DOI:
10.1039/C8QM00439K
(Research Article)
Mater. Chem. Front., 2018,
2, 2327-2332
An excellent cryogenic magnetic cooler: magnetic and magnetocaloric study of an inorganic frame material†
Received
29th August 2018
, Accepted 29th September 2018
First published on 1st October 2018
Abstract
The cryogenic magnetic coolers based on the magnetocaloric effect (MCE) of paramagnetic compounds usually need relatively large fields to realise a practical cooling performance. Thus, it is of significance to search for molecule-based cryogenic magnetic coolers with high performance of the MCE under low fields (smaller than 2 T), considering the ready availability of low fields by commercial Nd–Fe–B permanent magnets. In this work, the crystal structure, magnetic susceptibility and isothermal magnetization for the inorganic compound Gd(OH)SO4 (1) have been investigated. The title compound exhibits a 3D structure with Gd-oxygen chains as the supramolecular building units. Magnetic characterisations indicated that adjacent GdIII ions show weak magnetic couplings in 1. Because of the integration of large metal/ligand mass ratio, weak magnetic couplings and a dense framework, the observed maximum entropy change (−ΔSmaxm) for 1 was up to 53.5 J kg−1 K−1 or 276 mJ cm−3 K−1 for ΔH = 7 T and T = 2 K, comparable to the performance of commercial gadolinium gallium garnet Gd3Ga5O12 (GGG, −ΔSmaxm = 38.4 J kg−1 K−1 or 272 mJ cm−3 K−1 with ΔH = 7 T). Notably, the −ΔSmaxm of 1 still reaches 27.5 J kg−1 K−1 at T = 2 K and ΔH = 2 T, and 38.3 J kg−1 K−1 at T = 2 K and ΔH = 3 T, which already surpasses GGG (about 24 J kg−1 K−1 with ΔH = 3 T) and makes 1 a superior cryogenic magnetic cooler for low-temperature applications, even in low fields.
Introduction
The changes of the isothermal magnetic entropy and adiabatic temperature of a solid material induced by changes in the applied magnetic field are defined as the magnetocaloric effect (MCE), which was first discovered by Warburg in metallic iron in 1881 and can be used to attain ultra-low temperature by adiabatic demagnetization.1 Cryogenic magnetic cooling based on the MCE has been considered as an environmentally friendly and energy-saving technique.2 Recently, molecular cryogenic magnetic coolers have caught the eye of researchers due to their intrinsic characteristics such as stoichiometric composition, monodispersity, modifiability, etc.3
Four effective strategies have been proposed by Tong and coworkers to design and synthesize optimized molecule-based cryogenic magnetic coolers, including boosting ground-state spin, lowering magnetic anisotropy, weakening magnetic interactions, and reducing the molecular weight.3a Thus, the sound assembly of paramagnetic metal ions and bridging ligands directed by the previous lessons and strategies play a vital role in the cooling performance of the resultant compounds.3 Hitherto, most of the explored molecule-based cryogenic magnetic coolers are Gd-based clusters and coordination polymers (CPs),3 which is mainly ascribable to the characteristics of the GdIII ion (large spin ground state, magnetic isotropy and weak exchange coupling).4,5 Among the various organic ligands used, carboxylate ligands are broadly studied.3,6 Coordination-driven assembly of the GdIII ion and light carboxylate ligands has given birth to a series of impressive Gd-based magneto-coolants, examplified by 3D [Gd(HCOO)3] (−ΔSmaxm = 55.9 J kg−1 K−1 or 216 mJ cm−3 K−1 with ΔH = 7 T),7a 2D [Gd2(C2O4)3(H2O)6·0.6H2O] (−ΔSmaxm = 46.6 J kg−1 K−1 or 118 mJ cm−3 K−1 with ΔH = 7 T),7b 2D [Gd(C2O4)(H2O)3Cl] (−ΔSmaxm = 48.0 J kg−1 K−1 or 144 mJ cm−3 K−1 with ΔH = 7 T),7c and 1D [Gd(HCOO)(OAc)2(H2O)2] (−ΔSmaxm = 45.9 J kg−1 K−1 or 110 mJ cm−3 K−1 with ΔH = 7 T).7d
