C for 4 h in a 50 mL alumina crucible with a lid. After cooling to room temperature, the samples were obtained and denoted as PYMx-CN (x = 25, 50, 100, 150, 250), where x (mg) represents the mass of the added PYM. The possible reaction schematic illustration of PYM-CN is shown in Scheme 1. In addition, the preparation of pure CN is exactly the same as the above process without adding PYM.
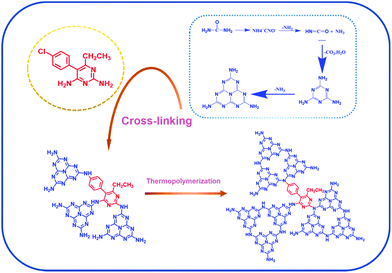 |
| Scheme 1 The possible synthetic reaction schematic illustration of PYM-CN. | |
Characterization of samples
The structure of the samples was determined on a DX-2700 model X-ray diffractometer (XRD) with Cu Kα radiation. The Fourier transform infrared (FT-IR) spectrum was measured on a Nicolet-560 spectrometer with KBr as the reference. The 13C solid-state nuclear magnetic resonance (NMR) spectrum was collected by a Bruker AVANCE III 400 nuclear magnetic resonance spectrometer. The scanning electron microscope (SEM) image was obtained using a JSM-7001F. The transmission electron microscope (TEM) image was obtained on a JEOL JEM-2011F with an accelerating voltage of 200 kV. The MFP930 instrument was used to measure the atomic force microscope (AFM) image. X-ray photoelectron spectroscopy (XPS) was detected by a Shimadzu AXIS-Ultra DLD (Japan) X-ray photoelectron spectrometer. The electron paramagnetic resonance spectrum (EPR) was obtained by the JEOL JES FA200 spectrometer. The UV-vis diffuse reflectance spectrum (DRS) was recorded by a UV-vis spectrophotometer (UV-3600Plus) with BaSO4 as the reference. The specific surface area and pore size distribution were measured at BEL SORP (MicrotracBEL, Japan) by using on a N2 adsorption–desorption method. Photoluminescence (PL) spectroscopy and time-resolved photoluminescence (TR-PL) decay curves were detected on a Horiba JobinYvon (FluoroMax 4) luminescence spectrometer.
Photocatalytic activity measurements
The photocatalytic activity test of PYM-CN samples was carried out in a closed glass instrument. In a typical process, 20 mg of photocatalyst was uniformly dispersed in 100 ml of triethanolamine solution (10 vol%). At the same time, 3% Pt (H2PtCl6·6H2O, 0.6 ml, 1 mg ml−1 Pt) of the added photocatalyst mass was added as a co-catalyst. Finally, argon was used to remove the air in the reactor. A 300 W xenon lamp was used as the light source and was equipped with a 420 nm cut-off filter. The temperature was controlled at 20 °C by circulating water. The amount of hydrogen produced in the reactor was detected by the thermal conductivity detector (TCD) of the online gas chromatography system (GC-2030). The apparent quantum efficiency (AQE) was tested by using LED lights with different wavelengths.
Electrochemical and photoelectrochemical measurements
The electrochemical and photoelectrochemical properties were tested on the VERSASTAT3 electrochemical workbench and the Shanghai Chenhua electrochemical workstation through the three-electrode model, in which a Pt sheet was used as the counter electrode, the saturated Ag/AgCl electrode was used as the reference electrode, and the sample-coated FTO glass and the sample-coated carbon paper were used as the working electrode. When testing transient photocurrent, electrochemical impedance spectroscopy (EIS) and Mott–Schottky plots, a 300 W xenon lamp equipped with a 420 nm cut-off filter was used as the visible light source, and 0.5 M sodium sulfate solution was used as the electrolyte. The preparation process of the working electrode was as follows: first, 300 mg of photocatalyst, 10 mg of PVP and 0.03 ml of oleic acid were measured and dispersed in 3 ml of ethanol by ultrasound for 6 h to form a uniform suspension. After that, the suspension was uniformly spin-coated on the FTO glass (20 mm × 20 mm) at 2000 r min−1 for 3 min, and finally vacuum dried at 80 °C for 12 h to obtain the working electrode. When testing linear sweep voltammetry (LSV) and cyclic voltammetry (CV) curves, 0.5 M H2SO4 was used as the electrolyte solution. The preparation process of the working electrode was as follows: firstly, 0.5 mg of photocatalyst was uniformly dispersed in the solution containing 960 μL of ethanol and 40 μL of Nafion. After that, 100 μL of the suspension was dripped on two sides of carbon paper (10 mm × 20 mm).
Theory calculations
The Dmol3 module in the Materials Studio software package was employed to perform DFT (Density Functional Theory) calculations.33,34 Blochl's all-electron-like PAW (Projector Augmented Wave) method was used to describe the interactions between ion cores and valence electrons, and GGA (Generalized Gradient Approximation) with PBE (Perdew–Burke–Ernzerhof) function was used for the exchange–correlation interaction.35 In calculation, the kinetic energy cutoff was 500.0 eV, convergence tolerance was 5.0 × 10−6 eV per atom, force tolerance was 0.002 eV Å−1, maximum displacement was 0.005 Å and band energy tolerance was 1.0 × 10−5 eV. The 4 × 4×1 k point within the gamma centered Monkhorst–Pack scheme was adopted to sample the Brillouin zone. When the forces on each atom are less than 0.005 eV Å−1, the lattice parameters and atomic coordinates are relaxed fully.
