DOI:
10.1039/C8RA03620A
(Review Article)
RSC Adv., 2018,
8, 23780-23804
Current progress, challenges and future prospects of diagnostic and therapeutic interventions in Alzheimer's disease
Received
26th April 2018
, Accepted 4th June 2018
First published on 29th June 2018
Abstract
Alzheimer's disease (AD) is the most prevalent, progressive and multifaceted neurodegenerative disorder associated with cognition, memory and behavioural impairments. There is no approved diagnosis or cure for AD, and it affects both developed and developing countries and causes a significant social and economic burden. Extracellular senile plaques of amyloid beta (Aβ) and intracellular neurofibrillary tangles of phosphorylated Tau (pTau) in the brain are considered to be the pathophysiological hallmarks of AD. In an attempt to explain the complexity and multifactorial nature of AD, various hypotheses (Aβ aggregation, Tau aggregation, metal dyshomeostasis, oxidative stress, cholinergic dysfunction, inflammation and downregulation of autophagy) based on pathophysiological changes that occur during the onset and progression of AD have been proposed. However, none of the hypotheses is capable of independently explaining the pathological conditions observed in AD. The complex and multifaceted pathophysiological nature of AD has hampered the identification and validation of effective biomarkers for early diagnosis and the development of disease-modifying therapies. Nevertheless, the amyloid hypothesis is the most widely accepted and is closely correlated with disease symptoms of AD that encompass all the disease hypotheses. Therefore, amyloid plaques are ideal biomarkers for the development of an early diagnosis of AD. Similarly, the formation of amyloid plaques can also serve as a target for the design of therapeutic tools via an inclusive approach that considers multiple disease pathways involved in AD. Our review article briefly introduces pathophysiological factors involved in AD using interdependent but diverse hypotheses. Recent advances in the development of effective molecular tools and techniques for diagnostic and therapeutic interventions in AD, especially those in the advanced stages (clinical trials) of development, are given special consideration. In addition, contributions from our laboratory to the development of selective molecular tools for diagnostic and therapeutic interventions that target multifaceted toxicity in AD are also covered. In summary, we discuss diverse aspects of molecular mechanisms that underlie the pathogenesis of multifactorial AD, current progress and possible bottlenecks that have hampered the development of early diagnostic tools and effective drugs. Challenges and future prospects include the integration of various disease pathways for the successful development of an early diagnosis and effective drugs for the treatment of AD.
1. Introduction
AD is the most devastating form of all neurodegenerative disorders and contributes to about 70–80% of all cases of dementia.1 Recent statistics reveal that >45 million people are affected by AD, and this number is expected to rise threefold to >135 million by 2050. Alarmingly, there is no approved diagnosis or cure for AD, and the development of effective diagnostic and therapeutic tools has been hindered by its multifactorial nature.2–4 AD is characterised by the accumulation of two kinds of toxic protein aggregates, namely, extracellular amyloid plaques and intracellular neurofibrillary tangles (NFTs)/paired helical filaments (PHFs) in the brain (Fig. 1a). The typical symptoms of AD patients include a decline in cognition, memory loss, a lack of task-performing ability and behavioural discrepancies. In the next decade, it is predicted that AD will severely affect both developed and developing countries and cause a significant social and economic burden (Fig. 1a). Whereas all major diseases such as prostate and breast cancer, heart diseases, and AIDS have undergone a decline, the number of patients affected by AD has risen by >71% (Fig. 1a).5,6 The continuous and rapid rise in disease pathogenesis is due to the absence of early detection methods and medication that can either slow or prevent progression of the disease. As a consequence, the scientific community is devoting immense efforts to understanding the pathways and progression of AD to facilitate the development of effective treatments. The exact mechanism of AD pathogenesis is not understood owing to multiple disease pathways (multifactorial nature). However, the presence of extracellular senile plaques and intracellular NFTs/PHFs in the brain in AD has been shown to play a key role in all disease pathogenesis.2,7 The amyloid hypothesis is the most widely accepted and is reasonably closely correlated with the disease symptoms observed during AD and related multiple disease pathways involving Tau hyperphosphorylation, inflammation, post-translational modifications, protein imbalances, and dysfunction of the cholinergic system, among others. Therefore, amyloid aggregates (senile plaques and NFTs) are ideal biomarkers for the development of early diagnostic and therapeutic interventions in AD.8–10 Recent advances in the development of effective diagnostic and therapeutic tools, especially those in the advanced stages of development, and contributions from our laboratory are covered with respect to current progress, challenges and future prospects. There is a vast literature on AD, and the reader is directed to review articles published elsewhere for reports that do not fall within the scope of this article.
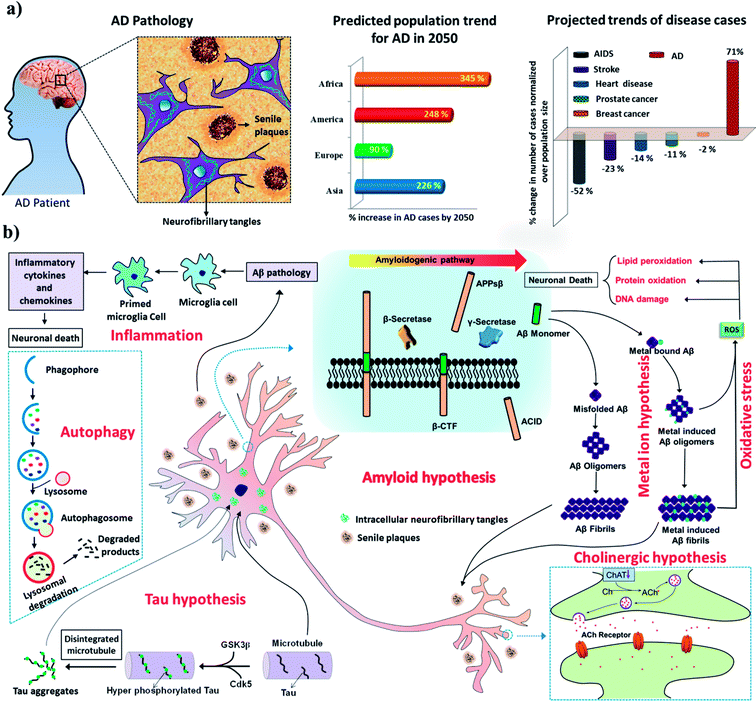 |
| Fig. 1 (a) Characteristic features of the pathology of AD in the human brain and recent statistics showing the dramatic and rapid rise of AD as a global epidemic. (b) Multiple pathological pathways of AD. | |
2. AD pathology
The multifactorial nature of AD has led to the proposal of various hypotheses based on the molecular and pathophysiological changes observed in the brain during the onset and progression of AD (Fig. 1B). We present some of the important hypotheses in the following sections.
2.1 Amyloid hypothesis
The amyloid hypothesis postulates that the generation, misfolding, aggregation and deposition of Aβ peptides as senile plaques in the brain lead to neurodegeneration, which is considered to be one of the main causative factors of AD (Fig. 1b).11 The aggregation of Aβ is thought to be the core of the pathology of AD and acts as a trigger for various other closely associated pathological pathways such as Tau phosphorylation, metal dyshomeostasis, mitochondrial damage, the generation of reactive oxygen species (ROS), oxidative stress, and inflammation, among others, which contribute to neuronal toxicity and cell death. Aβ peptides are derived from amyloid precursor protein (APP), which is a transmembrane neuronal glycoprotein, by the action of protease enzymes known as secretases. Under normal physiological conditions, APP is cleaved by α-secretase and γ-secretase and generates nontoxic fragments, which are cleared from the brain. However, adverse pathophysiological conditions alter the mode of processing of APP, as it is cleaved by β-secretase and γ-secretase to produce Aβ peptide fragments (37–43 amino acids). These Aβ peptide fragments, especially Aβ42, are highly amyloidogenic and undergo misfolding to form a β-sheet structure and hydrophobic interactions to form toxic oligomers, protofibrils, fibrils and senile plaques in extracellular regions of the brain (Fig. 1b). Among aggregated Aβ species, oligomers are considered to be the most toxic forms in comparison with protofibrils and fully grown fibrils. However, recent studies have shown that fully grown fibrils or aggregates serve as a catalytic surface to produce highly toxic oligomeric species via a secondary mechanism.2,12 Aβ oligomers interfere with a signalling cascade in the synaptic cleft, which leads to synaptic dysfunction and neuronal death. For instance, Aβ oligomers bind to glutamate receptors [NMDA receptor (N-methyl-D-aspartate receptor) and AMPA receptor (α-amino-3-hydroxy-5-methyl-4-isoxazolepropionic acid receptor)] and instigate the loss of synaptic plasticity and memory formation. In general, the amyloid hypothesis is the most widely studied and validated hypothesis for understanding the underlying mechanism of the pathogenesis and progression of AD. Moreover, various observations in cell biology and genetics have confirmed that Aβ plays a vital role in the pathology of AD.13
2.2 Tau hypothesis
Tau is a microtubule-associated protein (MAP), and its function is to modulate the stability of microtubules.14 Tau protein is predominantly found in the axons of neurons and is associated with axonal microtubules.15 The uncontrolled phosphorylation of Tau leads to the disintegration of microtubules and the subsequent intracellular aggregation of pTau to form NFTs and is the pathological basis of the Tau hypothesis of AD (Fig. 1b).16 Notably, the phosphorylation of Tau is a dynamic process and has biological significance for the structural stability and function of neurons. However, under disease conditions the specific phosphorylation of Tau becomes irreversible and leads to hyperphosphorylation, which triggers its collapse and intracellular aggregation to form NFTs. There are sufficient reports to conclude that Tau plays a definitive role in the pathology of AD. However, the Tau hypothesis alone is inadequate to explain all the symptomatic conditions observed in AD.17 Evidently, the amyloid and Tau hypotheses are closely linked to each other and together play a vital role in the pathology of AD. Clearly, there is a controversy over the identification of the first of these two hypotheses that initiates the pathology of AD, as there exist reports that support the theory that Aβ triggers Tau pathology and vice versa.11 However, recent studies point to the fact that the aggregation of Aβ and associated events trigger Tau pathogenesis.17,18 Nevertheless, Tau aggregates are also observed in relatively early stages of AD and are possibly potent biomarkers for early diagnostic and therapeutic targets for AD. Various therapeutic strategies that have been adopted to test the Tau hypothesis include (i) the inhibition of Tau phosphorylation or phosphorylating enzymes [glycogen synthase kinase-3β (GSK3β), cyclin-dependent kinase-5 (Cdk5), protein kinase A (PKA) and Ca2+/calmodulin-dependent protein kinase II (CaMKII)], (ii) the inhibition of Tau aggregation and improvement of its clearance, and (iii) the stabilisation of microtubules.19 Some of the lead candidates developed for AD as a result of these therapeutic strategies are at various stages of clinical trials and are discussed in the therapeutics section.
2.3 Metal hypothesis
The presence of high concentrations of metal ions (Cu, Fe, Zn and Al) in senile plaques indicates their possible role in the pathology of AD (Fig. 1b).20–23 Metal ion dyshomeostasis is a common phenomenon observed during AD pathogenesis.24 Copper and zinc ions are known to accelerate the aggregation of Aβ to form senile plaques.25 Redox-active metals, especially copper and iron, stabilize oligomeric forms of Aβ peptides. These oligomeric species are reported to interact with various synaptic receptors and cause signalling dysfunction.26 Moreover, Aβ-bound redox-active metals (Cu and Fe) undergo redox cycling in a reducing environment to generate excess ROS, which further result in oxidative stress and cause neuronal death. The obvious therapeutic intervention in accordance with the metal hypothesis would be the development of metal chelators that target Aβ-bound redox-active metals and maintain them in a redox-dormant state. However, testing the metal hypothesis using metal chelators has not been entirely successful, which was attributed to the multifaceted toxicity observed in AD.27 In recent times, the development of multifunctional molecules that target metal sequestration together with other pathological pathways (amyloid aggregation, oxidative stress, mitochondrial dysfunction and many others) has received greater interest, as such strategies are reported to yield good results.
2.4 ROS and oxidative stress hypothesis
The generation and tightly controlled homeostasis of ROS and reactive nitrogen species (RNS) is a common physiological process and plays a pivotal role in cell signalling and the fight against infections.28 However, the production of excess ROS and RNS causes oxidative stress and damage to cellular biomacromolecules, which severely affects normal physiological functions and causes neuronal cell death.29 The brain is highly vulnerable to oxidative stress owing to its high oxygen consumption rates in comparison with other organs in the body. Elevated levels of ROS are generally observed in pathophysiological conditions in AD, and various pathways are responsible for inducing such highly unfavorable cellular conditions. For instance, Aβ peptides chelate with redox-active metals (Cu and Fe) to form Aβ-metal complexes, which initiate redox cycling in a reducing environment and become the source of the continuous generation and accumulation of ROS (Fig. 1b). Mitochondrial dysfunction is a key consequence observed in the pathology of AD, and together with mitochondrial damage initiates the cascade of pathways that generate ROS.30 The use of natural antioxidants that are generally found in many foods and medicinal plants, such as curcumin, resveratrol, epigallocatechin-3-gallate (EGCG), brazilin, orcein, tanshinone and many others, has been shown to provide significant relief to AD patients by preventing the generation of excess ROS and related oxidative stress.31
2.5 Cholinergic hypothesis
The cholinergic hypothesis refers to dysfunction of the cholinergic system at the synaptic overlap of neurons in the brain, which triggers memory loss and subsequent cognitive impairment.32 Therefore, rejuvenation of the cholinergic system is essential to reduce the risk of cognitive dysfunction and improve the memory of the patient. Choline acetyltransferase (ChAT) and acetylcholinesterase (AChE) are the key enzymes in the cholinergic system and play critical roles in neural transmission. Acetylcholine (ACh) is a key neurotransmitter synthesised from acetyl-CoA and choline, which is catalysed by ChAT. ACh receptors (AChR) in the synaptic cleft bind to ACh and process neuronal signals. AChE that is present in the synaptic cleft terminates signal transmission by hydrolysing ACh to form acetic acid and choline. The production and breakdown of the neurotransmitter ACh, which involve ChAT and AChE, respectively, operate via a feedback loop mechanism.33 A steep decline in levels of ACh and ChAT is observed during the pathology of AD and is responsible for memory loss and cognitive dysfunction (Fig. 1b). Therefore, the modulation of ChAT activity to prevent the degradation of ACh can restore normal synaptic signalling and cognitive function. Similarly, the NMDA receptor is a glutamate receptor and plays a vital role in synaptic signalling and synaptic plasticity.34 In fact, excessive NMDA activity causes excitotoxicity and neuronal cell death. The activation of synaptic NMDA initiates synaptic plasticity and is neuroprotective, whereas the activation of extrasynaptic NMDA causes a decline in mitochondrial membrane potential and neuronal death.35 The development of an NMDA antagonist that could selectively block extrasynaptic NMDA would be an ideal disease-modifying therapeutic strategy. Glutamate has not been mentioned, but, like a decrease in ACh levels, an increase in glutamate levels causes excitotoxicity.