Paralleling the extensively studied organic ligands, the light inorganic ligands are also promising candidates for constructing Gd-based magnetic cryocoolants bearing large MCE in gravimetric units (J kg−1 K−1) and volumetric units (mJ cm−3 K−1) thanks to the weak exchange interactions and dense frame in the resulting compounds.8,9 The shielded 4f orbitals of GdIII make the GdIII–GdIII magnetic coupling usually weak, even if the ligand is a bridging-oxygen atom, which is well corroborated in Gd(OH)3 (−ΔSmaxm = 62.0 kg−1 K−1 or 346 mJ cm−3 K−1 with ΔH = 7 T) and Gd2O(OH)4(H2O)2 (−ΔSmaxm = 59.1 J kg−1 K−1 or 217 mJ cm−3 K−1 with ΔH = 7 T).8a Furthermore, the inorganic Gd-based magnetic coolants usually display good chemical and thermal stability.8b,c The effectiveness and efficiency of inorganic ligands to fabricate ideal Gd-based cryogenic magnetic coolers has been well testified in publications.8,9 Representative examples include the early investigated Gd2(SO4)3·8H2O,9a,b recently reported GdF3 (−ΔSmaxm = 71.6 J kg−1 K−1 or 506 mJ cm−3 K−1 with ΔH = 7 T),9c GdPO4 (−ΔSmaxm = 62.0 J kg−1 K−1 or 376 mJ cm−3 K−1 with ΔH = 7 T),9d and K3Li3Gd7(BO3)9 (−ΔSmaxm = 56.6 J kg−1 K−1 or 278 mJ cm−3 K−1 with ΔH = 7 T).9e Different from the Gd-based molecular magnetic coolers supported by organic ligands, their counterparts like Gd2(SO4)3·8H2O, Gd(OH)3, GdF3 and GdPO4 have usually been known for many years, which gives us a hint that utilising known results is also an avenue to explore brilliant molecular cryogenic magnetic coolers under the guidance of molecular design strategies.
Herein, we report the structure, magnetic susceptibility and isothermal magnetization of an inorganic frame material with the formula of Gd(OH)SO4. This material features weak magnetic exchange, low molecular weight (270.32 g mol−1) and dense frame structure (5.163 g cm−3), which is helpful for boosting the maximum entropy change (−ΔSmaxm). The −ΔSmaxm derived from isothermal magnetization is up to 53.5 J kg−1 K−1 or 276 mJ cm−3 K−1, comparable to the performance of commercial gadolinium gallium garnet Gd3Ga5O12 (GGG, −ΔSmaxm = 38.4 J kg−1 K−1 or 272 mJ cm−3 K−1 with ΔH = 7 T) and its derivative Gd3(Ga1−xFex)5O12 (GGIG).10 It is notable that the −ΔSmaxm of 1 still reaches 27.5 J kg−1 K−1 (T = 2 K and ΔH = 2 T) and 38.3 J kg−1 K−1 (T = 2 K and ΔH = 3 T) even in low fields, which already surpasses GGG (about 24 J kg−1 K−1 with ΔH = 3 T). All these magnetic characteristics make 1 an outstanding cryogenic magnetic cooler.
Experimental
Materials and instrumentation
All chemicals were analytical grade and used directly. IR data were collected on a MAGNA-560 (Nicolet) FT-IR spectrometer with KBr as pellets. Experimental powder X-ray diffraction (PXRD) data were collected on a Bruker D8 FOCUS diffractometer with a Cu-target tube and a graphite monochromator. Simulated PXRD data were obtained from the single crystal X-ray diffraction (SCXRD) data via the Mercury program. Thermogravimetric (TG) data were collected on a Rigaku Thermo plus EVO2 TG-DTA8121 analyzer. The magnetic data were collected using a MPMS XL-5 SQUID magnetometer. The diamagnetic corrections were finished with Pascal's constants.
Synthesis of Gd(OH)SO4 (1).
1 could be prepared according to the literature procedure.111 could also be synthesized by the following method. A mixture of Gd2(SO4)3·8H2O (0.075 g, 0.10 mmol), H2C2O4·2H2O (0.189 g, 1.50 mmol), urea (0.015 g, 0.25 mmol) and H2O (10 mL) was sealed in a Teflon-lined autoclave (20 mL) and heated to 180 °C for 7 days and then slowly cooled to ambient temperature. Yield: ca. 15% based on H2C2O4·2H2O. IR (cm−1): 3480(s), 3425(s), 2970(m), 2917(w), 1621(s), 1382(w), 1172(s), 1087(s), 990(s), 867(s), 773(s), and 600(s).