Results and discussion
Structure and morphology
XRD characterization studies were firstly executed to diagnose the composition and crystalline phase of the as-prepared photocatalysts. As exhibited in Fig. 1a, similar diffraction characteristics to those previously reported, two peaks located at 12.8° (100) and 27.6° (002) are presented, are ascribed to the in-plane repetitive motif of tri-s-triazine as well as the interlayer accumulation of aromatic systems, respectively. There are no other obvious diffraction peaks observed in PYM-CN, which confirms that the incoming cross-linked bridge doesn't change the original structure of CN owing to its low content or high dispersion in the framework structure.36 However, compared with pure CN, the peak intensity is decreased significantly and the width is broadened for PYM-CN with the increase of PYM content. It may be because the integration of PYM into the layer structure of CN alters its chemical environment and enlarges the in-plane packing distance, which is also proved by the enlarged view of the (002) diffraction peak regularly moving to the lower angle (Fig. 1b), suggesting that PYM can act as the cross-linked bridge to be loosely bound with CN and is most likely located among the polymer strands.
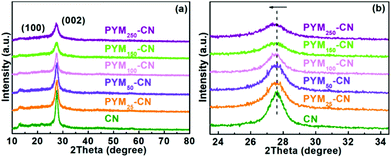 |
| Fig. 1 XRD patterns of different samples (a) and corresponding magnified image on (002) crystal planes (b). | |
To better prove that PYM was incorporated into CN frameworks, the FT-IR spectra (Fig. 2a and b) and solid-state 13C NMR spectra (Fig. 2c and d) were performed on samples. In Fig. 2a, all the PYM-CN samples show the characteristic peaks analogous with that of pristine CN, indicating that the chemical structure was maintained well after the introduction of PYM. To be specific, several absorption peaks appearing in the region of 1200–1700 cm−1 are induced by the stretching mode and vibration mode of the CN heterocyclic unit.37,38 The broad peaks among 3000–3600 cm−1 are assigned to the vibration mode of –NH2 or –OH groups.37,38 Besides, the peak at 807 cm−1 is derived from the breathing pattern of tri-s-triazine units.37,38 It is worth noting that the PYM-CN samples present a small peak at 1507 cm−1 (Fig. 2b), which results from the stretching vibration of C
C in the benzene ring.17 This result suggests consumingly that PYM should be incorporated into the CN framework and does not break the primitive CN structure, which accords with the XRD result. Moreover, it can be found through the solid 13C NMR spectrum that the two strong peaks located at 164.6 and 155.8 ppm confirm the chemical shift caused by C atoms attached to terminal C–NHx and bridging N in heptazine units (C–(N)3), respectively (Fig. 2c).39 Meanwhile, the peak intensity of PYM100-CN samples is obviously stronger in contrast with the pure CN, and the peak position has a certain shift (Fig. 2d), which further demonstrates that PYM has been incorporated into the CN framework. In addition, the gradually increased carbon contents for PYM-CN samples also certify the doping of PYM in CN (Table S1†).
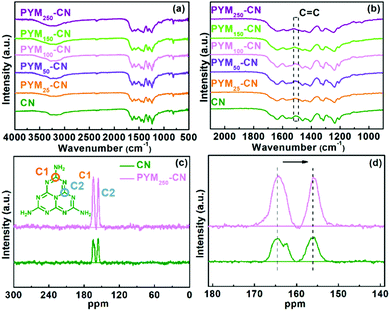 |
| Fig. 2 FT-IR spectra of different samples (a) and their magnified images (b); solid 13C NMR spectra of CN and PYM100-CN (c) and corresponding magnified images (d). | |
The morphology and microstructure of CN and PYM100-CN were studied in detail by SEM and TEM. As the SEM images show in Fig. 3a and b, both CN and PYM100-CN present a block structure, but the latter forms a relatively thinner and looser planar structure. It is owing to the inhibition effect of incoming PYM as a cross-linked bridge in CN frameworks, which results in a partly distorted structure. Moreover, TEM images in Fig. 3c and d further confirm the above information and nanosheet morphology of them. Furthermore, according to atomic force microscope (AFM) images in Fig. 3e and f, the average thickness of PYM-CN is probably ∼4.5 nm, which is thinner than that of pure CN (∼5.5 nm). The thinner and looser structure of PYM100-CN can not only provide a large number of active sites for water reduction, but also shorten the vertical diffusion distance of charge to suppress recombination of carriers, thereby further improving the PHE activity.