2.6 Inflammation hypothesis
Inflammation is the natural defence mechanism in living systems, whereby the immune system recognises pathogens, debris, damaged and misfolded proteins, and damaged cells and initiates their degradation and clearance. Under AD conditions, amyloid deposits (senile plaques and neurofibrillary tangles) and damaged or dead neurons activate microglia to initiate inflammation.36 Microglia are activated to form primed microglia, which release cytokines, acute-phase reactants, and other inflammatory mediators that cause neuronal death (Fig. 1b).37 Activation of the inflammatory system subsequently causes localised damage in the brain, which contributes to neuronal death. In vivo models have indicated that inflammation significantly contributes to and accelerates the pathogenesis of AD; moreover, targeting AD using anti-inflammatory drugs has shown promising results in preliminary studies. However, clinical studies of anti-inflammatory drugs for treating AD are still in their infancy.38 Nevertheless, the effect of anti-inflammatory drugs has been evaluated, and the results show significant improvements in slowing the pathology of AD.39
2.7 Autophagy
Autophagy is the natural cellular mechanism of the degradation and recycling of toxic debris and cellular organelles.40 In fact, the modulation of autophagy plays a key role in many disease conditions, such as AD, cancer, Parkinson's disease, osteoarthritis, cardiac diseases and many others. Recently, Yoshinori Ohsumi was awarded the Nobel Prize (2016) in medicine for his contributions to understanding the mechanism of autophagy, which signifies the importance of studying autophagy in biological systems and disease research. In brief, in the autophagy process a small double-membrane sac initially appears and then elongates to form a phagophore, which engulfs part of the cytoplasm containing damaged proteins or dysfunctional organelles and is closed up by the fusion of the two ends of the membrane to form an autophagosome (Fig. 1b). The autophagosome fuses with lysosomes (autolysosome), which results in proteolytic degradation of the inner membrane and trapped contents. Finally, the autolysosome ruptures to release the degraded contents into the cytoplasm. Literature reports are in good agreement that dysfunction of autophagy is likely to contribute significantly to the onset and progression of AD.41 Dysregulation of autophagy impairs neuronal and nutrient homeostasis, which in turn contributes to pathogenic processes in AD. Defects in autophagy occur in the early stage of AD pathogenesis, and therefore the development of activators of autophagy is an effective strategy for the clearance of toxic peptide or protein aggregates (Aβ and Tau).42
3. Diagnostic intervention
The timely diagnosis of AD is the most important requirement and is critical for understanding the severity of the disease condition and providing appropriate treatment to the patient. As was mentioned previously, there are no approved methods for the diagnosis of AD, which in turn affects the design and development of therapeutic modalities. The current diagnosis of AD is mostly based on the assessment of the behaviour and cognitive state of patients. Cognitive deficits are observed only in the advanced stage of the disease, and irreversible damage has already occurred in the brain of the patient. Studies suggest that amyloid deposits and changes symptomatic of the disease possibly develop decades before a noticeable decline in cognitive function.43 Therefore, the development of molecular tools and techniques for early diagnosis has been the prime focus of research into AD. In this context, Aβ peptides, polymorphic aggregated Aβ species, hyperphosphorylated Tau, Tau aggregates, neurogranin, APP and monocyte chemoattractant protein-1 (MCP-1) in the brain, cerebrospinal fluid (CSF) and blood are considered to be potential biomarkers for early diagnosis (Fig. 2). Currently, a great amount of resources and research efforts are directed at the development of molecular tools and techniques for the identification and quantification of these biomarkers.44
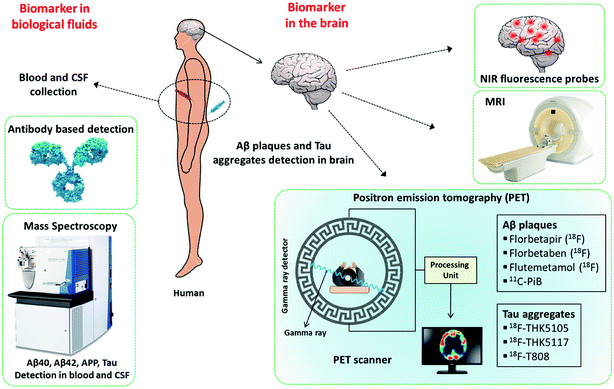 |
| Fig. 2 Molecular tools and techniques available for the detection of biomarkers in AD. | |
3.1 Far-red fluorescent probes
It is widely accepted that the presence of Aβ plaques precedes the clinical symptoms of AD. Furthermore, the amyloid hypothesis plays a key role in all other disease hypotheses that have been put forward for AD. Therefore, targeting Aβ species as biomarkers is an ideal strategy for developing tools and methods for the early diagnosis of AD (Fig. 2). Fluorescent probes provide a real-time, non-radioactive, high-resolution and inexpensive imaging technique for detecting Aβ species in the brain. In particular, far-red fluorescent probes are a special class of probes that are useful for the detection and imaging of Aβ species and have distinct advantages over normal probes. Red and near-infrared (NIR) molecular probes with emissions in the region of 650–800 nm are highly sought-after tools owing to their excitation by visible wavelengths, minimal interference from autofluorescence and scattering by biomolecules, and the advantages of a great sample penetration depth and enhanced sensitivity. These important and valuable attributes make them viable probes for developing detection and imaging tools for AD. In recent years, derivatives of oxazine, BODIPY, curcumin, styryl, stilbene, thiophenes and benzothiazole have been developed and extensively used as fluorescent probes for Aβ and Tau aggregates.45–48 A dual-response probe, namely, benzothiazole-coumarin (TC), was developed by our group for the detection of Aβ42 aggregates.49 TC exhibited turn-on red fluorescence (λex = 537 nm, λem = 654 nm) with a large Stokes shift (∼117 nm) and a characteristic colourimetric response upon binding to Aβ42 aggregates (Fig. 3a and b). Although the TC probe displayed high sensitivity and specificity towards Aβ42 aggregates over other abnormal protein aggregates, namely, α-synuclein (α-Syn, Parkinson's disease) and islet amyloid polypeptide (IAPP, type II diabetes), the probe was found to bind DNA. In the presence of both Aβ42 fibrillar aggregates and DNA, TC preferentially binds to Aβ42 fibrillar aggregates over DNA, which is attributed to the high binding affinity of TC for Aβ42 fibrillar aggregates.
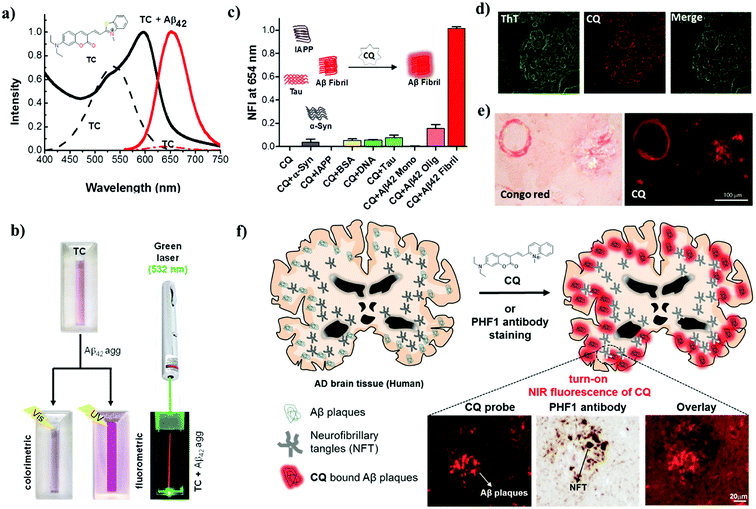 |
| Fig. 3 Red and NIR (far-red) fluorescent probes for diagnosis of AD. (a) Absorption and emission (λex = 537 nm) spectra of the probe TC in the presence and absence (dotted lines) of Aβ42 fibrillar aggregates. Inset: molecular structure of TC. (b) Photographs of TC and samples of TC + Aβ42 fibrillar aggregates illuminated with visible and UV light (365 nm) and TC + Aβ42 fibrillar aggregates illuminated with a green laser (532 nm), which gives a red beam in the sample solution. (c) Normalized fluorescence intensity (NFI) of CQ upon interaction with various protein aggregates, biomacromolecules and polymorphic Aβ species. (d) Dual staining of human brain cross-sections using CQ and ThT (100 nM CQ: 1.57 mM thioflavin T). (e) CQ stains Aβ plaques and congophilic angiopathy in human brain tissue with Congo red as control. (f) Molecular structure of the probe CQ and selective staining of Aβ plaques in human brain tissue. CQ selectively stains Aβ plaques in brain tissue in AD even in the presence of NFTs of Tau. This figure has been adapted from ref. 49 and 50 with permission from Nature Publishing Group and Elsevier, respectively. | |
The β-sheet structure is the common structural conformation observed among most amyloid aggregates, which has hindered the development of probes for the selective and specific detection of any individual protein aggregate. In fact, numerous fluorescent probes have been reported for Aβ aggregates, Aβ polymorphs, Tau aggregates and NFTs. However, most of these probes lack selectivity towards a specific protein aggregate and therefore cannot be developed into diagnostic tools for the detection of AD. This underlines the need for the development of selective probes for specific protein aggregates to accomplish a definitive diagnosis of AD. Hyperphosphorylated Tau and NFTs are signatures of tauopathies (frontotemporal dementia and supranuclear palsy, among others), which involve a breakdown of the normal functionality of Tau protein and are responsible for causing dementia independently of AD. The differential diagnosis of AD from tauopathies, other neurodegenerative diseases and, in particular, cases of mixed dementia demands the specific detection of Aβ species. We have developed a unique turn-on NIR fluorescent probe (CQ) by subjecting the TC probe to a rigorous structure–activity study for the detection of Aβ42 aggregates over Tau and other protein aggregates (Fig. 3).50 Coumarin-quinoline (CQ, λex = 521 nm, λem = 654 nm) exhibited remarkable fluorescence enhancement, binding affinity (82 nM), quantum yield (0.36) and selectivity towards Aβ42 aggregates. The selectivity of the probe was further confirmed by insignificant interference from the presence of various biomacromolecules (human serum albumin and DNA) and other toxic protein aggregates of Tau, α-Syn and IAPP (Fig. 3c). The differential detection of Aβ42 aggregates from Tau and other protein aggregates is vital in the clinical differentiation of AD from Tau pathology and cases of mixed dementia.
Furthermore, the specificity and sensitivity of CQ towards Aβ plaques were assessed in brain tissue from an AD patient. CQ efficiently stained Aβ plaques with a high signal-to-noise ratio and required a concentration that was lower by a factor of 15
700 to stain Aβ plaques in comparison with the control dyes thioflavin T (ThT) and thioflavin S (ThS) (Fig. 3d). Interestingly, various forms of Aβ plaques such as amyloid angiopathy and core/diffuse plaques were observed (Fig. 3e). The specific staining of Aβ plaques in AD brain tissue in the presence of coexisting Tau aggregates/NFTs is a remarkable property that is useful for developing specific tools for the clinical diagnosis of AD and differentiating it from Tau pathology (Fig. 3f). The utility of NIR probes for the in vitro detection, imaging and monitoring of Aβ aggregates has been quite successful. However, in vivo NIR fluorescence imaging of the human brain is limited by the current scope of fluorescence imaging techniques. This limitation of fluorescent probes for in vivo imaging of the human brain has led to the study of radiolabeling of fluorescent probes for positron emission tomography (PET) and single-photon emission computed tomography (SPECT) imaging, which are well-established techniques for application in humans. The NIR probe CQ, which exhibits good tissue penetration depths and blood–brain barrier (BBB) permeability, is a viable tool for academic/medical/disease research, monitoring disease progression, and in cellulo, in vitro (tissues) and in vivo imaging in animal models. The efficient BBB permeability and high sensitivity and selectivity of CQ towards Aβ plaques make it an ideal candidate for development as a PET probe, and currently work is in progress in our laboratory.
3.2 PET and SPECT
The pathological markers Aβ plaques and NFTs form in the brain decades before cognitive symptoms are manifested in an AD patient. Therefore, the in vivo imaging of Aβ plaques or NFTs in the human brain is a current requirement for the early diagnosis of AD pathology, and PET is considered to be a potential technique that may serve this purpose (Fig. 2).51 In this context, 11C-labelled Pittsburgh compound B (11C-PiB), which is a radioactive analogue of ThT, was one of the first tracers used to image Aβ plaques in the brain. However, 11C-PiB suffers from the very short half-life of 11C (20.33 min) (Fig. 4).52 Flutemetamol (18F), which is a derivative of PiB labelled with 18F, exhibited improved longevity for imaging, which was attributed to the longer half-life of 18F (109.7 min). Flutemetamol is approved by the FDA for in vivo PET imaging of Aβ plaques in the brain. Florbetapir (18F) and florbetaben (18F) are two other PET tracer analogues from the PiB family that are approved for in vivo imaging of amyloid plaques in the brain in AD (Fig. 4).53,54 Similarly, several PET probes have been developed for imaging Tau aggregates involved in AD and tauopathies.55 However, these probes are not approved for clinical imaging of Tau aggregates in the brain (Fig. 4). The quinolone derivatives 18F-THK5105 and 18F-THK5117 were developed for imaging Tau aggregates in AD patients. The retention of these probes was found to be good in regions of Tau deposits in the brain.56,57 The benzimidazole derivative 18F-T808 is a PET probe that has been reported for the detection of hyperphosphorylated Tau over other forms of Tau aggregates. Data showed a good kinetic profile and retention time for this probe in the brain, which differentiate AD patients from healthy controls.58 Similarly to PET probes, SPECT probes were also developed to study and detect amyloid aggregates in the brain.59 SPECT is more convenient than PET with respect to the number of facilities available and its use of radionuclides with longer half-lives. However, SPECT suffers from lower sensitivity and spatial resolution in comparison with PET imaging. Among the SPECT probes used for imaging amyloid, 123I-IMPY has shown promising results because it preferentially binds to Aβ plaques in the brain.60 Currently, PET-based imaging is the only technique available for the early clinical diagnosis of AD. However, this method involves radioactive tracers and requires expensive and sophisticated instrumentation, and affordability issues have hindered the widespread use of PET imaging for the diagnosis of AD. Moreover, the entire range of PET probes (based on ThT, which is a non-selective probe for the detection and staining of protein aggregates), whether approved or undergoing clinical trials, lack complete selectivity towards their target protein aggregates, which may lead to incorrect diagnosis and treatment. Non-selective staining becomes a severe problem when using PET probes in the case of patients with mixed dementia. Therefore, there is an urgent need to develop selective PET probes for both Aβ plaques and Tau aggregates/NFTs.