Crystallographic data collection and refinement
The SCXRD data were collected on a XtaLAB-mini diffractometer at 293(2) K with Mo-Kα radiation (λ = 0.71073 Å) by ω scan mode. SHELX-2016 software was used to solve the frame of 1.12 Detailed crystallographic data are presented in Table 1 and the selected bond lengths and angles are given in Table S1 (ESI†). Further details on the structural investigation is available from the Fachinformationszentrum Karlsruhe, 76344 Eggenstein-Leopoldshafen, Germany (fax: (+49) 7247-808-666; e-mail: crysdata@fiz-karlsruhe.de), on quoting the depository number CSD-261284 (Gd(OH)SO4).
Table 1 Crystal data and structural refinement for Gd(OH)SO4
Chemical formula |
Gd(OH)SO4 |
Formula mass/g mol−1 |
270.32 |
T/K |
293(2) |
λ/Å |
0.71073 |
Crystal system |
Monoclinic |
Space group |
P21/n |
a/Å |
4.3950(4) |
b/Å |
12.2030(8) |
c/Å |
6.7606(6) Å |
β/° |
106.434(9) |
V/Å3 |
347.77(5) |
Z
|
4 |
D
c/g cm−3 |
5.163 |
μ/mm−1 |
19.547 |
F(000) |
484 |
Total reflns |
1144 |
Unique reflns |
612 |
R
int
|
0.0254 |
Final R indices [I > 2σ(I)] |
R
1 = 0.0308, wR2 = 0.0817 |
R indices (all data) |
R
1 = 0.0320, wR2 = 0.0833 |
GOF on F2 |
1.054 |
Results and discussion
Description of crystal structures
Because the structure of 1 has been reported,11 the structural delineations are introduced briefly. SCXRD analysis indicates that compound 1 crystallizes in the monoclinic space group of P21/n. The asymmetric unit of 1 has one crystallographically independent Gd atom, one sulfate (SO42−), and one hydroxy anion (OH−). As displayed in Fig. 1a, all Gd atoms are in the [GdO9] capped square antiprism geometry. The SO42− groups have the Harris notation of [6.2211] to link six Gd atoms,13 while the OH− moieties exhibit μ3 mode to link three Gd atoms. The presence of sulfate and OH− moieties in the frame has also been confirmed by their corresponding characteristic IR peaks (3480, 867, 773 cm−1 for OH− and 1172 cm−1 for sulfate) (Fig. S1, ESI†). The μ3-OH− together with the η2-O-atom from sulfate connect symmetry-related Gd-ions to form the 1D Gd-oxygen chain (Fig. 1b), which is further connected to the other η2-O-atom of sulfate to generate the 2D layer (Fig. 1c). The adjacent 2D layers connect with each other via the η1-O-atom of sulfate to give rise to the resultant 3D dense framework (Fig. 1d).
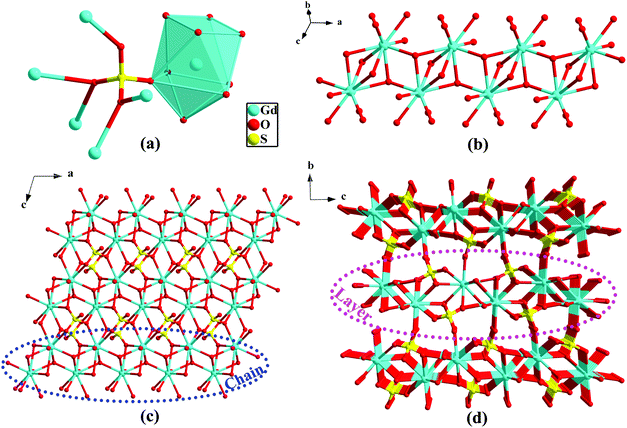 |
| Fig. 1 (a) The coordination environments of sulfate and Gd atoms; (b) the 1D Gd-oxygen chain; (c) the 1D Gd-oxygen chain connected by sulfate to form the 2D layer; (d) the 2D layer linked by an additional O-atom of sulfate to generate the 3D structure. | |
TG analysis for 1 was measured under air atmosphere between 25 and 1000 °C (10 °C min−1). As illustrated in Fig. S2 (ESI†), no apparent weight loss was observable from ambient temperature to 600 °C, implying good thermal stability of 1. An apparent weight loss is present upon further heating of the sample above 610 °C, indicating the collapse of the framework. To confirm the phase purities before property measurements, PXRD was conducted. Fig. S3 (ESI†) illustrates that the positions of the experimental peak values are in accordance with the fitted ones derived from SCXRD, suggesting the purity of the samples. The distinct intensities between the peaks may be attributable to the variation in the preferred orientation of the experimental sample.