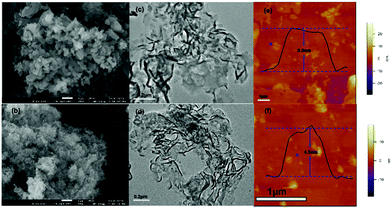 |
| Fig. 3 SEM, TEM and AFM images of pristine CN (a, c and e) and PYM100-CN (b, d and f). | |
The elemental composition and chemical state were analyzed by XPS technology. From survey XPS in Fig. 4a, both CN and PYM100-CN are mainly composed of carbon and nitrogen elements. Meanwhile, the high-resolution C 1s XPS of CN in Fig. 4b shows three peaks with 287.6, 285.6 and 284.4 eV as centers, which belong to sp2 hybridized C of C–(N)3 in the aromatic ring, sp2 C attached to the NHx group in the aromatic ring and the C of C–C/C
C bonds, respectively.40–42 For the high-resolution N 1s XPS of CN in Fig. 4c, there also are three peaks at 400.2, 398.7 and 398.0 eV, which ascribes the amino C–N–H, N–(C)3 groups and C
N–C bonds, respectively.40–42 Interestingly, the C 1s and N 1s peaks of PYM100-CN are all shifted to the high binding energy relative to that of CN, which may be attributed to the partial electron cloud density variation owing to incorporating PYM as an intramolecular cross-linked bridge into CN frameworks. It is further confirmed by following electron paramagnetic resonance (EPR) spectra. As shown in Fig. 4d, a single Lorentz line centered on g = 2.0054 is observed in CN and PYM100-CN derived from the unpaired electrons on the aromatic ring sp2-C atoms. Obviously, the EPR signal of PYM100-CN is significantly enhanced compared with CN, which indicates that the incorporation of PYM effectively extends the delocalization of unpaired electrons from the heptazine π-conjugated rings, resulting in the changed electron cloud density.28,43 It is accounted for by the incorporation of PYM in CN frameworks with a multi-bonding mode in favor of the effective separation of charge carriers, which is the main reason for the improved PHE activity.
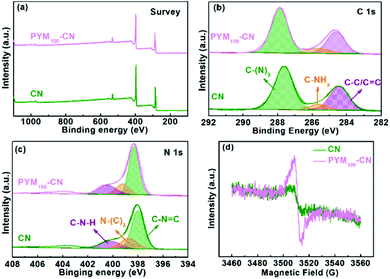 |
| Fig. 4 XPS (a–c) and EPR (d) spectra of CN and PYM100-CN. | |
Photocatalytic activity
The photocatalytic performance was evaluated by the PHE experiment under the visible-light irradiation (λ > 420 nm). As displayed in Fig. 5a, all the PYM-CN samples show higher photocatalytic activity than pure CN. The optimal PHE rate is 5.418 mmol h−1 g−1 for PYM100-CN, which is about 11.6 times that of pure CN (0.467 mmol h−1 g−1). The improved photocatalytic activity could be due to to the introduction of PYM as a cross-linked bridge into CN frameworks, which can strengthen the electronic polarizability and extend the forceful conjugated effect, resulting in more effective separation of electron–hole pairs.17 However, when further increasing the content of PYM, the PHE rate decreases in contrast, owing to the excessive PYM perhaps acting as the recombination centers of charge carriers. Moreover, Fig. 5b shows that PYM100-CN exhibits a great stability within five cycles of experiments for 20 h, which is an important indicator for the practical application of photocatalysts. And by comparing XRD spectra of PYM100-CN before and after the cyclic reactions (Fig. S1†), the similar diffraction patterns demonstrate that the crystal phase is basically unchanged, suggesting PYM100-CN exhibits outstanding recyclability and stability. Furthermore, the AQE values of PHE over PYM100-CN are measured under various monochromatic light irradiation. Fig. 5c shows that the change trend of AQE values of PYM100-CN is similar to its UV-vis DRS. A high AQE value of 17.7% is reached at 420 nm, and even at 560 nm, an AQE value of 2.2% is still obtained. It is worth noting that PYM-CN also exhibits excellent PHE activity in different water environments. As shown in Fig. 5d, when using the deionized water and purified water, PHE rates are nearly equal, which is 5.418 mmol h−1 g−1 and 5.221 mmol h−1 g−1, respectively. More amazingly, when making use of simulated seawater and municipal water, the PHE rates are significantly enhanced, which are as high as 8.351 mmol h−1 and 10.671 mmol h−1 g−1, respectively. It is most likely the result of a combination of factors such as solution pH, inorganic ion species, and dissolved organic matter.44 This discovery further broadens the practical application range of the as-prepared photocatalysts in complex water environments.
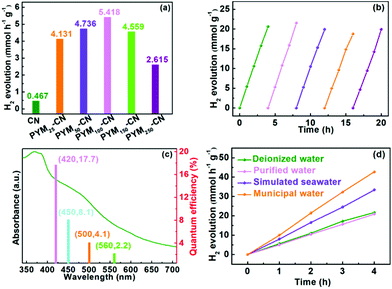 |
| Fig. 5 PHE rates of different samples (a), cyclic PHE kinetics curves of PYM100-CN (b), AQE values dependent on UV-vis DRS (c), PHE kinetics curves of PYM100-CN in different water environments (d). | |
In order to gain an insight into essential reasons for boosted PHE performance, the effect of PYM as a cross-linked bridge incorporated into CN frameworks on the physical, photoelectrochemical and electrochemical properties are investigated in detail. The optical absorption was firstly studied by the UV-vis DRS. As shown in Fig. 6a, CN has an absorption edge located at about 450 nm, but PYM-CN samples show the significant red shift phenomenon, and the absorption intensity and range gradually are increased and broadened with increasing amount of PYM, certifying their greater visible-light utilization than pure CN. Simultaneously, the band gap values (Eg) of all the prepared samples were evaluated by the plots of (αhν)2versus hν.45Fig. 6b displays that Eg values decrease correspondingly when increasing the amount of PYM, which are 2.92, 2.84, 2.80, 2.72, 2.69 and 2.66 eV for PYMx-CN (x = 0, 25, 50, 100, 150, 250) samples, respectively (Table S1†). It implies the electrons can be effortlessly excited from the valence band (VB) to the corresponding conduction band (CB) as for PYM-CN samples to improve the photocatalytic activity.