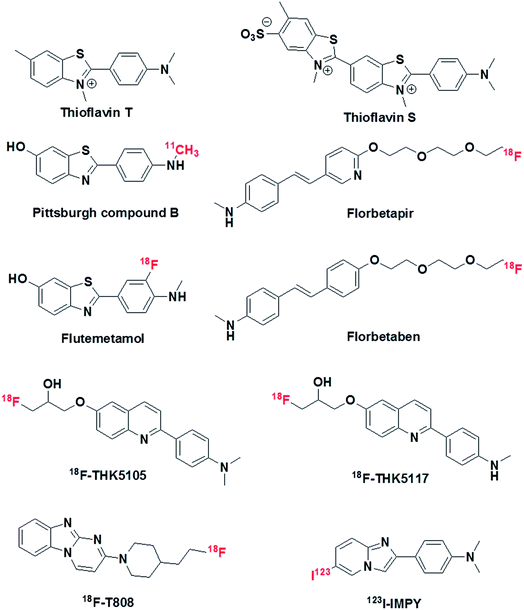 |
| Fig. 4 Molecular structures of thioflavin T, thioflavin S, PET probes and SPECT probe for the detection and imaging of Aβ and Tau aggregates. | |
3.3 Magnetic resonance imaging
Magnetic resonance imaging (MRI) is a non-invasive method for studying both structural and functional features of the brain.61 There are two types of MRI techniques, namely, structural imaging (sMRI) and functional imaging (fMRI). sMRI is used to study atrophy of the brain and is not a suitable diagnostic tool for AD. Atrophy that is observed may not be specific to AD and can be associated with any form of neuronal disorder. This lack of specificity also limits the utility of sMRI for the detection and imaging of histopathological hallmarks of AD such as senile plaques and NFTs. fMRI is employed to study the functional integrity of the brain, which includes memory formation, cognitive changes over time, and neuronal activity. fMRI studies have shown significant differences in the brain's complex network during aging, mild cognitive impairment (MCI), and AD. Moreover, fMRI revealed changes in functional connectivity during the progression of AD. This suggests that monitoring changes in functional connectivity using fMRI could be a promising biomarker for AD. However, work in this field is at a very early stage and needs to be standardized in multiple patients for the successful application of fMRI as a diagnostic tool for AD.62 Nevertheless, combining the power of MRI and PET imaging using selective and sensitive molecular probes may possibly deliver better tools for the early diagnosis of AD.
3.4 Mass spectrometry
The detection of biomarkers in the brain is an exceptionally challenging task and demands sophisticated, safe and practically viable techniques. On the other hand, the development and validation of tools and methods for the identification of biomarkers in biological fluids such as blood and CSF is appealing owing to the ease of accessibility of samples and requires the immediate attention of researchers and clinicians.63 Numerous techniques, such as ELISA, DNA-based probes, and antibodies, have been reported in the literature for the detection of biomarkers in blood and CSF; however, these techniques suffer from some common as well as specific limitations.64 CSF is a colourless fluid that is produced in the choroid plexus, which fills and circulates in the ventricular system, cisterns, sulci and the central canal of the spinal cord.65 It is believed that any biochemical changes and alterations in the levels of biomolecular markers that occur in the brain must be reflected in CSF. As a result, CSF has been used for the detection of Aβ and Tau aggregates and their concentrations for the early diagnosis of AD. Unlike blood, CSF is drawn from the spine by a procedure called lumbar puncture, which is painful and can cause adverse side effects in patients.65 Therefore, the use of blood samples for identifying biomarkers has several advantages and has received interest for the diagnosis of AD.66 The concentrations of Aβ species and Tau in blood (plasma and serum) are much lower in comparison with their levels in CSF. This bottleneck necessitates the development of ultrasensitive techniques for the analysis of biomarkers in blood samples. The use of highly sensitive mass spectrometry (MS) for the detection of biomarkers in blood samples is a promising technique. A number of biomolecules, including APP, Aβ species, Tau, pTau, apolipoprotein E (ApoE), interleukins (IL-1α, IL-6), clusterin (CLU) and α-1-antichymotrypsin (α-ACT), among others, have been considered as potential biomarkers, and the assessment of their precise concentrations in blood is anticipated to provide a reliable diagnostic method for AD.67 In particular, Aβ peptides and their concentrations in plasma have been extensively investigated as biomarkers for AD. However, it is unclear to what extent Aβ levels in blood samples can serve as a precise diagnostic indicator of AD. Several studies have indicated that the mere presence of Aβ40 and Aβ42 in a blood sample is not correlated with pathological conditions in AD.68 The majority of reports have confirmed the fact that the Aβ42/Aβ40 concentration ratio in blood samples has greater significance for the diagnosis of AD in comparison with the quantification of individual species. In a recent study, Yanagisawa et al. used immunoprecipitation coupled with mass spectrometry for the definitive detection of biomarkers in blood that are correlated with amyloid deposits in the brain.69 A composite parameter was derived from the APP/Aβ42 and Aβ40/Aβ42 ratios to define the Aβ-positive or -negative status of the brain, and the results were validated via PET imaging of the brain. In this study two independent datasets were obtained from Japan (n = 121) and Australia (n = 252), which included normal individuals, individuals with MCI and individuals with AD. Composite biomarker data obtained by blood analysis have been used to determine the Aβ plaque load in the brain with an accuracy of 90% and statistical significance. This MS-based technique appears to be promising; however, much more standardization and improvements are needed for its real-world application.
4. Therapeutic intervention
AChE inhibitors (Aricept (donepezil), nivalin (galantamine), Rivastach (rivastigmine), Cognex (tacrine)) and a weak antagonist of the NMDA receptor (Namenda (memantine)) are the few drugs approved for the treatment of AD.27 However, these drugs do not act directly on the underlying disease mechanisms and only offer symptomatic and temporary relief in cases of mild to moderate AD.70 In other words, there are no approved drugs that target the core pathology of AD to slow or prevent the progression of the disease.71 Moreover, memantine was approved in 2003, and since then no new treatments have been developed for AD. Another cause for concern in the community is that there are absolutely no treatments for advanced stages of AD. Although a large number of drugs have undergone clinical trials, most of them failed (99.6%) at various stages of the trials. In spite of disappointing failures, there has been appreciable progress in understanding the disease mechanisms and with respect to tangible biomarkers and targets. The lessons and knowledge gained from numerous failures and setbacks will definitely help academic researchers, clinicians and pharmaceutical companies to develop effective and successful drug candidates for AD in the near future. In fact, a significant number of drug candidates that target various therapeutic pathways in AD are undergoing preclinical and clinical trials. We discuss drug candidates that target various therapeutic pathways in AD in the following section, which includes efforts by our own research group to develop multifunctional modulators as drug candidates.
4.1 Targeting Aβ and Tau aggregation
The presence of extracellular senile plaques (Aβ peptide) and intracellular NFTs (Tau protein) is a hallmark of AD. Targeting the aggregation process of Aβ and Tau has been an active strategy for the design and development of therapeutic agents for pharmacological intervention in AD. Molecules that can effectively (i) prevent fibrillogenesis or dissolve plaques, (ii) destabilize toxic oligomers, (iii) prevent metal-mediated aggregation or (iv) clear toxic aggregates from the brain are termed as aggregation modulators. This section has been divided into several subsections on the basis of the nature and action of the drug candidate.
4.1.1 Small molecules. The modulation of amyloid aggregation (Aβ and Tau) by small molecules has been an effective strategy and has met with reasonable success. However, most small-molecule-based aggregation modulators have failed in clinical trials.72 This scenario necessitates the careful and comprehensive design of efficient functional drug candidates for targeting amyloid aggregation that takes into account the reasons for failure and missing links in previous approaches. Several synthetic derivatives have been developed and tested as modulators of Aβ or Tau aggregation.73 Among these, very few molecules, such as cyclohexane-1,2,3,4,5,6-hexaol (Aβ aggregation) and methylthioninium chloride (LMTM: TRx-0237) (Tau aggregation), have advanced to clinical trials, although the trials were subsequently stopped owing to discouraging results (Fig. 5).74,75 In the search for aggregation modulators, natural products have displayed promising results. Natural products such as curcumin, resveratrol and EGCG have been shown to effectively decrease the Aβ plaque load and improve cognition in mice with AD as a result of their anti-aggregation and antioxidant properties (Fig. 5).76 Similarly, brazilin, which is derived from Caesalpinia sappan, inhibits the aggregation of Aβ42 and prevents the formation of secondary nucleation cores for the further fibrillogenesis of Aβ42 to form toxic Aβ42 species.77 Tanshinone, which is extracted from beans from Voacanga africana, inhibits the fibrillogenesis of Aβ42, disrupts preformed Aβ42 aggregates and ameliorates the neuronal toxicity of Aβ.78 Orcein, which is a natural product extracted from Roccella tinctoria, is an accelerator of the aggregation of Aβ42. It interacts with the hydrophobic region of toxic Aβ42 oligomers and protofibrils and accelerates the formation of relatively less toxic Aβ42 fibrillar aggregates.79 Oleuropein aglycone, which is a phenolic derivative obtained from olives, binds to Tau and prevents its aggregation to form toxic species.80 Although small molecules and natural products have shown promising results as aggregation modulators, their effectiveness in clinical trials has yet to be systematically assessed. Moreover, the success of aggregation modulators based on small molecules or natural products depends on the incorporation of additional features that alleviate the multifaceted toxicity of AD.
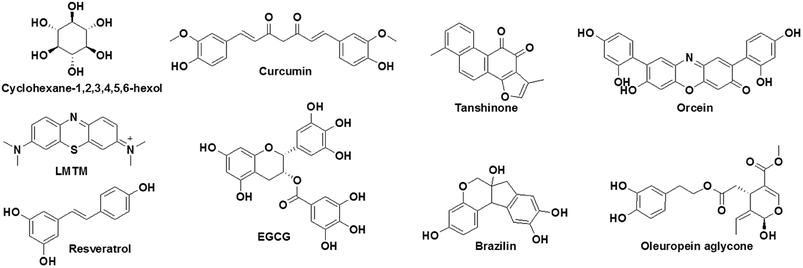 |
| Fig. 5 Aggregation inhibitors based on small molecules and natural products. | |
4.1.2 Peptidomimetics. Peptidomimetics are synthetic analogues of natural peptides or proteins with improved biostability, bioavailability and ability to interact with biological targets to produce similar or superior biological effects.81,82 Design strategies for developing peptidomimetic-based aggregation modulators have mostly relied on targeting the hydrophobic core recognition units of Aβ (KLVFF) and Tau (VQIVYK and VQIINK).83,84 The hydrophobic core recognition sequences interact with and inhibit the aggregation of the respective parent peptide or protein. However, weak interactions of the core recognition sequence with the parent peptide or protein and proteolytic instability result in low inhibition efficiency. Attempts to improve the proteolytic stability and binding interactions of natural core recognition sequences led to the development of peptidomimetic inhibitors. Some of the synthetic approaches used to generate peptidomimetics as aggregation modulators include backbone modification, side chain modification, the introduction of specific organic moieties and cyclization.81,85–87 As has already been suggested, the inhibition of aggregation alone is not sufficient to tackle the multifactorial pathology of AD. Therefore, multifunctional peptidomimetic-based aggregation modulators have been developed, which are capable of targeting other pathophysiological factors involved in AD. We have reported a new class of hybrid cyclic peptoids that were designed to modulate autophagy, which is a natural process for clearing toxic aggregates in AD.88 A simple, differential cyclization of Nα-alkyl- and Nα-acyl-substituted N-(2-aminoethyl)glycine derivatives was devised by taking advantage of the rigid and flexible backbone to form six- and twelve-membered products, respectively, via intra- and intermolecular cyclization (Fig. 6a). The synthesised hybrid cyclic peptoids were screened for the modulation of autophagy using a firefly luciferase assay in yeast, in which the degradation of a cargo marker (GFP-tagged) was an indicator of autophagy activity. Among the cyclic peptoids that were screened, 4-benzylpiperazin-2-one (4a) gave rise to an increase in the rate of degradation of the protein marker via autophagy in a dose-dependent manner (Fig. 6b). Furthermore, modifications of 4a in terms of the structure–activity relationship (SAR) are currently in progress to develop effective modulators of autophagy that target the pathology of AD.
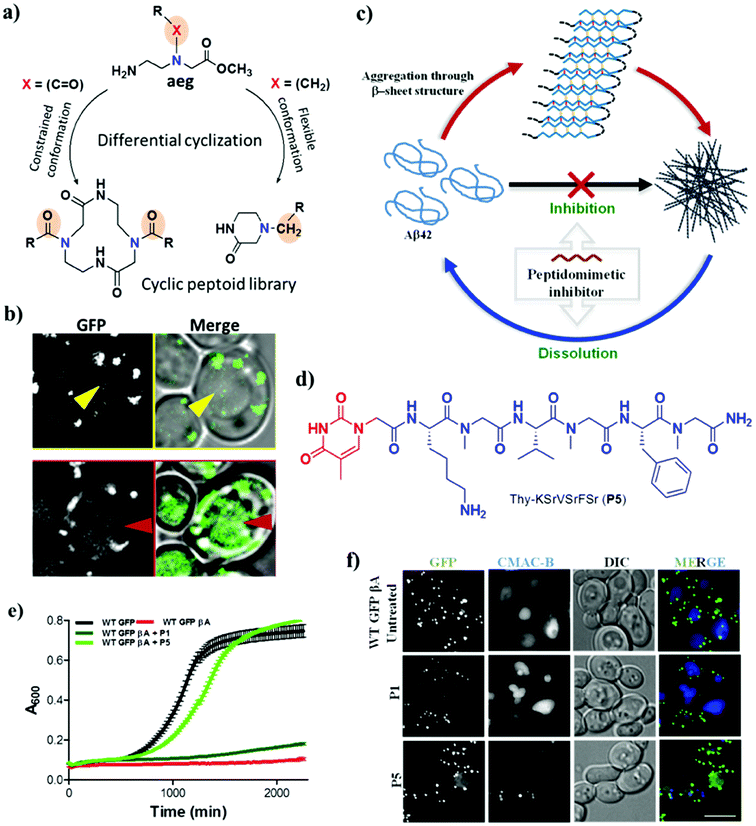 |
| Fig. 6 Peptidomimetic modulators. Cyclic hybrid peptoids (a) as autophagy modulators (b). (c) Schematic representation of inhibition and dissolution of Aβ42 aggregates by the peptidomimetic inhibitor P5. (d) Structure of P5. (e) Growth curve of yeast (autophagy model) with Aβ toxicity. P5 rescues yeast cells from Aβ toxicity. (f) Degradation of toxic GFP-βA by P5 in a WT GFP-βA yeast model. This figure has been adapted from ref. 88 and 89 with permission from Wiley and Nature Publishing Group, respectively. | |
In a similar strategy, linear peptidomimetic modulators were designed, and the hybrid peptoid P5 was identified as a potential modulator of the aggregation of Aβ and promoted the clearance of toxic Aβ aggregates by activating autophagy in a yeast model of AD (Fig. 6c).89 The design of these peptidomimetics was based on the recognition unit of Aβ peptide (KLVFFA), which interferes with hydrogen bonding and other non-covalent interactions that are responsible for the aggregation Aβ. The peptidomimetics that were designed contain N-terminal thymine/barbiturate and sarcosine (N-methylglycine) at alternate amino acid positions in KLVFFA to target intramolecular and intermolecular hydrogen bonds involved in the aggregation of Aβ. The hybrid peptoid P5 with an N-terminal thymine unit and sarcosine at alternate positions effectively inhibited the aggregation of Aβ and dissolved preformed Aβ42 aggregates (Fig. 6d). Furthermore, P5 exhibited high proteolytic and serum stability in comparison with the unmodified peptide (KLVFFA). Interestingly, P5 rescued yeast cells affected by the toxicity of Aβ by preventing aggregation or dissolving preformed aggregates and in the process promoted their clearance via the autophagy pathway (Fig. 6e and f). In other words, P5 exhibited no rescue effect in autophagy-defective mutant (Δatg1 mutant) yeast cells, which confirms the autophagy-dependent rescue of cells from the toxicity of Aβ. This is the first study that successfully employed a peptidomimetic/peptoid modulator to activate autophagy and promote the clearance of toxic Aβ aggregates from cells.90 Although there have been many reports on the modification of recognition units to develop aggregation modulators, few of these were equipped with the multifunctional ability to alleviate the multifaceted toxicity of AD.91–94
According to the metal ion hypothesis, the complexation of redox-active metal ions such as Cu2+ with Aβ peptide or their inclusion in amyloid aggregates accelerates the toxicity of Aβ.25,95 Therefore, the design of peptide- or peptidomimetic-based metal chelators/sequestrators has been an active area of research, although most of the reported chelators lack multifunctional features.96,97 We developed the multifunctional peptidomimetic P6 by conjugating the copper-chelating natural tripeptide Gly-His-Lys (GHK) found in humans and the peptoid-based Aβ aggregation modulator P5 (Fig. 7a and b).98 The Aβ42/Cu2+ complex has an association constant (Ka) of ∼109 to 1012, and hence a metal chelator with a Ka value of >109 is required to sequester Cu2+ from the Aβ-Cu2+ complex. GHK has a Ka value of ∼1014, and therefore it can easily sequester the metal ion from the Aβ-Cu2+ complex. Notably, GHK is incapable of sequestering Cu2+ from biologically relevant metalloprotein complexes (Ka of ∼1015 to 1017), which makes GHK an ideal candidate for sequestering Cu2+ from the Aβ-Cu2+ complex without interfering with the activity of copper-based metalloenzymes. P6 effectively inhibits the formation of toxic Aβ oligomeric species and fibrillar aggregates. The GHK unit in P6 retains the ability to chelate CuII, and as a result P6 effectively sequesters CuII from the Aβ42–Cu2+ complex and maintains it in a redox-dormant state. Aβ42–CuII in a reducing environment is known to generate excess ROS via the redox cycling of CuII, and elevated levels of ROS damage biomacromolecules, which contributes to additional cellular toxicity. Data from an in vitro assay to assess Aβ42–CuII-induced DNA damage showed that P6 efficiently sequestered CuII from Aβ42–CuII and protected DNA from ROS-mediated damage (Fig. 7c). The multifunctional ability of P6 to inhibit the multifaceted toxicity of Aβ was studied in PC12 cells (Fig. 7d). Cells were rescued from Aβ42–CuII-induced toxicity, and the amount of ROS that was generated was substantially reduced. The sequestration of the metal, moderation of the aggregation of Aβ and generation of ROS, protection of DNA, and antioxidant property to prevent oxidative stress make P6 a multifunctional inhibitor of the multifaceted toxicity of Aβ. Although the multifunctional ability of peptides and peptidomimetics to modulate the multifaceted toxicity of Aβ is promising, their probable limitations, including blood–brain barrier (BBB) permeability, size and overall stability, could hamper their potential applications for the treatment of AD. Moreover, the modulatory efficacies of small molecules are far superior in comparison with those of peptidomimetics, and as a result none of the peptidomimetics has reached clinical trials. Notwithstanding their limitations, the design and study of peptidomimetics have huge implications for understanding the mechanism of amyloid aggregation (amyloid hypothesis) and its relation to other hypotheses, which in turn may assist the development of selective and efficient disease-modifying therapeutics for AD.