Magnetic properties
The mole magnetic susceptibility (χM) of 1 was investigated in the solid state in the temperature range of 2–300 K at 1 kOe field. All magnetic measurements were obtained with crushed crystalline samples. The observed χMT product of 8.04 cm3 K mol−1 at 300 K basically matches with the value for one isolated GdIII (7.88 cm3 K mol−1, 8S7/2, g = 2) in 1 (Fig. 2a). During the process of cooling, the χMT products of 1 decline very slowly from 300 K to 10 K. Below 10 K, an apparent fall was present with the minimum value of 7.51 cm3 K mol−1 at 2 K. The declining trend of the χMT vs. T plot for 1 manifests that adjacent GdIII ions feature antiferromagnetic (AF) coupling, which is further corroborated by the negative Weiss constant θ = −0.22 K from Curie–Weiss simulation (Fig. 2a). The accordance between zero field cooled (ZFC) and field cooled (FC) magnetizations excluded the possibilities of long-range magnetic ordering (Fig. 2b).
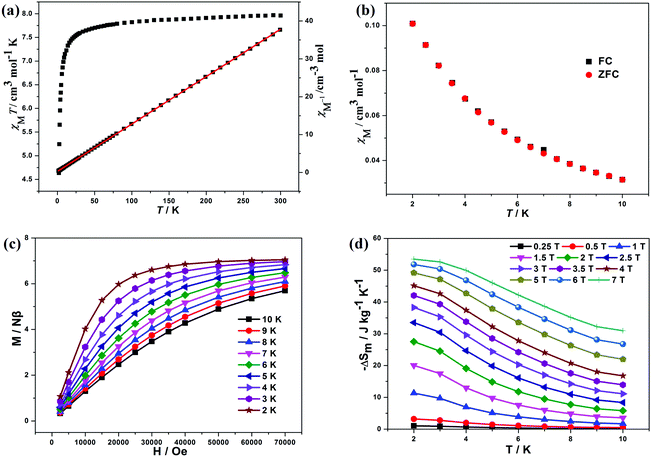 |
| Fig. 2 (a) The plot of χMT vs. T for 1 (red part for the Curie–Weiss simulation). (b) FC and ZFC magnetization of 1 in the dc field of 50 Oe. (c) Variable-field magnetization plots at specific temperatures for 1. (d) −ΔSm derived from experimental magnetization data of 1 at distinct fields and temperatures. | |
The variable-field magnetization (M) characterizations were finished in the scope of 0–7 T at 2–10 K for 1 (Fig. 2c). The curves of M vs. H show a gradual increase with the increasing fields. The M is 7.41 Nβ at 2 K and 7 T, basically agreeing with the theoretical value of 7.0 Nβ for one GdIII (g = 2, S = 7/2). The weak magnetic exchange interactions, together with high metal/ligand mass ratio, suggest that compound 1 holds great promise as a cryogenic magnetorefrigerant. As a vital factor in appraising the MCE, magnetic entropy change −ΔSm can be inferred from experimental magnetization data via Maxwell equation
.3 The magnetic fields and temperature-dependent entropy changes are presented in Fig. 2d, with the maximum −ΔSm (−ΔSmaxm) up to 53.5 J kg−1 K−1 at 2 K with ΔH = 7 T. The experimental −ΔSmaxm is lower than the theoretical one of 64.0 J kg−1 K−1 for one free GdIII in the chemical formula (judged by nGdR
ln(2SGd + 1)), which may be ascribable to the global AF behaviours in 1. Among reported GdIII-based molecular magnetic cryocoolers, −ΔSmaxm above 40.0 J kg−1 K−1 is limited.3,4a The −ΔSmaxm for 1 is 276 mJ cm−3 K−1 from the volumetric aspect, which is still very competitive among the GdIII-containing complexes.3,4a Compared with the sulfate-based molecular cryogenic magnetic coolers (Table 2), the maximum MCE per unit mass of 1 (53.5 J kg−1 K−1) is slightly larger than those reported for (3,12)-connected [Gd4] cluster-based compound [Gd4(SO4)4(OH)4(H2O)4] (51.3 J