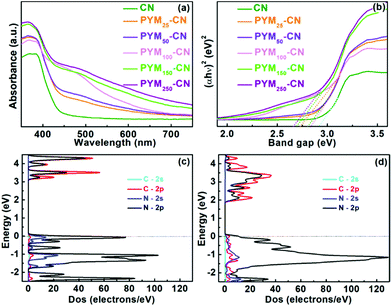 |
| Fig. 6 UV-vis DRS (a) and plots of (αhv)2versus hv (b) of different samples; PDOS of CN (c) and PYM-CN (d). | |
For insight into the variation of band structure and composition, DFT calculations are performed to investigate the influence of the introduction of PYM into CN on electronic properties. As shown in Fig. 6c and d, the partial density of states (PDOS) of pure CN and PYM-CN have similar orbital hybrid characteristics, in which the CB of them consists of the hybridized N 2p and C 2p orbitals. However, the VB of pure CN is composed of dominant N 2p and a certain amount of N 2s orbitals, while that of PYM-CN is almost absolutely occupied by N 2p orbitals, implying the more conducive electron transfer from VB to CB. Moreover, comparing the PDOS distributions between CN and PYM-CN, the latter exhibits the distinctly enhanced electron delocalization, which is conducive to facilitating charge transfer and separation in the in-plane CN framework. Besides, the theoretically calculated CB bottom decreases from 3.03 eV of pure CN to 1.80 eV of PYM-CN, which indicates the bandgap becomes narrowly rooted in the doping effect of PYM as a cross-linked bridge in CN frameworks via a multi-bonding mode, identifying with the above UV-vis DRS results. Therefore, the orbital hybridization and delocalization effects can effectively improve visible light absorption capacity and promote charge separation and transfer, thus boosting the PHE performance.
Moreover, for identifying the CB and VB positions of samples, the XPS VB spectra were obtained and the results are shown in Fig. 7a and b. The VB position of CN is located at 1.80 eV, while that of PYM100-CN goes up to 1.60 eV after introducing PYM into CN frameworks. Based on the above obtained band gap values, the CB positions of CN and PYM100-CN are all calculated to be −1.12 eV. It is obvious that the narrowed bandgap of PYM100-CN is attributed to the up-shift of VB. In addition, the Mott–Schottky plots are measured for two samples (Fig. 7c and d), in which both PYM100-CN and CN exhibit typical n-type semiconductor features due to their own positive slopes, and the smaller slope of the former indicates its higher electron density, electric conductivity and charge carrier mobility.46,47 We note that the flat-band potentials of CN and PYM100-CN are −0.58 eV and −0.62 eV, respectively, suggesting the upward shift of Fermi energy level (Ef). The narrowing of the bandgap and up-shift of Ef demonstrate that the electrons are more easily excited on PYM100-CN than CN, thus availing the PHE reaction.
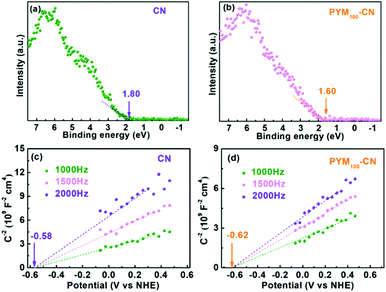 |
| Fig. 7 XPS VB spectra (a and b) and Mott–Schottky plots (c and d) of CN and PYM100-CN. | |
In addition, we investigated the specific surface area and pore size distribution characters by means of nitrogen adsorption and desorption isotherms (Fig. S2†). CN and PYM100-CN reveal an analogous isotherm hysteresis located between 0.4–1.0 (P/P0) with typical IV type, indicating the presence of mesopores, which is also proved by the pore size distribution around 60 nm. Meanwhile, the specific surface area of pure CN is 80.608 m2 g−1, which is slightly smaller than that of PYM100-CN (97.617 m2 g−1). The above results confirm that the introduction of PYM as a cross-linked bridge in CN frameworks has almost no effect on the relative surface area and pore size, indicating that they are not the main factors affecting the PHE activity.
It is well known that the effective separation of photoinduced carriers is an important factor for the improved photocatalytic performance. Generally, the photocurrent response is deemed as qualitative, questioning the separation efficiency of carriers during the photocatalytic reactions. As shown in Fig. 8a, CN and PYM100-CN have fast uniform photocurrent response under the visible light, in which the significantly enhanced photocurrent intensity of PYM100-CN is almost 8 times that of pure CN. It demonstrated the more effective separation and faster charge carriers for PYM100-CN than pure CN.46,47 At the same time, the photocurrent responses of PYM100-CN are clearly produced under the various monochromatic light irradiation (Fig. 8b), corresponding well to the absorption spectra and AQE values as per the above description. In addition, the higher separation efficiency of photogenic carriers over PYM100-CN is further indirectly confirmed by the photoluminescence (PL) spectra. In Fig. 8c, the pristine CN exhibits a strong emission peak located at 450 nm. In contrast, the peak of PYM100-CN is slightly red-shifted compared with pure CN, which is consistent with UV-Vis DRS. Besides, the PL emission intensity of PYM100-CN is significantly reduced, suggesting its lower recombination efficiency of charge carriers than pure CN.47,48 Furthermore, the time-resolved PL decay curves (Fig. 8d) exhibit a lower fluorescence lifetime of PYM100-CN (2.98 ns) than that of pure CN (6.59 ns), which indicates effective charge transfer and suppressed recombination of carriers via producing new nonradiative channels.47,48 More intuitively, the photographs of the CN and PYM100-CN powders or their suspensions under the ultraviolet light irradiation also confirm the PL quenching phenomenon (Fig. S3†).