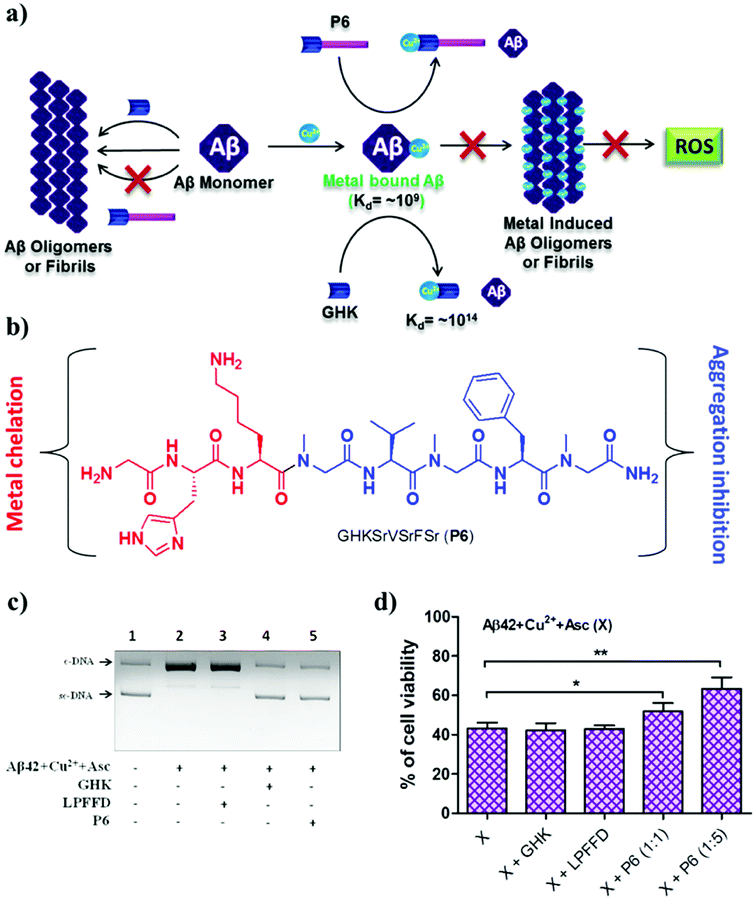 |
| Fig. 7 Multifunctional peptidomimetic modulators. (a) Schematic representation showing modulation of the multifaceted toxicity of Aβ by the multifunctional modulator P6. (b) Molecular structure of P6. (c) P6 rescues plasmid DNA (pBR322) from damage by ROS generated by the Aβ42 + CuII + Asc system. (d) P6 rescues PC12 cells from toxicity induced by metal-induced Aβ42 aggregates. This figure has been adapted from ref. 98 with permission from the American Chemical Society. | |
4.1.3 Metal chelators. Aβ–metal (Cu, Fe and Zn) complexation and the related toxicity is at the core of the metal ion hypothesis.24,27 The disruption of Aβ–metal interactions using suitably designed metal chelators has been an active area of research. In recent years, extensive research into the development of metal chelators that target CuII in the Aβ–CuII complex has been undertaken to modulate metal-induced toxicity.99 Several metal chelators have been reported to exhibit promising activity in both in vitro and in vivo studies. Among these, the 8-hydroxyquinoline analogues clioquinol (Clq) and PBT2 have reached clinical trials (Fig. 7a). In fact, Clq was the first metal chelator to receive approval for clinical trials, but unfortunately failed owing to extreme toxicity.100 The second-generation analogue PBT2 displayed promising results in comparison with Clq, although it failed in phase 2 clinical trials (Fig. 8a).101 Despite these failures, Clq and its derivatives continue to inspire the next generation of metal chelators for modulating the toxicity of Aβ–CuII.102–104 In recent years, the design of multifunctional metal chelators has received enormous interest for targeting the multifactorial toxicity found in AD. We designed hybrid multifunctional modulators (HMMs) by the integration of structural (hydroxyquinoline and polyphenolic moieties) and functional features (metal chelation and antioxidant properties) of Clq and EGCG, respectively (Fig. 8).105 The lead HMM, namely, TGR86, effectively modulated both metal-dependent and -independent aggregation of Aβ. A computational study revealed that TGR86 interacts with the loop region of Aβ42 in the aggregate state via multiple hydrogen-bonding and π-alkyl interactions. The enhanced modulation of the aggregation of Aβ42 by TGR86 is also attributed to disruption of the formation of a salt bridge (Lys28-Asp23), as revealed by the computational study. TGR86 exhibits strong affinity for CuII and hence sequesters it from the Aβ–CuII complex and maintains it in a redox-dormant state, which prevents the redox cycling process responsible for the generation of excess ROS. Unlike highly cytotoxic Clq, the lead HMM TGR86 enabled high cell viability. In cellulo studies of the effect of TGR86 against the toxicity of Aβ42–CuII in PC12 cells showed a significant improvement in cell viability (Fig. 8d). It has been reported in the literature that Aβ interacts with import channels present in the outer mitochondrial membrane, which leads to a decrease in the mitochondrial membrane potential (MMP) and elevated levels of oxidative stress.30,106 Mitochondrial membrane damage and dysfunction are also considered to be among the hallmarks of the toxicity of Aβ observed in AD. Thus, the prevention of mitochondrial damage and the generation of ROS is of immense interest in modulating the pathology of AD.107 Evidently, cellular studies of TGR86 showed a significant improvement in the MMP in comparison with neuronal cells treated with Aβ alone (Fig. 8e and f). TGR86 binds toxic Aβ42 and prevents its interaction with the mitochondrial membrane, which is confirmed by the improvements in the membrane potential and cell viability.108 The development of multifunctional metal chelators is at an early stage, and more dedicated efforts are required to produce efficient and viable next-generation drug candidates to target the multifaceted toxicity of Aβ.
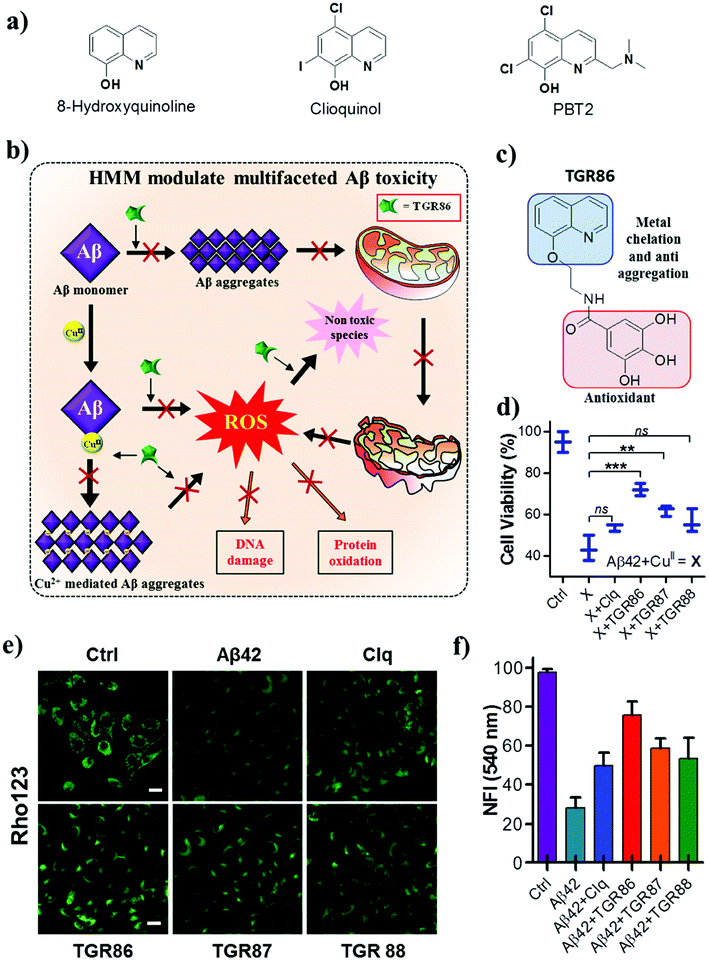 |
| Fig. 8 (a) Small-molecule-based metal chelators and hybrid multifunctional modulators (HMMs). (b) Schematic representation of inhibition of the multifaceted toxicity of Aβ and mitochondrial damage by an HMM. (c) Molecular structure of an HMM (TGR 86). (d) TGR86 rescues PC12 cells from toxicity induced by metal-induced Aβ42 aggregates. TGR86 rescues the mitochondrial membrane potential of PC12 cells from the metal-induced toxicity of Aβ42. (e) Microscopic images and (f) quantification of fluorescence at 540 nm (λex = 511 nm). This figure has been adapted from ref. 105 with permission from the American Chemical Society. | |
4.1.4 Immunotherapy. Immunotherapy is the manipulation of the immune system to elicit the necessary immune response for the treatment of disease.109 The concept of immunotherapy has received strong interest from researchers, clinicians and pharmaceutical companies for the development of an effective treatment for AD. Several immunotherapies have been developed and tested in clinical studies aimed at countering the pathology of amyloid or Tau. Immunotherapy in AD can be broadly divided into two major categories: (i) active immunisation (Aβ or Tau is administered to patients as an antigen) and (ii) passive immunisation (antibodies against Aβ or Tau are administered to the patient) (Fig. 9a). Antibodies are expected to clear amyloid deposits in the brain by binding to plasma Aβ and promoting its clearance from the blood, which further creates a gradient effect and ultimately causes the clearance of Aβ from the brain. Alternatively, antibodies can bind to deposits of Aβ or Tau and activate macrophages, which in turn affect the clearance of aggregates of Aβ or Tau from the brain.110 Currently, immunotherapy has gained considerable importance over other therapeutic strategies; in particular, targeting amyloid aggregates (Aβ and Tau) has been found to be a promising approach. In fact, most of the notable players in this area are pharmaceutical companies, which signifies the importance of the amyloid hypothesis and amyloid aggregates as drug targets for the development of disease-modifying therapies for the pathology of AD. The relatively large size, biostability, ineffective crossing of the BBB, undesired immunogenic response and high cost of antigens/antibodies are some of the key factors that need to be considered when developing an effective immunotherapy for AD.
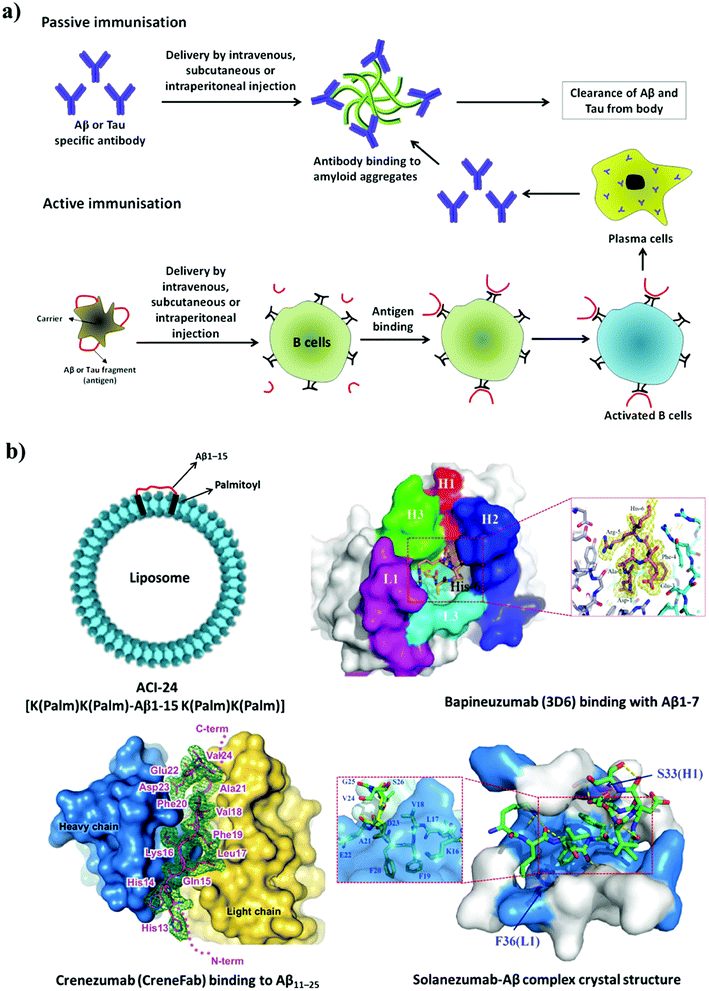 |
| Fig. 9 (a) Active and passive immunotherapy. (b) Liposome-based active immunisation and interaction of anti-Aβ antibodies with Aβ. This figure has been adapted from ref. 120, 123 and 124 with permission from Nature Publishing Group (120 and 124) and BioMed Central (123). | |
4.1.4.1 Active immunotherapy. Peptide-based vaccines have been designed on the basis of sequences derived from Aβ and Tau for active immunisation (Fig. 9). In the selection of peptides, sequences responsible for the activation of T-cells were avoided and only fragments responsible for the generation of specific antibodies were considered. Nicolau et al. developed a liposome-based vaccine (ACI-24, phase 1 clinical trials) to generate antibodies against Aβ aggregates without causing activation of inflammatory T-cells.111 The N-terminal fragment of Aβ (Aβ1–15) is devoid of T-cell epitopes and prevents the activation of T-cells. In the design of peptide vaccines, Aβ1–15 was modified with palmitoylated lysine linkers on both the N-terminus and the C-terminus, and this synthetic modification helped to anchor Aβ1–15 to a liposome (Fig. 9b). Aβ1–15 when immobilized on a liposomal membrane adopts a β-sheet structure, which acts as an epitope and rapidly stimulates the generation of anti-Aβ antibodies. These antibodies have been shown to dissolve amyloid aggregates in mice with AD (APPxPS-1). Similarly, a liposome-based vaccine for targeting defined pathological conformers of phosphorylated Tau (ACI-35, phase 1 trials) was developed.112 This vaccine comprised a synthetic phosphorylated Tau fragment (16 copies) anchored to a lipid bilayer. Long-term vaccination with ACI-35 in a mouse model of AD improved the clinical condition and reduced tauopathy in the brain without initiating neuroinflammation.ABvac40 (Araclon Biotech) is the first active vaccine developed to target the C-terminus of Aβ40 and is undergoing phase 2 clinical trials.113 Patients who were treated with ABvac40 generated antibodies against Aβ40 without any significant side effects. AADvac-1 is another active vaccine developed to provoke an immune response against pathological Tau and is undergoing phase 3 trials. It consists of a peptide derived from Tau (KDNIKHVPGGGS) coupled to keyhole limpet hemocyanin.114 Vaccination with AADvac-1 induced an active immune response with the production of antibodies capable of discriminating pathological and physiological Tau. A significant reduction in the formation of neurotoxic Tau oligomers and the hyperphosphorylation of Tau (∼95%) in the brain was reported. CAD106 (Novartis Pharmaceuticals, phase 2 trials) is a virus-particle-based active vaccine that was prepared by coupling multiple copies of the Aβ1–6 peptide fragment to a Qβ virus-like particle.115 In models of AD, CAD106 induced anti-Aβ antibodies without activating T-cells, which prevented an unwanted inflammatory response. The administration of CAD106 to APP transgenic mice caused a significant reduction in the accumulation of Aβ, and there was no evidence of an increase in microhemorrhages or inflammatory reactions. LuAF20513 (phase 1 trials) is an active vaccine that contains three alternating repeats of an Aβ peptide fragment (Aβ1–12) with sequences from tetanus toxin.116 This engineered mixed-peptide antigen induces the production of anti-Aβ antibodies that reduce the pathology of AD without inducing microglial activation in a model of AD. Another distinct and alternative approach to active immunisation involves a DNA-based vaccine, whereby DNA that encodes Aβ peptide is injected into a patient. DNA is translated to produce Aβ peptide, which subsequently triggers an immune response to generate an Aβ-specific antibody.117 Although DNA-based immunisation is an interesting strategy, this approach is in the early stages of development and has not yet reached clinical trials.
4.1.4.2 Passive immunotherapy. Humanised anti-Aβ antibodies are administered in passive immunisation therapy (Fig. 9). Aducanumab (Biogen, phase 3 trials) is a human monoclonal antibody used against Aβ aggregates. In mice with AD, aducanumab was found to cross the BBB and preferentially binds to parenchymal over vascular amyloid aggregates.118 Aducanumab reduces soluble and insoluble Aβ aggregates in a dose-dependent manner in the brain in AD. Crenezumab (Genentech, phase 3 trials) is a humanised monoclonal antibody developed to recognise polymorphic forms of Aβ aggregates such as oligomers, fibrils and amyloid plaques with high affinity over Aβ monomer (Fig. 9b).119,120 This binding interaction of the antibody promotes the clearance of neurotoxic Aβ aggregate species from the brain, with limited effects on microglia, which prevents the unwanted release of inflammatory cytokines and thus avoids side effects. Similarly, gantenerumab (Chugai Pharmaceutical), which is a human antibody that specifically binds to Aβ fibrils, is undergoing phase 3 trials.121 This antibody binds to Aβ amyloid plaques, attracts microglia and activates phagocytosis. Interestingly, gantenerumab interacts with both parenchymal and vascular Aβ aggregates. In transgenic mice with AD (APP/PS-1), gantenerumab was found to bind Aβ and dissolve plaques by recruiting microglia and prevents the formation of newer plaques without altering plasma Aβ. BAN2401 (Biogen) is a monoclonal antibody that is currently undergoing phase 2 clinical trials and selectively binds to soluble Aβ protofibrils, which promotes their clearance and the neutralisation of toxic species.122 Bapineuzumab (Pfizer) is a humanised form of a murine monoclonal antibody (3D6) that targets the N-terminal region of Aβ (Fig. 9b).123 Bapineuzumab initially showed promising results and reached clinical trials; however, it failed in advanced clinical trials. Solanezumab (Eli Lilly, phase 3 trials) is a humanised monoclonal antibody developed against an Aβ peptide fragment (Aβ13–28) (Fig. 9b).124 It recognises Aβ monomeric species and not fibrillar aggregates. Solanezumab has shown promising results in phase 2 clinical trials; however, in phase 3 trials it failed to prevent cognitive decline in patients with mild AD. The passive immunisation strategy has been promising, although the low BBB permeability, autoimmune response and inefficient retention of antibodies in the brain have hindered the potential application of drugs to treat AD.BIIB092 (Biogen, phase 2 trials) is a humanised anti-Tau monoclonal antibody raised against extracellular N-terminally fragmented truncated forms of Tau (eTau), which have been found to cause neuronal hyperactivity and an increase in the production of Aβ.125 BIIB092 was found to promote the clearance of Tau aggregates from the brain. Similarly, C2N 8E12 is another humanised antibody developed to recognise extracellular pathological Tau aggregates.126 Extracellular Tau has been implicated in the transneuronal propagation of Tau in models of AD. The antibody C2N 8E12 has successfully completed phase 1 clinical trials, and phase 2 trials are in progress. In general, the outcomes of the ongoing and planned clinical trials will show whether immunotherapy that targets Aβ and Tau can prevent or delay the pathology of AD.
4.2 Targeting protein-processing enzymes
The generation of toxic Aβ and pTau is mediated by various processing enzymes. For example, the processing of APP by the successive proteolytic action of β-secretase and γ-secretase releases toxic Aβ peptide. Tau protein bound to microtubules is hyperphosphorylated by the enzymes (kinases) GSK3β and Cdk5, which leads to the abrupt release of pTau and collapse of the microtubules. Thus, therapeutic candidates that target the activity of such processing enzymes have shown significant improvements in slowing the pathology of AD.
4.2.1 β-Secretase inhibitors. CNP520 is an oral drug candidate that inhibits the proteolytic activity of β-secretase on APP and reduces the production of Aβ.127 Tolerability, pharmacokinetics and pharmacodynamics data are encouraging, and CNP520 is currently undergoing phase 2 clinical trials. Similarly, elenbecestat, JNJ-54861911 and LY3202626 are dihydrothiazine-based β-secretase inhibitors that efficiently cross the BBB and displayed a dose-dependent reduction in the production of Aβ peptide (Fig. 10).128,129 Lanabecestat (Eli Lilly, phase 3 clinical trials) displayed significant dose- and time-dependent decreases in Aβ40, Aβ42, and sAβPPβ in plasma, CSF and the brain.130 A sulfonyl-based drug, namely, verubecestat (MK-8931, Merck, phase 3 clinical trials) is a potent and selective β-secretase inhibitor that has been shown to reduce the concentrations of Aβ40, Aβ42, and sAPPβ in biological fluids (Fig. 10).131 However, in phase 3 trials verubecestat failed to decrease the Aβ levels in a patient's brain. It should be noted that β-secretase plays a vital role in improving synaptic plasticity and cognitive performance.132 Therefore, inhibitors of β-secretase activity have given rise to severe adverse effects, which included reduced myelination of neurons, disturbances in glucose homeostasis and hepatotoxicity. This emphasises the need to adopt an unconventional design strategy such as the development of molecular clamps that bind to APP at the β-secretase cleavage site, prevent protease activity and consequently reduce the production of Aβ peptide.133
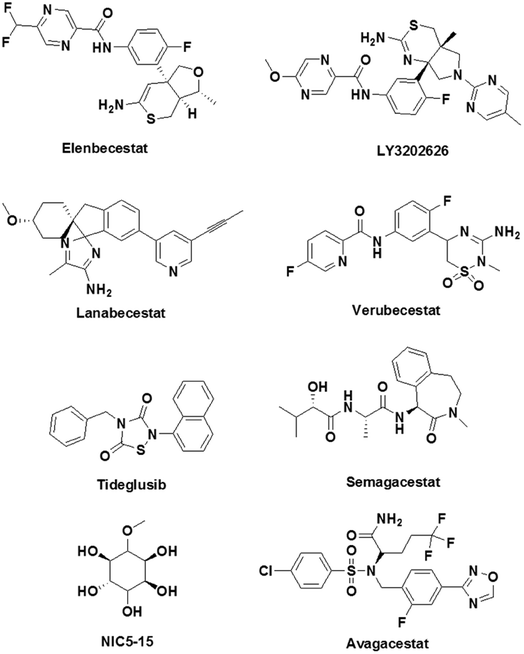 |
| Fig. 10 Molecular structures of inhibitors of β-secretase, γ-secretase and kinases that are undergoing clinical trials. | |
4.2.2 γ-Secretase inhibitors. Semagacestat (Eli Lilly), which is a γ-secretase inhibitor, has enabled reductions in Aβ concentrations in plasma and CSF in a dose-dependent manner. However, semagacestat failed in phase 3 clinical trials owing to severe side effects (Fig. 10). Further investigations revealed that apart from inhibiting γ-secretase, semagacestat interferes with the activity of Notch signalling proteins, which accounts for the observed side effects.134 On the other hand, an arylsulfonamide-based drug, namely, avagacestat, selectively inhibits the activity of γ-secretase without affecting the function of Notch protein, although it failed in phase 2 clinical trials.135 The naturally occurring cyclic sugar alcohol NIC5-15 (phase 3 trials) is a γ-secretase inhibitor that does not interfere with Notch functions, while pharmacokinetics and pharmacodynamics data have shown that NIC5-15 is safe and tolerable for humans without causing side effects (Fig. 10).136 Apart from processing APP, γ-secretase is involved in cleaving numerous physiologically important proteins such as Notch, E-cadherin, CD44, tyrosinase and TREM2.137 Therefore, targeting γ-secretase could potentially result in severe side effects by interfering with other physiological functions involving γ-secretase. As was mentioned in the β-secretase section, the development of molecular clamps that bind to the cleavage site in APP may prevent the activity of γ-secretase and possibly overcome this drawback to provide potential drug candidates.
4.2.3 Kinase inhibitors. Protein kinases have been identified as promising targets because of their involvement in the hyperphosphorylation of Tau.138 An imbalance between the phosphorylation and dephosphorylation of Tau leads to an irreversibly hyperphosphorylated state of the protein. This process is driven by a reduction in the dephosphorylation of Tau or the overactivity of protein kinases. GSK3β plays a vital role in the Tau phosphorylation process and is responsible for the highest percentage of hyperphosphorylation that has been observed. An increase in the deposition of toxic Aβ is known to significantly increase the activity of GSK3β, although the actual mechanism is not clear. Therefore, GSK3β is a potential therapeutic target and has been pursued by many academic researchers and pharmaceutical companies. Many GSK3β inhibitors have been developed, including paullone, maleimide derivatives and indirubin.139 However, high cytotoxicity and undesirable side effects have prevented these GSK3β inhibitors from entering clinical trials. Tideglusib is an orally available thiadiazolidinone-based GSK3β inhibitor, and in preclinical studies it has shown reductions in Tau phosphorylation and neuron loss in a mouse model (Fig. 10).140 The other important Tau protein kinase is Cdk5. The dysfunctioning of Cdk5 contributes to senile plaques, neurofibrillary tangles, synaptic damage, mitochondrial dysfunction and cell cycle-dependent neuronal apoptosis.141 Small-molecule-based Cdk5 inhibitors have been reported in the literature; however, adverse side effects have limited their practical utility. The search for effective potent kinase inhibitors must follow a novel and unconventional molecular approach to drug design that includes finding ways to prevent the phosphorylation of Tau without altering other physiological functions of enzymes. One such unorthodox suggestion would comprise the design of molecular inhibitors that bind to an appropriate site on Tau protein and prevent interactions with kinases and the specific phosphorylation of Tau.
4.3 Targeting inflammation
Inflammation plays a key role in the pathology of AD, and therefore targeting inflammatory pathways is considered to be a promising drug design strategy for AD.38 To target inflammatory processes in AD, two types of drug design strategies are being pursued: (i) the use of anti-inflammatory drugs for the downregulation of inflammation in the brain in AD and (ii) the activation of the immune system to counteract the pathological feature (Aβ deposits) by initiating inflammation. Some of the promising drug candidates that have been developed to target inflammation in AD and are currently undergoing clinical trials are shown in Fig. 11. An imidazole-based drug, namely, azeliragon (Pfizer, phase 3 trials), is an inhibitor of the receptor for advanced glycation end products (RAGE), which is a key receptor in inflammatory processes.142 The cell surface receptor RAGE binds to lipids and proteins, which are glycated when exposed to sugars (advanced glycation end products, AGEs).143 Under conditions in AD the production of AGEs is upregulated, and these subsequently bind to RAGE causing inflammation and oxidative damage. Interestingly, azeliragon blocks AGE/RAGE interactions and prevents inflammation. In clinical studies, treatment with a RAGE antagonist (azeliragon) gave rise to a decrease in the brain load of Aβ and also prevented cognitive decline.