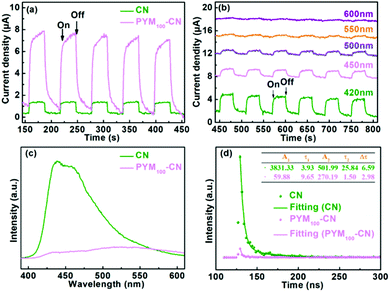 |
| Fig. 8 Transient photocurrent curves of CN and PYM100-CN (a); transient photocurrent curves of PYM100-CN at different wavelengths (b); PL spectra (c) and time-resolved PL decay curves (d) of CN and PYM100-CN. | |
Furthermore, the EIS was performed to study the charge transport resistance. Fig. 9a shows that the EIS Nyquist plots over PYM100-CN have a smaller arc radius than that of CN, which represents the faster interfacial electron transfer for the redox reaction.47,48 Moreover, the electrochemically active surface area (ECSA) has the positive linear relationship with double layer capacitance (Cdl). As shown in Fig. 9b, the Cdl values are calculated through cyclic voltammetry curves at different scan rates (Fig. S4†), in which PYM100-CN exhibits the higher 0.0308 mF cm−2 than that of CN (0.0241 mF cm−2), implying that the former can furnish more active sites than the latter in the process of hydrogen evolution reaction.49,50 In addition, the linear sweep voltammetry (LSV) of PYM100-CN was further measured and shown in Fig. 9c, where the overpotential of PYM100-CN (−0.74 V) is lower than that of CN (−0.91 V) under the current density of 10 mA cm−2. Meanwhile, on the basis of LSV curves, the fitted Tafel slope of PYM100-CN is 199 mV dec−1, which is much lower than that of CN (278 mV dec−1) (Fig. 9d), which reflects the more favorable reaction kinetics for PYM100-CN than CN in the hydrogen evolution process.51
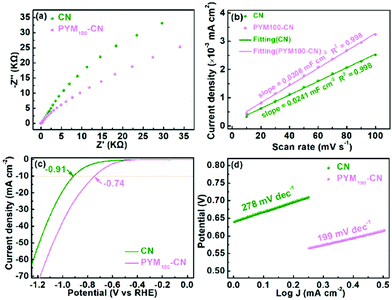 |
| Fig. 9 EIS Nyquist plots (a), capacitive currents at different scan rates (b); LSV curves (c) and corresponding Tafel plots (d) of CN and PYM100-CN. | |
Based on the above results, the band structure variation and possible PHE reaction mechanism are proposed. As shown in the schematic diagram (Fig. 10), the band position is effectively adjusted after incorporating PYM as a cross-linked bridge into the CN frameworks, which results in the up-shift of 0.20 V of VB and reduction of the band gap. At the same time, the doping effect of PYM via a multi-bonding mode also makes Ef of PYM100-CN slightly move up 0.04 V relative to pure CN, demonstrating the easier electron transfer from VB to CB in PYM100-CN than pure CN. More precisely, in the visible light irradiation, the VB electrons are excited to transfer to the CB to produce the photogenerated electron–hole pairs. In the meantime, the doping effect of PYM will propel the effective separation of photogenerated charge carriers in the plane of PYM100-CN frameworks. Therefore the photogenerated electrons immediately transfer to a Pt cocatalyst to react with H+/H2O along with releasing hydrogen. The photogenerated holes can be reduced by TEOA as a sacrifice agent constantly.
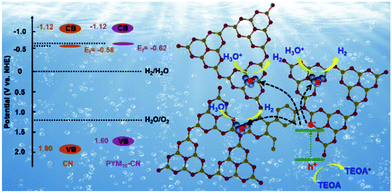 |
| Fig. 10 Band structure variation and possible PHE reaction mechanism of PYM-CN. | |
Conclusions
All in all, a new modified CN polymer photocatalyst with cross-linked structure is prepared via forming a multi-bonding mode between PYM and CN frameworks. A series of experimental investigations combined with DFT theoretical calculations reveal that the doping effect of PYM effectively broadens the visible-light absorption range and facilitates the transfer and separation of intermolecular electron–hole pairs by expanding π-conjugated channels, which stems from the orbital hybridization and delocalization effects owing to the unique intramolecular cross-linked structure in PYM-CN. Consequently, the photocatalytic performance of all PYM-CN samples is boosted dramatically. The optimal PYM100-CN presents a PHE rate of 5.418 mmol h−1 g−1, which is about 11.6 times higher than that of pristine CN and achieves an AQE value of 17.7% at 420 nm. Our work provides a deep insight into the exploitation of cross-linked CN with a multi-bonding mode for promoting the separation efficiency of intramolecular charge carriers in the application of converting solar energy into clean hydrogen fuel.
Conflicts of interest
The authors declare no conflict of interest.
Acknowledgements
This work was supported by the NSFC-Shanxi Coal Based Low Carbon Joint Fund (U1810117), the National Natural Science Foundation of China (52072153), and the Natural Science Foundation of Jiangsu Province (BK20190867).
References
- X. Tian, P. Zhao and W. Sheng, Hydrogen evolution and oxidation: mechanistic studies and material advances, Adv. Mater., 2019, 31, 1808066 CrossRef.
- J. Qi, W. Zhang and R. Cao, Solar-to-hydrogen energy conversion based on water splitting, Adv. Energy Mater., 2018, 8, 1701620 CrossRef.
- Z. Liang, R. Shen, Y. H. Ng, P. Zhang, Q. Xiang and X. Li, A review on 2D MoS2 cocatalysts in photocatalytic H2 production, J. Mater. Sci. Technol., 2020, 56, 89–121 CrossRef.
- L. Mao, X. Cai and M. Zhu, Hierarchically 1D CdS decorated on 2D perovskite-type La2Ti2O7 nanosheet hybrids with enhanced photocatalytic performance, Rare Met., 2021, 40, 1067–1076 CrossRef CAS.
- C. Li, D. Zhu, S. Cheng, Y. Zuo, Y. Wang, C. Ma and H. Dong, Recent research progress of bimetallic phosphides-based nanomaterials as cocatalyst for photocatalytic hydrogen evolution, Chin. Chem. Lett., 2021 DOI:10.1016/j.cclet.2021.07.057.
- H. Dong, M. Xiao, S. Yu, H. Wu, Y. Wang, J. Sun, G. Chen and C. Li, Insight into the activity and stability of RhxP nano-species supported on g-C3N4 for Photocatalytic H2 Production, ACS Catal., 2020, 10, 458–462 CrossRef CAS.
- R. Shen, Y. Ding, S. Li, P. Zhang, Q. Xiang, Y. H. Ng and X. Li, Constructing low-cost Ni3C/twin-crystal Zn0.5Cd0.5S heterojunction/homojunction nanohybrids for efficient photocatalytic H2 evolution, Chin. J. Catal., 2021, 42, 25–36 CrossRef CAS.
- E. Liu, X. Lin, Y. Hong, L. Yang, B. Luo, W. Shi and J. Shi, Rational copolymerization strategy engineered C self-doped g-C3N4 for efficient and robust solar photocatalytic H2 evolution, Renew. Energy, 2021, 178, 757–765 CrossRef CAS.
- L. Yan, J. Hou, T. Li, Y. Wang, C. Liu, T. Zhou, W. Jiang, D. Wang and G. Che, Tremella-like integrated carbon nitride with polyvinylimine-doped for enhancing photocatalytic degradation and hydrogen evolution performances, Sep. Purif. Technol., 2021, 279, 119766 CrossRef CAS.
- R. Shen, D. Ren, Y. Ding, Y. Guan, Y. H. Ng, P. Zhang and X. Li, Nanostructured CdS for efficient photocatalytic H2 evolution: A review, Sci. China Mater., 2020, 63, 2153–2188 CrossRef CAS.
- L. Wang, Y. Hong, E. Liu, X. Duan, X. Lin and J. Shi, A bottom-up acidification strategy engineered ultrathin g-C3N4 nanosheets towards boosting photocatalytic hydrogen evolution, Carbon, 2020, 163, 234–243 CrossRef CAS.
- Y. Yang, B. Mao, G. Gong, D. Li, Y. Liu, W. Cao, L. Xing, J. Zeng, W. Shi and S. Yuan,
In situ growth of Zn–AgIn5S8 quantum dots on g-C3N4 towards 0D/2D heterostructured photocatalysts with enhanced hydrogen production, Int. J. Hydrogen Energy, 2019, 44, 15882–15891 CrossRef CAS.
- Y. Xing, X. Wang, S. Hao, X. Zhang, X. Wang, W. Ma, G. Zhao and X. Xu, Recent advances in the improvement of g-C3N4 based photocatalytic materials, Chin. Chem. Lett., 2021, 32, 13–20 CrossRef CAS.
- J. Fu, J. Yu, C. Jiang and B. Cheng, g-C3N4-Based heterostructured photocatalysts, Adv. Energy Mater., 2018, 8, 1701503 CrossRef.
- X. Ruan, X. Cui, G. Jia, J. Wu, J. Zhao, D. J. Singh, Y. Liu, H. Zhang, L. Zhang and W. Zheng, Intramolecular heterostructured carbon nitride with heptazine-triazine for enhanced photocatalytic hydrogen evolution, Chem. Eng. J., 2022, 428, 132579 CrossRef CAS.
- Y. Hong, L. Wang, E. Liu, J. Chen, Z. Wang, S. Zhang, X. Lin, X. Duan and J. Shi, A curly architectured graphitic carbon nitride (g-C3N4) towards efficient visible-light photocatalytic H2 evolution, Inorg. Chem. Front., 2020, 7, 7347–7355 Search PubMed.
- J. Wu, C. Li, H. Dong, H. Zhang, J. Han, L. Wang, S. Yu and Y. Wang, Doping effect of metalloid group in graphitic carbon nitride molecular structure for significantly improved photocatalytic hydrogen production and photoelectric performance, Renew. Energy, 2020, 157, 660–669 CrossRef CAS.