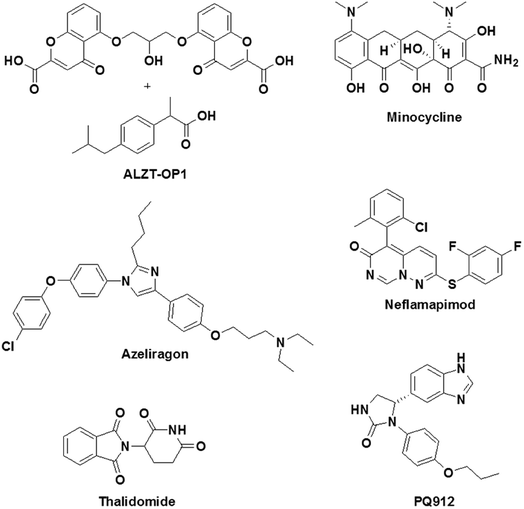 |
| Fig. 11 Molecular structures of modulators of inflammation that are undergoing clinical trials. | |
Glutaminyl cyclase (QC), which is a metalloenzyme, has been shown to be upregulated in AD patients. QC catalyses the cyclisation of the glutamate residue at the N-terminus of Aβ peptide to generate pyroglutamate-Aβ (pGlu-Aβ), which is a highly pathogenic form of Aβ.144 pGlu-Aβ is reported to be extremely toxic and is a major constituent of senile plaques in the brain in AD.145 A benzimidazole-based drug, namely, PQ912 (phase 2 trials), has been shown to inhibit QC in rats, mice and humans. In vivo studies have indicated that PQ912 effectively reduces the pathology of amyloid by inhibiting QC and improves learning and memory.146 A combination therapy using two drugs, namely, ibuprofen and cromolyn, has been developed (ALZT-OP1) for the treatment of AD (Fig. 11). Ibuprofen is a non-steroidal anti-inflammatory drug (suppresses cytokine release) that has been shown to have a significant effect on AD. On the other hand, cromolyn inhibits the aggregation of Aβ monomer to form oligomers and fibrils under in vitro and in vivo conditions.147 ALZT-OP1 is currently undergoing phase 3 clinical trials, and the results are promising. A tetracycline derivative, namely, minocycline (phase 2 trials), is known for its anti-inflammatory properties; it effectively crosses the BBB and exhibits neuroprotective properties via the modulation of inflammation and oxidative stress.148 Mice with AD treated with minocycline exhibited improved spatial memory accompanied by downregulation of the levels of anti-inflammatory cytokines in the brain. Neflamapimod (phase 2 trials) is an inhibitor of the alpha isoform of p38 mitogen-activated protein kinases (p38 MAPK), which are known to activate pro-inflammatory cytokines. In microglia, p38 MAPK activate the release of tumor necrosis factor-alpha (TNF-α) and IL-1β (pro-inflammatory cytokines) in response to various types of stressors such as Aβ42. In neurons, p38 MAPK are involved in manipulating the localisation of Tau and neuronal plasticity.149 A rat model of AD (Tg2576) treated with neflamapimod has shown a decrease of 50% in Aβ load and a reduction in the level of pro-inflammatory cytokines such as IL-1β.150 Thalidomide (phase 2 trials) is an immunomodulatory agent that exhibits anti-inflammatory activity by preventing the production of TNF-α.151
Etanercept (Pfizer, phase 2 trials) is a fusion protein developed from a TNF-α receptor and the Fc end of the antibody immunoglobulin G. Etanercept binds to the pro-inflammatory cytokine TNF-α and prevents its function.152 In fact, etanercept is an FDA-approved treatment for various rheumatological and inflammatory skin diseases. However, etanercept failed to provide significant improvements in cognition and behavioural assays.153 Although none of the drug candidates that have been discussed are effective without causing significant side effects, there is a need to develop modulators of inflammation that target inflammation in the pathology of AD.
4.4 Targeting cholinergic system
Small molecules that target the cholinergic system and NMDA receptor are the only FDA-approved drugs available for the treatment of mild to moderate AD (Fig. 12). These drugs provide symptomatic and temporary relief to the patient by modulating the levels of neurotransmitters and do not directly treat the underlying disease mechanisms.32 Donepezil, galantamine, rivastigmine and tacrine are FDA-approved drugs that inhibit AChE and/or butyrylcholinesterase, which are involved in the degradation of the neurotransmitter ACh (Fig. 12). The inhibition of AChE activity leads to increased levels of ACh in synapses, which improves cholinergic transmission and provides temporary improvements in memory and cognition. A prodrug of galantamine (GLN-1062, phase 1 trials) has been developed to increase the lipophilicity to improve its BBB permeability. The prodrug GLN-1062 enters the brain and is enzymatically cleaved to release the active drug galantamine.154 Histamine H3 receptors are predominantly expressed in the central nervous system over the peripheral nervous system and modulate cholinergic systems. An active histamine H3 receptor antagonist, namely, SUVN-G3031, was developed to treat the cognitive deficits found in AD. In vivo studies have shown enhanced cholinergic signalling, reduced phosphorylation of Tau, and improved behavioural deficits.155 Memantine is a low- to medium-affinity NMDA antagonist of glutamate receptors in the brain. The binding of memantine affects NMDA receptor-operated ion channels, which reduces the effects of excitotoxic glutamate release. Memantine binds to the NMDA receptor with greater affinity than Mg2+, which inhibits the prolonged influx of Ca2+ from extrasynaptic receptors and forms the basis of neuronal excitotoxicity.156
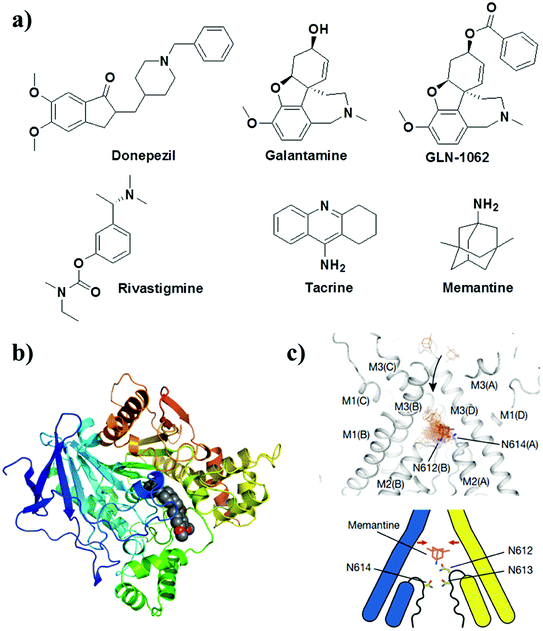 |
| Fig. 12 (a) Cholinergic inhibitors and NMDA antagonist for treating AD. Molecular docking of donepezil (b) and memantine (c) with AChE and NMDA receptors, respectively. This figure has been adapted from ref. 156 with permission from Nature Publishing Group. | |
5. Conclusion and outlook
We have described various molecular and pathophysiological mechanisms that encompass synaptic function, metal homeostasis, neural transmission, ATP production, memory formation and autophagy, among others that are severely affected in AD. Although multiple hypotheses have been proposed to explain the pathology of AD, the amyloid hypothesis is the most widely accepted and is considered to be a realistic target for the development of diagnostic and therapeutic interventions. The current diagnosis mostly comprises evaluation of mental status, mood testing and cognitive behavior, but symptomatic and behavioral changes are manifested only in the advanced stages of AD. The detection of biomarkers (Aβ load) in biological fluids such as blood and CSF via in vivo fluorescence imaging of the human brain using selective NIR probes is a promising approach, which needs to be further developed into a viable technique and validated for clinical use. In vivo fluorescence imaging of the human brain is currently limited by the technique (unavailability of suitable equipment) itself, but the situation is expected to change in the near future. At present, PET is the most promising technique for the early diagnosis of AD. Although some probes have been approved for clinical use, the lack of highly selective and sensitive PET probes for biomarkers of AD (Aβ and Tau aggregates) has limited their widespread clinical utility. Nevertheless, PET imaging coupled with MRI is expected to enhance our understanding of structural and functional changes in the brain during the progression of AD, which can be used to develop brain maps as a standard in brain analysis for the early diagnosis of AD. The detection of biomarkers in the brain is still a challenging task and requires sophisticated, safe and practically viable techniques. Recent efforts in the identification of biomarkers (APP/Aβ42 and Aβ40/Aβ42) in biological fluids (blood) have been promising. In this study, a composite parameter was derived from the concentrations of biomarkers to define the amyloid load in the brain with an accuracy of 90% and statistical significance. However, further standardisation and improvements in sensitivity and accuracy are necessary for its practical utilisation. Furthermore, many biomarkers have a direct or indirect role in the pathology of AD, and multiplexing their analysis in biological fluids could possibly lead to a realistic and timely diagnosis of AD.
Progress in the development of therapeutic interventions for preventing the symptoms of AD has been encouraging, although several drug candidates have unfortunately failed at various stages of clinical trials. These failures are mainly attributed to the fact that most of these drugs interfere with one of the many disease pathways of multifactorial AD. In other words, the complex pathophysiology of AD involves the dysfunction of various key molecular pathways, and successful drug design must take into account the integration of multiple disease pathways. In this context, the multifunctional approach that we adopted in our laboratory could be an effective and promising strategy for developing drugs to target the multifaceted pathology of AD. In fact, targeting amyloid aggregation (Aβ and Tau aggregates) using small molecules, natural products, peptidomimetics and metal chelators has been actively pursued; however, the discouraging outcomes have reoriented the attention of researchers to focus on the development of multifunctional drug candidates. Such drug candidates must be capable of preventing the aggregation of Aβ and Tau, metal chelation, metal-induced aggregation, the generation of excess ROS and oxidative stress, damage to biomolecules by ROS, inflammation, mitochondrial damage, synaptic toxicity, the activity of secretase and kinase enzymes and many other processes. In recent years, immunotherapy has been considered to be one of the most probable therapeutic strategies for targeting the clearance of amyloid from the brain and has been actively pursued by leading pharmaceutical companies. Although immunotherapy is promising, a few critical issues, such as low BBB permeability, size, autoimmune response, stability, retention in the brain and the high cost of development and treatment, need to be addressed before it finds practical application. Preventing the activity of enzymes such as β-secretase, γ-secretase and kinases in the pathology of AD is a viable strategy for drug discovery. Current approaches to targeting these enzymes have shown adverse side effects, which were attributed to their vital role in many other biological process in the brain. Thus, we propose the design of molecular clamps that bind to the β-secretase/γ-secretase cleavage site in APP or specific phosphorylation sites in Tau and subsequently prevent the undesirable specific activity of enzymes. Such a therapeutic design approach may yield potential drug candidates that effectively inhibit enzymatic (protease/phosphorylation) activity without interfering with the other biological functions of enzymes. Drugs that target inflammation and the cholinergic system have mostly provided symptomatic relief, although these drugs can be made potent with improved therapeutic effects if used in combination with multifunctional drugs that target the core pathology of AD. Our understanding of AD in terms of complex molecular and pathophysiological mechanisms is very limited in spite of the vast available literature. In fact, the actual cause and realistic molecular targets need to be discovered and validated on the basis of existing and new knowledge. AD has become a common epidemic worldwide, and the research community across various disciplines needs to collaborate to provide better and faster solutions in terms of managing the disease via effective diagnostic and therapeutic interventions. The ambitious neuroscience and brain projects launched in the United States (Brain Research through Advancing Innovative Neurotechnologies (BRAIN) Initiative), Europe (Human Brain Project (HBP)), Japan (Brain Mapping by Integrated Neurotechnologies for Disease Studies (Brain/MINDS)) and China (China Brain Project) with the aim of developing novel technologies and unraveling mysteries of the brain are expected to help researchers to understand and develop effective diagnostic and therapeutic strategies for brain disorders, mainly AD. Although many individual research groups are actively involved, there are no dedicated grand initiatives in the area of neuroscience and brain disorders in India. Notwithstanding the setbacks, we are hopeful that research efforts and knowledge accumulated over the years will supplement the ongoing and forthcoming efforts in drug discovery to successfully develop effective diagnostic and therapeutic interventions for AD in the near future.
Conflicts of interest
There are no conflicts to declare.
Acknowledgements
We thank Prof. C. N. R. Rao FRS for constant support and encouragement, JNCASR, the Swarnajayanti Fellowship, the Department of Science and Technology (DST), Government of India (Grant: DST/SJF/CSA-02/2015–2016), TRC-JNCASR (TRC-JNC/ TG/4426) and Sheikh Saqr Laboratory (SSL), ICMS-JNCASR, for financial support.
Reference
- M. Prince, R. Bryce, E. Albanese, A. Wimo, W. Ribeiro and C. P. Ferri, Alzheimer's Dementia, 2013, 9, 63–75 CrossRef PubMed.
- K. Rajasekhar, M. Chakrabarti and T. Govindaraju, Chem. Commun., 2015, 51, 13434–13450 RSC.
- R. E. Becker, N. H. Greig, E. Giacobini, L. S. Schneider and L. Ferrucci, Nat. Rev. Drug Discovery, 2013, 13, 156 CrossRef PubMed.
- Y. Huang and L. Mucke, Cell, 2012, 148, 1204–1222 CrossRef PubMed.
- Alzheimer's Association, Alzheimer's Dementia, 2016, 12, 459–509 CrossRef.
- http://www.sciwri.club/archives/6194.
- I. W. Hamley, Chem. Rev., 2012, 112, 5147–5192 CrossRef PubMed.
- A. S. DeToma, S. Salamekh, A. Ramamoorthy and M. H. Lim, Chem. Soc. Rev., 2012, 41, 608–621 RSC.
- C. A. Ross and M. A. Poirier, Nat. Med., 2004, 10, S10 CrossRef PubMed.
- S.-Y. Hung and W.-M. Fu, J. Biomed. Sci., 2017, 24, 47 CrossRef PubMed.
- D. J. Selkoe and J. Hardy, EMBO Mol. Med., 2016, 8, 595–608 CrossRef PubMed.
- S. I. A. Cohen, S. Linse, L. M. Luheshi, E. Hellstrand, D. A. White, L. Rajah, D. E. Otzen, M. Vendruscolo, C. M. Dobson and T. P. J. Knowles, Proc. Natl. Acad. Sci. U. S. A., 2013, 110, 9758–9763 CrossRef PubMed.
- M. P. Murphy and H. LeVine, J. Alzheimer's Dis., 2010, 19, 311 Search PubMed.
- L. Buée, T. Bussière, V. Buée-Scherrer, A. Delacourte and P. R. Hof, Brain Res. Rev., 2000, 33, 95–130 CrossRef.
- K. Iqbal, F. Liu, C.-X. Gong and I. Grundke-Iqbal, Curr. Alzheimer Res., 2010, 7, 656–664 CrossRef PubMed.
- R. B. Maccioni, G. Farías, I. Morales and L. Navarrete, Arch. Med. Res., 2010, 41, 226–231 CrossRef PubMed.
- C. J. Lansdall, Biosci. Horiz., 2014, 7, hzu002 CrossRef.
- G. S. Bloom, JAMA Neurol., 2014, 71, 505–508 CrossRef PubMed.
- F. Panza, V. Solfrizzi, D. Seripa, B. P. Imbimbo, M. Lozupone, A. Santamato, C. Zecca, M. R. Barulli, A. Bellomo, A. Pilotto, A. Daniele, A. Greco and G. Logroscino, BioMed Res. Int., 2016, 2016, 3245935 Search PubMed.
- K. J. Barnham and A. I. Bush, Chem. Soc. Rev., 2014, 43, 6727–6749 RSC.
- D. Maity and T. Govindaraju, Chem. Commun., 2010, 46, 4499–4501 RSC.
- D. Maity, A. K. Manna, D. Karthigeyan, T. K. Kundu, S. K. Pati and T. Govindaraju, Chem.–Eur. J., 2011, 17, 11152–11161 CrossRef PubMed.
- D. Maity and T. Govindaraju, Chem. Commun., 2012, 48, 1039–1041 RSC.
- M. G. Savelieff, S. Lee, Y. Liu and M. H. Lim, ACS Chem. Biol., 2013, 8, 856–865 CrossRef PubMed.
- P. Faller, C. Hureau and G. La Penna, Acc. Chem. Res., 2014, 47, 2252–2259 CrossRef PubMed.
- S. J. C. Lee, E. Nam, H. J. Lee, M. G. Savelieff and M. H. Lim, Chem. Soc. Rev., 2017, 46, 310–323 RSC.
- A. I. Bush and R. E. Tanzi, Neurotherapeutics, 2008, 5, 421–432 CrossRef PubMed.
- H. Sies, Redox Biol., 2015, 4, 180–183 CrossRef PubMed.