- S. Tian, S. Chen, X. Ren, R. Cao, H. Hu and F. Bai, Bottom-up fabrication of graphitic carbon nitride nanosheets modified with porphyrin via covalent bonding for photocatalytic H2 evolution, Nano Res., 2019, 12, 3109–3115 CrossRef CAS.
- Y. Chen, Y. Qu, X. Zhou, D. Li, P. Xu and J. Sun, Phenyl-bridged graphitic carbon nitride with a porous and hollow sphere structure to enhance dissociation of photogenerated charge carriers and visible-light-driven H2 generation, ACS Appl. Mater. Interfaces, 2020, 12, 41527–41537 CrossRef CAS PubMed.
- Y. Guo, S. Chu, S. Yan, Y. Wang and Z. Zou, Developing a polymeric semiconductor photocatalyst with visible light response, Chem. Commun., 2010, 46, 7325–7327 RSC.
- S. Jhulki, J. Kim, I. Hwang, G. Haider, J. Park, J. Park, Y. Lee, W. Hwang, A. Dar, B. Dhara, S. Lee, J. Kim, J. Koo, M. Jo, C. Hwang, Y. Jung, Y. Park, M. Kataria, Y. Chen, S. Jhi, M. Baik, K. Baek and K. Kim, Solution-processable, crystalline π-conjugated two-dimensional polymers with high charge carrier, Chem, 2020, 6, 1–11 Search PubMed.
- K. Li, M. Sun and W. Zhang, Polycyclic aromatic compounds-modified graphitic carbon nitride for efficient visible-light-driven hydrogen evolution, Carbon, 2018, 134, 134–144 CrossRef CAS.
- C. Li, H. Wu, D. Zhu, T. Zhou, M. Yan, G. Chen, J. Sun, G. Dai, F. Ge and H. Dong, High-efficient charge separation driven directionally by pyridine rings grafted on carbon nitride edge for boosting photocatalytic hydrogen evolution, Appl. Catal., B, 2021, 297, 120433 CrossRef CAS.
- E. Suh, Y. Jeong, J. Oh, K. Lee, J. Jung, Y. Kang and J. Jang, Doping of donor-acceptor polymers with long side chains via solution mixing for advancing thermoelectric properties, Nano Energy, 2019, 58, 585–595 CrossRef CAS.
- W. Xing, G. Chen, C. Li, Z. Han, Y. Hu and Q. Meng, Doping effect of non-metal group in porous ultrathin g-C3N4 nanosheets towards synergistically improved photocatalytic hydrogen evolution, Nanoscale, 2018, 10, 5239–5245 RSC.
- G. Damas, F. Kieseritzky, J. Hellberg, C. Marchiori and C. Araujo, Symmetric small-molecules with acceptor−donor−acceptor architecture for efficient visible-light driven hydrogen production: optical and thermodynamic aspects, J. Phys. Chem. C, 2019, 123, 30799–30808 CrossRef CAS.
- K. Li and W. Zhang, Creating graphitic carbon nitride based donor-π-acceptor-π-donor structured catalysts for highly photocatalytic hydrogen evolution, Small, 2018, 14, 1703599 CrossRef PubMed.
- H. Che, C. Liu, G. Che, G. Liao, H. Dong, C. Li, N. Song and C. Li, Facile construction of porous intramolecular g-C3N4-based donor-acceptor conjugated copolymers as highly efficient photocatalysts for superior H2 evolution, Nano Energy, 2020, 67, 104273 CrossRef CAS.
- Y. Liu, Z. Liao, X. Ma and Z. Xiang, Ultrastable and efficient visible-light-driven hydrogen production based on donor−acceptor copolymerized covalent organic polymer, ACS Appl. Mater. Interfaces, 2018, 10, 30698–30705 CrossRef CAS PubMed.
- Z. Lan, W. Ren, X. Chen, Y. Zhang and X. Wang, Conjugated donor-acceptor polymer photocatalysts with electron-output “tentacles” for efficient hydrogen evolution, Appl. Catal., B, 2019, 245, 596–603 CrossRef CAS.
- C. Zhao, Z. Chen, R. Shi, X. Yang and T. Zhang, Recent advances in conjugated polymers for visible-light-driven water splitting, Adv. Mater., 2020, 32, 1907296 CrossRef CAS PubMed.
- V. Vyas, V. Lau and B. Lotsch, Soft photocatalysis: organic polymers for solar fuel production, Chem. Mater., 2016, 28, 5191–5204 CrossRef CAS.
- B. Delley, An all–electron numerical method for solving the local density functional for polyatomic molecules, J. Chem. Phys., 1990, 92, 508–517 CrossRef CAS.
- B. Delley, From molecules to solids with the DMol3 approach, J. Chem. Phys., 2000, 113, 7756–7764 CrossRef CAS.
- J. Perdew, K. Burke and M. Ernzerhof, Generalized gradient approximation made simple, Phys. Rev. Lett., 1996, 77, 3865–3868 CrossRef CAS.
- X. Lan, Q. Li, Y. Zhang, Q. Li, L. Ricardez-Sandoval and G. Bai, Engineering donor-acceptor conjugated organic polymers with boron nitride to enhance photocatalytic performance towards visible-light-driven metalfree selective oxidation of sulfides, Appl. Catal., B, 2020, 277, 119274 CrossRef CAS.