- N. Narayanaswamy, S. Narra, R. R. Nair, D. K. Saini, P. Kondaiah and T. Govindaraju, Chem. Sci., 2016, 7, 2832–2841 RSC.
- A. Eckert, K. Schmitt and J. Götz, Alzheimer's Res. Ther., 2011, 3, 15 CrossRef PubMed.
- Y. Feng and X. Wang, Oxid. Med. Cell. Longevity, 2012, 2012, 17 Search PubMed.
- P. Francis, A. Palmer, M. Snape and G. Wilcock, J. Neurol., Neurosurg. Psychiatry, 1999, 66, 137–147 CrossRef.
- A. V. Terry and J. J. Buccafusco, J. Pharmacol. Exp. Ther., 2003, 306, 821–827 CrossRef PubMed.
- R. Wang and P. H. Reddy, J. Alzheimer's Dis., 2017, 57, 1041–1048 Search PubMed.
- R. Malinow, Curr. Opin. Neurobiol., 2012, 22, 559–563 CrossRef PubMed.
- G. Neuroinflammation Working, H. Akiyama, S. Barger, S. Barnum, B. Bradt, J. Bauer, G. M. Cole, N. R. Cooper, P. Eikelenboom, M. Emmerling, B. L. Fiebich, C. E. Finch, S. Frautschy, W. S. T. Griffin, H. Hampel, M. Hull, G. Landreth, L. F. Lue, R. Mrak, I. R. Mackenzie, P. L. McGeer, M. K. O'Banion, J. Pachter, G. Pasinetti, C. Plata–Salaman, J. Rogers, R. Rydel, Y. Shen, W. Streit, R. Strohmeyer, I. Tooyoma, F. L. Van Muiswinkel, R. Veerhuis, D. Walker, S. Webster, B. Wegrzyniak, G. Wenk and T. Wyss–Coray, Neurobiol. Aging, 2000, 21, 383–421 CrossRef.
- V. H. Perry and C. Holmes, Nat. Rev. Neurol., 2014, 10, 217 CrossRef PubMed.
- T. Wyss-Coray and J. Rogers, Cold Spring Harbor Perspect. Med., 2012, 2, a006346 Search PubMed.
- E. Zotova, J. A. R. Nicoll, R. Kalaria, C. Holmes and D. Boche, Alzheimer's Res. Ther., 2010, 2, 1 CrossRef PubMed.
- D. Glick, S. Barth and K. F. Macleod, J. Pathol., 2010, 221, 3–12 CrossRef PubMed.
- A. Zare-shahabadi, E. Masliah, G. V. W. Johnson and N. Rezaei, Rev. Neurosci., 2015, 26, 385–395 Search PubMed.
- J. W. Steele, E. Fan, Y. Kelahmetoglu, Y. Tian and V. Bustos, Postdoc J., 2013, 1, 21–34 Search PubMed.
- A. D. Smith, Proc. Natl. Acad. Sci. U. S. A., 2002, 99, 4135–4137 CrossRef PubMed.
- E. Bagyinszky, Y. C. Youn, S. S. A. An and S. Kim, J. Toxicol. Environ. Health Sci., 2014, 6, 133–147 CrossRef.
- M. Staderini, M. A. Martin, M. L. Bolognesi and J. C. Menendez, Chem. Soc. Rev., 2015, 44, 1807–1819 RSC.
- M.-m. Xu, W.-m. Ren, X.-c. Tang, Y.-h. Hu and H.-y. Zhang, Acta Pharmacol. Sin., 2016, 37, 719 CrossRef PubMed.
- P. Verwilst, H. S. Kim, S. Kim, C. Kang and J. S. Kim, Chem. Soc. Rev., 2018, 47, 2249–2265 RSC.
- J. Hatai, L. Motiei and D. Margulies, J. Am. Chem. Soc., 2017, 139, 2136–2139 CrossRef PubMed.
- K. Rajasekhar, N. Narayanaswamy, N. A. Murugan, G. Kuang, H. Ågren and T. Govindaraju, Sci. Rep., 2016, 6, 23668 CrossRef PubMed.
- K. Rajasekhar, N. Narayanaswamy, N. A. Murugan, K. Viccaro, H.-G. Lee, K. Shah and T. Govindaraju, Biosens. Bioelectron., 2017, 98, 54–61 CrossRef PubMed.
- A. G. Vlassenko, T. L. S. Benzinger and J. C. Morris, Biochim. Biophys. Acta, 2012, 1822, 370–379 CrossRef PubMed.
- W. E. Klunk, H. Engler, A. Nordberg, Y. Wang, G. Blomqvist, D. P. Holt, M. Bergström, I. Savitcheva, G. F. Huang, S. Estrada, B. Ausén, M. L. Debnath, J. Barletta, J. C. Price, J. Sandell, B. J. Lopresti, A. Wall, P. Koivisto, G. Antoni, C. A. Mathis and B. Långström, Ann. Neurol., 2004, 55, 306–319 CrossRef PubMed.
- D. F. Wong, P. B. Rosenberg, Y. Zhou, A. Kumar, V. Raymont, H. T. Ravert, R. F. Dannals, A. Nandi, J. R. Brašić, W. Ye, J. Hilton, C. Lyketsos, H. F. Kung, A. D. Joshi, D. M. Skovronsky and M. J. Pontecorvo, J. Nucl. Med., 2010, 51, 913–920 CrossRef PubMed.
- O. Sabri, M. N. Sabbagh, J. Seibyl, H. Barthel, H. Akatsu, Y. Ouchi, K. Senda, S. Murayama, K. Ishii, M. Takao, T. G. Beach, C. C. Rowe, J. B. Leverenz, B. Ghetti, J. W. Ironside, A. M. Catafau, A. W. Stephens, A. Mueller, N. Koglin, A. Hoffmann, K. Roth, C. Reininger and W. J. Schulz-Schaeffer, Alzheimer's Dementia, 2015, 11, 964–974 CrossRef PubMed.
- H. Watanabe, M. Ono and H. Saji, Sci. World J., 2015, 2015, 6 Search PubMed.
- N. Okamura, S. Furumoto, M. T. Fodero-Tavoletti, R. S. Mulligan, R. Harada, P. Yates, S. Pejoska, Y. Kudo, C. L. Masters, K. Yanai, C. C. Rowe and V. L. Villemagne, Brain, 2014, 137, 1762–1771 CrossRef PubMed.
- M. Brendel, A. Jaworska, F. Probst, F. Overhoff, V. Korzhova, S. Lindner, J. Carlsen, P. Bartenstein, R. Harada, Y. Kudo, C. Haass, F. Van Leuven, N. Okamura, J. Herms and A. Rominger, J. Nucl. Med., 2016, 57, 792–798 CrossRef PubMed.
- L. Declercq, S. Celen, J. Lecina, M. Ahamed, T. Tousseyn, D. Moechars, J. Alcazar, M. Ariza, K. Fierens, A. Bottelbergs, J. Mariën, R. Vandenberghe, I. J. Andres, K. Van Laere, A. Verbruggen and G. Bormans, Mol. Imaging, 2016, 15, 1–15 CrossRef PubMed.
- M. Ono and H. Saji, Int. J. Mol. Imaging, 2011, 2011, 543267 Search PubMed.
- A. B. Newberg, N. A. Wintering, K. Plössl, J. Hochold, M. G. Stabin, M. Watson, D. Skovronsky, C. M. Clark, M.-P. Kung and H. F. Kung, J. Nucl. Med., 2006, 47, 748–754 Search PubMed.
- K. A. Johnson, N. C. Fox, R. A. Sperling and W. E. Klunk, Cold Spring Harbor Perspect. Med., 2012, 2, a006213 Search PubMed.
- R. Wolz, V. Julkunen, J. Koikkalainen, E. Niskanen, D. P. Zhang, D. Rueckert, H. Soininen and J. Lötjönen, The Alzheimer's Disease Neuroimaging, PLoS One, 2011, 6, e25446 CrossRef PubMed.
- B. Olsson, R. Lautner, U. Andreasson, A. Öhrfelt, E. Portelius, M. Bjerke, M. Hölttä, C. Rosén, C. Olsson, G. Strobel, E. Wu, K. Dakin, M. Petzold, K. Blennow and H. Zetterberg, Lancet Neurol., 2016, 15, 673–684 CrossRef PubMed.
- K. Blennow, Neurology and Therapy, 2017, 6, 15–24 CrossRef PubMed.
- L. Sakka, G. Coll and J. Chazal, Eur. Ann. Otorhinolaryngol. Head Neck Dis., 2011, 128, 309–316 CrossRef PubMed.
- L.-F. Lue, A. Guerra and D. G. Walker, Neurology and Therapy, 2017, 6, 25–36 CrossRef PubMed.
- Y. Liu, H. Qing and Y. Deng, Int. J. Mol. Sci., 2014, 15, 7865–7882 CrossRef PubMed.
- A. Koyama, O. I. Okereke, T. Yang, D. Blacker, D. J. Selkoe and F. Grodstein, Arch. Neurol., 2012, 69, 824–831 CrossRef PubMed.
- A. Nakamura, N. Kaneko, V. L. Villemagne, T. Kato, J. Doecke, V. Doré, C. Fowler, Q.-X. Li, R. Martins, C. Rowe, T. Tomita, K. Matsuzaki, K. Ishii, K. Ishii, Y. Arahata, S. Iwamoto, K. Ito, K. Tanaka, C. L. Masters and K. Yanagisawa, Nature, 2018, 554, 249 CrossRef PubMed.
- J. Cummings, G. Lee, T. Mortsdorf, A. Ritter and K. Zhong, Alzheimer's Dementia, 2017, 3, 367–384 Search PubMed.
- Z. Liu, A. Zhang, H. Sun, Y. Han, L. Kong and X. Wang, RSC Adv., 2017, 7, 6046–6058 RSC.
- S.-Y. Hung and W.-M. Fu, J. Biomed. Sci., 2017, 24, 47 CrossRef PubMed.
- K. Cisek, G. L. Cooper, C. J. Huseby and J. Kuret, Curr. Alzheimer Res., 2014, 11, 918–927 CrossRef PubMed.
- S. Salloway, R. Sperling, R. Keren, A. P. Porsteinsson, C. H. van Dyck, P. N. Tariot, S. Gilman, D. Arnold, S. Abushakra, C. Hernandez, G. Crans, E. Liang, G. Quinn, M. Bairu, A. Pastrak and J. M. Cedarbaum, Neurology, 2011, 77, 1253–1262 CrossRef PubMed.
- F. van Bebber, D. Paquet, A. Hruscha, B. Schmid and C. Haass, Neurobiol. Dis., 2010, 39, 265–271 CrossRef PubMed.
- J. Bieschke, Neurotherapeutics, 2013, 10, 429–439 CrossRef PubMed.
- W.-J. Du, J.-J. Guo, M.-T. Gao, S.-Q. Hu, X.-Y. Dong, Y.-F. Han, F.-F. Liu, S. Jiang and Y. Sun, Sci. Rep., 2015, 5, 7992 CrossRef PubMed.
- T. Kai, L. Zhang, X. Wang, A. Jing, B. Zhao, X. Yu, J. Zheng and F. Zhou, ACS Chem. Neurosci., 2015, 6, 879–888 CrossRef PubMed.
- J. Bieschke, M. Herbst, T. Wiglenda, R. P. Friedrich, A. Boeddrich, F. Schiele, D. Kleckers, J. M. Lopez del Amo, B. A. Grüning, Q. Wang, M. R. Schmidt, R. Lurz, R. Anwyl, S. Schnoegl, M. Fändrich, R. F. Frank, B. Reif, S. Günther, D. M. Walsh and E. E. Wanker, Nat. Chem. Biol., 2011, 8, 93 CrossRef PubMed.
- A. Daccache, C. Lion, N. Sibille, M. Gerard, C. Slomianny, G. Lippens and P. Cotelle, Neurochem. Int., 2011, 58, 700–707 CrossRef PubMed.
- I. Avan, C. D. Hall and A. R. Katritzky, Chem. Soc. Rev., 2014, 43, 3575–3594 RSC.
- A. F. Mark, Curr. Opin. Drug Discovery Dev., 2002, 2, 417–423 Search PubMed.
- P. M. Seidler, D. R. Boyer, J. A. Rodriguez, M. R. Sawaya, D. Cascio, K. Murray, T. Gonen and D. S. Eisenberg, Nat. Chem., 2017, 10, 170 CrossRef PubMed.
- L. O. Tjernberg, J. Näslund, F. Lindqvist, J. Johansson, A. R. Karlström, J. Thyberg, L. Terenius and C. Nordstedt, J. Biol. Chem., 1996, 271, 8545–8548 CrossRef PubMed.
- J. Vagner, H. Qu and V. J. Hruby, Curr. Opin. Chem. Biol., 2008, 12, 292–296 CrossRef PubMed.
- J. Kaffy, D. Brinet, J.-L. Soulier, I. Correia, N. Tonali, K. F. Fera, Y. Iacone, A. R. F. Hoffmann, L. Khemtémourian, B. Crousse, M. Taylor, D. Allsop, M. Taverna, O. Lequin and S. Ongeri, J. Med. Chem., 2016, 59, 2025–2040 CrossRef PubMed.
- S. Pellegrino, N. Tonali, E. Erba, J. Kaffy, M. Taverna, A. Contini, M. Taylor, D. Allsop, M. L. Gelmi and S. Ongeri, Chem. Sci., 2017, 8, 1295–1302 RSC.
- K. Rajasekhar, N. Narayanaswamy, P. Mishra, S. N. Suresh, R. Manjithaya and T. Govindaraju, ChemPlusChem, 2014, 79, 25–30 CrossRef.
- K. Rajasekhar, S. N. Suresh, R. Manjithaya and T. Govindaraju, Sci. Rep., 2015, 5, 8139 CrossRef PubMed.
- L. G. Friedman, Y. H. Qureshi and W. H. Yu, Neurotherapeutics, 2015, 12, 94–108 CrossRef PubMed.
- S. Gilead and E. Gazit, Angew. Chem., Int. Ed., 2004, 43, 4041–4044 CrossRef PubMed.
- J. P. Turner, T. Lutz-Rechtin, K. A. Moore, L. Rogers, O. Bhave, M. A. Moss and S. L. Servoss, ACS Chem. Neurosci., 2014, 5, 552–558 CrossRef PubMed.
- A. Paul, K. C. Nadimpally, T. Mondal, K. Thalluri and B. Mandal, Chem. Commun., 2015, 51, 2245–2248 RSC.
- H. Amijee, C. Bate, A. Williams, J. Virdee, R. Jeggo, D. Spanswick, D. I. C. Scopes, J. M. Treherne, S. Mazzitelli, R. Chawner, C. E. Eyers and A. J. Doig, Biochemistry, 2012, 51, 8338–8352 CrossRef PubMed.
- M. G. Savelieff, A. S. DeToma, J. S. Derrick and M. H. Lim, Acc. Chem. Res., 2014, 47, 2475–2482 CrossRef PubMed.
- M. Jensen, A. Canning, S. Chiha, P. Bouquerel, J. T. Pedersen, J. Ostergaard, O. Cuvillier, I. Sasaki, C. Hureau and P. Faller, Chem.–Eur. J., 2012, 18, 4836–4839 CrossRef PubMed.
- A. B. Caballero, L. Terol-Ordaz, A. Espargaró, G. Vázquez, E. Nicolás, R. Sabaté and P. Gamez, Chem.–Eur. J., 2016, 22, 7268–7280 CrossRef PubMed.
- K. Rajasekhar, C. Madhu and T. Govindaraju, ACS Chem. Neurosci., 2016, 7, 1300–1310 CrossRef PubMed.
- A. Robert, Y. Liu, M. Nguyen and B. Meunier, Acc. Chem. Res., 2015, 48, 1332–1339 CrossRef PubMed.
- R. A. Cherny, C. S. Atwood, M. E. Xilinas, D. N. Gray, W. D. Jones, C. A. McLean, K. J. Barnham, I. Volitakis, F. W. Fraser, Y.-S. Kim, X. Huang, L. E. Goldstein, R. D. Moir, J. T. Lim, K. Beyreuther, H. Zheng, R. E. Tanzi, C. L. Masters and A. I. Bush, Neuron, 2001, 30, 665–676 CrossRef PubMed.
- P. J. Crouch, M. S. Savva, L. W. Hung, P. S. Donnelly, A. I. Mot, S. J. Parker, M. A. Greenough, I. Volitakis, P. A. Adlard, R. A. Cherny, C. L. Masters, A. I. Bush, K. J. Barnham and A. R. White, J. Neurochem., 2011, 119, 220–230 CrossRef PubMed.
- A. K. Sharma, S. T. Pavlova, J. Kim, D. Finkelstein, N. J. Hawco, N. P. Rath, J. Kim and L. M. Mirica, J. Am. Chem. Soc., 2012, 134, 6625–6636 CrossRef PubMed.
- C. Lu, Y. Guo, J. Yan, Z. Luo, H.-B. Luo, M. Yan, L. Huang and X. Li, J. Med. Chem., 2013, 56, 5843–5859 CrossRef PubMed.
- J. Geng, M. Li, L. Wu, J. Ren and X. Qu, J. Med. Chem., 2012, 55, 9146–9155 CrossRef PubMed.
- K. Rajasekhar, K. Mehta and T. Govindaraju, ACS Chem. Neurosci., 2018, 9, 1432–1440 CrossRef PubMed.
- L. Pagani and A. Eckert, Int. J. Alzheimer's Dis., 2011, 2011, 925050 Search PubMed.
- C. Guo, L. Sun, X. Chen and D. Zhang, Neural Regener. Res., 2013, 8, 2003–2014 Search PubMed.
- M.-Y. Cha, S.-H. Han, S. M. Son, H.-S. Hong, Y.-J. Choi, J. Byun and I. Mook-Jung, PLoS One, 2012, 7, e34929 CrossRef PubMed.
- P. A. Ott, Nat. Rev. Clin. Oncol., 2018, 15, 267–268 CrossRef PubMed.
- A. Fettelschoss, F. Zabel and M. F. Bachmann, Hum. Vaccines Immunother., 2014, 10, 847–851 CrossRef.
- A. Muhs, D. T. Hickman, M. Pihlgren, N. Chuard, V. Giriens, C. Meerschman, I. van der Auwera, F. van Leuven, M. Sugawara, M.-C. Weingertner, B. Bechinger, R. Greferath, N. Kolonko, L. Nagel-Steger, D. Riesner, R. O. Brady, A. Pfeifer and C. Nicolau, Proc. Natl. Acad. Sci. U. S. A., 2007, 104, 9810–9815 CrossRef PubMed.
- C. Theunis, N. Crespo-Biel, V. Gafner, M. Pihlgren, M. P. López-Deber, P. Reis, D. T. Hickman, O. Adolfsson, N. Chuard, D. M. Ndao, P. Borghgraef, H. Devijver, F. Van Leuven, A. Pfeifer and A. Muhs, PLoS One, 2013, 8, e72301 CrossRef PubMed.
- A.-M. Lacosta, M. Pascual-Lucas, P. Pesini, D. Casabona, V. Pérez-Grijalba, I. Marcos-Campos, L. Sarasa, J. Canudas, H. Badi, I. Monleón, I. San-José, J. Munuera, O. Rodríguez-Gómez, C. Abdelnour, A. Lafuente, M. Buendía, M. Boada, L. Tárraga, A. Ruiz and M. Sarasa, Alzheimer's Res. Ther., 2018, 10, 12 CrossRef PubMed.
- E. Kontsekova, N. Zilka, B. Kovacech, P. Novak and M. Novak, Alzheimer's Res. Ther., 2014, 6, 44 CrossRef PubMed.
- C. Wiessner, K.-H. Wiederhold, A. C. Tissot, P. Frey, S. Danner, L. H. Jacobson, G. T. Jennings, R. Lüönd, R. Ortmann, J. Reichwald, M. Zurini, A. Mir, M. F. Bachmann and M. Staufenbiel, J. Neurosci., 2011, 31, 9323–9331 CrossRef PubMed.
- H. Davtyan, A. Ghochikyan, I. Petrushina, A. Hovakimyan, A. Davtyan, A. Poghosyan, A. M. Marleau, N. Movsesyan, A. Kiyatkin, S. Rasool, A. K. Larsen, P. J. Madsen, K. M. Wegener, D. K. Ditlevsen, D. H. Cribbs, L. O. Pedersen and M. G. Agadjanyan, J. Neurosci., 2013, 33, 4923–4934 CrossRef PubMed.
- D. Lambracht-Washington and R. N. Rosenberg, Transl. Neurosci., 2012, 3, 307–313 Search PubMed.
- J. Sevigny, P. Chiao, T. Bussière, P. H. Weinreb, L. Williams, M. Maier, R. Dunstan, S. Salloway, T. Chen, Y. Ling, J. O'Gorman, F. Qian, M. Arastu, M. Li, S. Chollate, M. S. Brennan, O. Quintero-Monzon, R. H. Scannevin, H. M. Arnold, T. Engber, K. Rhodes, J. Ferrero, Y. Hang, A. Mikulskis, J. Grimm, C. Hock, R. M. Nitsch and A. Sandrock, Nature, 2016, 537, 50 CrossRef PubMed.
- T. Blaettler, J. Smith, J. Smith, R. Paul, V. Asnaghi, R. Fuji, A. Quartino, L. Honigberg, M. A. Rabbia Jr, S. Yule, S. Ostrowitzki and P. Fontoura, Alzheimer's Dementia, 2016, 12, P609 CrossRef.
- M. Ultsch, B. Li, T. Maurer, M. Mathieu, O. Adolfsson, A. Muhs, A. Pfeifer, M. Pihlgren, T. W. Bainbridge, M. Reichelt, J. A. Ernst, C. Eigenbrot, G. Fuh, J. K. Atwal, R. J. Watts and W. Wang, Sci. Rep., 2016, 6, 39374 CrossRef PubMed.
- S. Ostrowitzki, D. Deptula and L. Thurfjell, et al., Arch. Neurol., 2012, 69, 198–207 CrossRef PubMed.
- R. Lai, V. Logovinsky, J. M. Kaplow, K. Gu, Y. Yu, C. Möller, B. Navia, C. Swanson, L. Lannfelt and A. Satlin, Alzheimer's Dementia, 2013, 9, P689 CrossRef.
- H. Feinberg, J. W. Saldanha, L. Diep, A. Goel, A. Widom, G. M. Veldman, W. I. Weis, D. Schenk and G. S. Basi, Alzheimer's Res. Ther., 2014, 6, 31 CrossRef PubMed.
- G. A. N. Crespi, S. J. Hermans, M. W. Parker and L. A. Miles, Sci. Rep., 2015, 5, 9649 CrossRef PubMed.
- J. Bright, S. Hussain, V. Dang, S. Wright, B. Cooper, T. Byun, C. Ramos, A. Singh, G. Parry, N. Stagliano and I. Griswold-Prenner, Neurobiol. Aging, 2015, 36, 693–709 CrossRef PubMed.
- K. Yanamandra, N. Kfoury, H. Jiang, T. E. Mahan, S. Ma, S. E. Maloney, D. F. Wozniak, M. I. Diamond and D. M. Holtzman, Neuron, 2013, 80, 402–414 CrossRef PubMed.
- M. Ufer, M.-L. Rouzade-Dominguez, G. Huledal, N. Pezous, A. Avrameas, O. David, S. Kretz, K. Kucher, U. Neumann, J.-H. Cha, A. Graf and C. Lopez-Lopez, Alzheimer's Dementia, 2016, 12, P200 CrossRef.
- R. Y. K. Lai, B. Darpo, S. Dayal, N. Hall, M.-K. Chang, B. Albala, J. Ferry and B. Rege, Alzheimer's Dementia, 2017, 13, P250–P251 CrossRef.
- R. Yan, Transl. Neurodegener., 2016, 5, 13 CrossRef PubMed.
- S. Eketjäll, J. Janson, K. Kaspersson, A. Bogstedt, F. Jeppsson, J. Fälting, S. B. Haeberlein, A. R. Kugler, R. C. Alexander and G. Cebers, J. Alzheimer's Dis., 2016, 50, 1109–1123 Search PubMed.
- M. E. Kennedy, A. W. Stamford, X. Chen, K. Cox, J. N. Cumming, M. F. Dockendorf, M. Egan, L. Ereshefsky, R. A. Hodgson, L. A. Hyde, S. Jhee, H. J. Kleijn, R. Kuvelkar, W. Li, B. A. Mattson, H. Mei, J. Palcza, J. D. Scott, M. Tanen, M. D. Troyer, J. L. Tseng, J. A. Stone, E. M. Parker and M. S. Forman, Sci. Transl. Med., 2016, 8, 363ra150 CrossRef PubMed.
- C. Venugopal, C. M. Demos, K. S. J. Rao, M. A. Pappolla and K. Sambamurti, CNS Neurol. Disord.: Drug Targets, 2008, 7, 278–294 CrossRef.
- L. Rajendran, A. Schneider, G. Schlechtingen, S. Weidlich, J. Ries, T. Braxmeier, P. Schwille, J. B. Schulz, C. Schroeder, M. Simons, G. Jennings, H.-J. Knölker and K. Simons, Science, 2008, 320, 520–523 CrossRef PubMed.
- R. S. Doody, R. Raman, M. Farlow, T. Iwatsubo, B. Vellas, S. Joffe, K. Kieburtz, F. He, X. Sun, R. G. Thomas, P. S. Aisen, E. Siemers, G. Sethuraman and R. Mohs, N. Engl. J. Med., 2013, 369, 341–350 CrossRef PubMed.
- V. Coric, C. H. van Dyck and S. Salloway, et al., Arch. Neurol., 2009, 69, 1430–1440 CrossRef PubMed.
- H. Grossman, G. Marzloff, X. Luo, D. LeRoith, M. Sano and G. Pasinetti, Alzheimer's Dementia, 5, P259 CrossRef.
- X. Zhang, Y. Li, H. Xu and Y.-w. Zhang, Front. Cell. Neurosci., 2014, 8, 427 Search PubMed.
- V. Tell and A. Hilgeroth, Front. Cell. Neurosci., 2013, 7, 189 Search PubMed.
- P. J. Dolan and G. V. W. Johnson, Curr. Opin. Drug Discovery Dev., 2010, 13, 595–603 Search PubMed.
- J. M. Domínguez, A. Fuertes, L. Orozco, M. del Monte-Millán, E. Delgado and M. Medina, J. Biol. Chem., 2012, 287, 893–904 CrossRef PubMed.
- S.-L. Liu, C. Wang, T. Jiang, L. Tan, A. Xing and J.-T. Yu, Mol. Neurobiol., 2016, 53, 4328–4342 CrossRef PubMed.
- M. N. Sabbagh, A. Agro, J. Bell, P. S. Aisen, E. Schweizer and D. Galasko, Alzheimer Dis. Assoc. Disord., 2011, 25, 206–212 CrossRef PubMed.
- L.-F. Lue, D. G. Walker, S. Jacobson and M. Sabbagh, Future Neurol., 2009, 4, 167–177 CrossRef PubMed.
- S. Schilling, U. Zeitschel, T. Hoffmann, U. Heiser, M. Francke, A. Kehlen, M. Holzer, B. Hutter-Paier, M. Prokesch, M. Windisch, W. Jagla, D. Schlenzig, C. Lindner, T. Rudolph, G. Reuter, H. Cynis, D. Montag, H.-U. Demuth and S. Rossner, Nat. Med., 2008, 14, 1106 CrossRef PubMed.
- J. L. Frost, K. X. Le, H. Cynis, E. Ekpo, M. Kleinschmidt, R. M. Palmour, F. R. Ervin, S. Snigdha, C. W. Cotman, T. C. Saido, R. J. Vassar, P. S. George-Hyslop, T. Ikezu, S. Schilling, H.-U. Demuth and C. A. Lemere, Am. J. Pathol., 2013, 183, 369–381 CrossRef PubMed.
- T. Hoffmann, A. Meyer, U. Heiser, S. Kurat, L. Böhme, M. Kleinschmidt, K.-U. Bühring, B. Hutter-Paier, M. Farcher, H.-U. Demuth, I. Lues and S. Schilling, J. Pharmacol. Exp. Ther., 2017, 362, 119–130 CrossRef PubMed.
- Y. Hori, S. Takeda, H. Cho, S. Wegmann, T. M. Shoup, K. Takahashi, D. Irimia, D. R. Elmaleh, B. T. Hyman and E. Hudry, J. Biol. Chem., 2015, 290, 1966–1978 CrossRef PubMed.
- J. Budni, M. L. Garcez, J. d. Medeiros, E. Cassaro, T. Bellettini-Santos, F. Mina and J. Quevedo, Curr. Alzheimer Res., 2016, 13, 1319–1329 CrossRef PubMed.
- S. M. Roy, V. L. Grum-Tokars, J. P. Schavocky, F. Saeed, A. Staniszewski, A. F. Teich, O. Arancio, A. D. Bachstetter, S. J. Webster, L. J. Van Eldik, G. Minasov, W. F. Anderson, J. C. Pelletier and D. M. Watterson, ACS Chem. Neurosci., 2015, 6, 666–680 CrossRef PubMed.
- J. J. Alam, J. Alzheimer's Dis., 2015, 48, 219–227 Search PubMed.
- T. Alkam, A. Nitta, H. Mizoguchi, K. Saito, M. Seshima, A. Itoh, K. Yamada and T. Nabeshima, Behav. Brain Res., 2008, 189, 100–106 CrossRef PubMed.
- C. Holmes, C. Cunningham, E. Zotova, D. Culliford and V. H. Perry, Neurology, 2011, 77, 212–218 CrossRef PubMed.
- J. Butchart, L. Brook, V. Hopkins, J. Teeling, U. Püntener, D. Culliford, R. Sharples, S. Sharif, B. McFarlane, R. Raybould, R. Thomas, P. Passmore, V. H. Perry and C. Holmes, Neurology, 2015, 84, 2161–2168 CrossRef PubMed.
- A. Maelicke, A. Hoeffle-Maas, J. Ludwig, A. Maus, M. Samochocki, U. Jordis and A. K. E. Koepke, J. Mol. Neurosci., 2010, 40, 135–137 CrossRef PubMed.
- R. S. Bitner, S. Markosyan, A. L. Nikkel and J. D. Brioni, Neuropharmacology, 2011, 60, 460–466 CrossRef PubMed.
- X. Song, M. O. Jensen, V. Jogini, R. A. Stein, C.-H. Lee, H. S. McHaourab, D. E. Shaw and E. Gouaux, Nature, 2018, 556, 515–519 CrossRef PubMed.
|
This journal is © The Royal Society of Chemistry 2018 |
Click here to see how this site uses Cookies. View our privacy policy here.