- W. Li, Z. Guo, L. Jiang, L. Zhong, G. Li, J. Zhang, K. Fan, S. Gonzalez-Cortes, K. Jin, C. Xu, T. Xiao and P. Edwards, Facile in situ reductive synthesis of both nitrogen deficient and protonated g-C3N4 nanosheets for the synergistic enhancement of visible-light H2 evolution, Chem. Sci., 2020, 11, 2716–2728 RSC.
- Y. Qin, H. Li, J. Lu, Y. Feng, F. Meng, C. Ma, Y. Yan and M. Meng, Synergy between van der waals heterojunction and vacancy in ZnIn2S4/g-C3N4 2D/2D photocatalysts for enhanced photocatalytic hydrogen evolution, Appl. Catal., B, 2020, 277, 119254 CrossRef CAS.
- C. Yang, B. Wang, L. Zhang, L. Yin and X. Wang, Synthesis of layered carbonitrides from biotic molecules for photoredox transformations, Angew. Chem., Int. Ed., 2017, 56, 1–6 CrossRef.
- H. Niu, Y. Liu, B. Mao, N. Xin, H. Jia and W. Shi,
In situ embedding MOFs-derived copper sulfide polyhedrons in carbon nanotube networks for hybrid supercapacitor with superior energy density, Electrochim. Acta, 2020, 329, 135130 CrossRef CAS.
- X. Zhang, S. He and S. Jiang, WOx/g-C3N4 layered heterostructures with controlled crystallinity towards superior photocatalytic degradation and H2 generation, Carbon, 2020, 156, 488–498 CrossRef CAS.
- H. Dong, S. Hong, P. Zhang, S. Yu, Y. Wang, S. Yuan, H. Li, J. Sun, G. Chen and C. Li, Metal-free Z-scheme 2D/2D VdW heterojunction for high-efficiency and durable photocatalytic H2 production, Chem. Eng. J., 2020, 395, 125150 CrossRef CAS.
- H. Li, H. Lee, G. Park, B. Lee, J. Park, C. Shin, W. Hou and J. Yu, Conjugated polyene-functionalized graphitic carbon nitride with enhanced photocatalytic water-splitting efficiency, Carbon, 2018, 129, 637–645 CrossRef CAS.
- H. Wang, Y. Wu, M. Feng, W. Tu, T. Xiao, T. Xiong, H. Ang, X. Yuan and J. W. Chew, Visible-light-driven removal of tetracycline antibiotics and reclamation of hydrogen energy from natural water matrices and wastewater by polymeric carbon nitride foam, Water Res., 2018, 144, 215–225 CrossRef CAS PubMed.
- H. Dong, X. Zhang, J. Li, P. Zhou, S. Yu, N. Song, C. Liu, G. Che and C. Li, Construction of morphology-controlled nonmetal 2D/3D homojunction towards enhancing photocatalytic activity and mechanism insight, Appl. Catal., B, 2020, 263, 118270 CrossRef CAS.
- H. Dong, Y. Zuo, N. Song, S. Hong, M. Xiao, D. Zhu, J. Sun, G. Chen and C. Li, Bimetallic synergetic regulating effect on electronic structure in cobalt/vanadium co-doped carbon nitride for boosting photocatalytic performance, Appl. Catal., B, 2021, 287, 119954 CrossRef CAS.
- X. Peng, J. Wu, Z. Zhao, X. Wang, H. Dai, L. Xu, G. Xu, Y. Jian and F. Hu, Activation of peroxymonosulfate by single atom Fe-g-C3N4 catalysts for high efficiency degradation of tetracycline via non-radical pathways: Role of high-valent iron-oxo species and Fe-Nx sites, Chem. Eng. J., 2022, 427, 130803 CrossRef CAS.
- H. Dong, N. Song, M. Yan, H. Wu, H. Zhang, C. Ma and Y. Wang, Fabrication of HRP/Bi2WO6 photoenzyme-coupled artificial catalytic system for efficiently degrading bisphenol A, Chin. Chem. Lett., 2021, 32, 2047–2051 CrossRef CAS.
- J. Lin, P. Wang, H. Wang, C. Li, X. Si, J. Qi, J. Cao, Z. Zhong, W. Fei and J. Feng, Defect-rich heterogeneous MoS2/NiS2 nanosheets electrocatalysts for efficient overall water splitting, Adv. Sci., 2019, 6, 1900246 CrossRef PubMed.
- J. Wang, H. Zhong, Z. Wang, F. Meng and X. Zhang, Integrated three-dimensional carbon paper/carbon tubes/cobalt-sulfide sheets as an efficient electrode for overall water splitting, ACS Nano, 2016, 10, 2342–2348 CrossRef CAS PubMed.
- N. Song, S. Hong, M. Xiao, Y. Zuo, E. Jiang, C. Li and H. Dong, Fabrication of Co(Ni)-P surface bonding states on core-shell Co(OH)2@P-NiCo-LDH towards electrocatalytic hydrogen evolution reaction, J. Colloid Interface Sci., 2021, 582, 535–542 CrossRef CAS PubMed.
Footnote |
† Electronic supplementary information (ESI) available. See DOI: 10.1039/d1qi01122g |
|
This journal is © the Partner Organisations 2022 |
Click here to see how this site uses Cookies. View our privacy policy here.