kg−1 K−1),8b (3,11)-connected [Gd4] cluster-based compound [Gd4(SO4)3(OH)4(C2O4)(H2O)5]·H2O (51.5 J kg−1 K−1),5e and 3D [Gd(HCOO)(SO4)(H2O)] (49.9 J kg−1 K−1)14 because of the synergetic effect of weak magnetic interactions and small molecular weight. However, due to the dense frame characteristic, the −ΔSmaxm per unit volume of 1 (276 mJ cm−3 K−1) is obviously higher than the related ones with 199 mJ cm−3 K−1 for the Gd-sulfate system,8b 190 mJ cm−3 K−1 for the Gd-sulfate-oxalate system,5e and 190 mJ cm−3 K−1 for the Gd-sulfate-formate system.13 It is, indeed, more significant for application when appraising the cryogenic magnetorefrigerants from the point of volumetric unit.15 Notably, the −ΔSmaxm of 1 is larger than most of the reported GdIII-based molecular magnetic coolers fabricated by organic ligands (Table 2), implying the efficiency of light inorganic ligands in the synthesis of attractive molecular magneto-cryocoolants. The high −ΔSmaxm of 1 is mainly due to the weak magnetic coupling between adjacent GdIII ions, small molecular weight and dense framework characteristics. Furthermore, the −ΔSm per unit mass still reaches the values of 27.5 J kg−1 K−1 (T = 2 K and ΔH = 2 T) and 38.3 J kg−1 K−1 (T = 2 K and ΔH = 3 T) even in lower fields, which already surpasses the performances of commercial gadolinium gallium garnet Gd3Ga5O12 (GGG) (about 24 J kg−1 K−1 with ΔH = 3 T)10 and makes 1 a promising cryocoolant for potential application, considering the ready availability of low fields by commercial Nd–Fe–B permanent magnets.3f,17
Table 2 Comparison of −ΔSmaxm (larger than 40.0 J kg−1 K−1) between 1 and sulfate-based molecular cryogenic magnetic coolers together with some selected Gd-complexes fabricated by organic ligands
Complex |
D |
−ΔSmaxm |
[J kg−1 K−1] [mJ cm−3 K−1] |
−ΔSmaxm [mJ cm−3 K−1] = −ΔSmaxm [J kg−1 K−1]*ρcald [g cm−3] D: dimensionality. |
[Gd(HCOO)3]7a |
3D |
55.9 |
216 |
Gd(OH)SO4 (this work) |
3D |
53.5 |
276 |
[Gd4(SO4)3(OH)4(C2O4)(H2O)5]·H2O5e |
3D |
51.5 |
190 |
[Gd4(SO4)4(OH)4(H2O)4]8b |
3D |
51.3 |
199 |
[Gd(HCOO)(SO4)(H2O)]14 |
3D |
49.9 |
190 |
[Gd(C2O4)(H2O)3Cl]7c |
2D |
48.0 |
144 |
[Gd(OAc)3(H2O)0.5]16a |
1D |
47.7 |
106 |
[Gd(HCOO)(C8H4O4)]16b |
2D |
47.0 |
125 |
[Gd2(C2O4)3(H2O)6·0.6H2O]7b |
2D |
46.6 |
118 |
[Gd(HCOO)(OAc)2(H2O)2]7d |
1D |
45.9 |
110 |
[Gd(OAc)3(MeOH)]16a |
1D |
45.0 |
97 |
[Gd(C4O4)(C2O4)0.5(H2O)2]16c |
3D |
44.0 |
128 |
[Gd(cit)(H2O)]16d |
2D |
43.6 |
115 |
{[Gd(OAc)3(H2O)2]}2·4H2O16e |
0D |
41.6 |
83 |
{[Gd2(IDA)3]·2H2O}16f |
3D |
40.6 |
101 |
Conclusions
The structure, magnetic susceptibility and isothermal magnetizations for the inorganic Gd-based coordination polymer (CP) Gd(OH)SO4 (1) have been investigated. The title CP exhibits the 3D dense architecture with a Gd-oxygen chain as supramolecular building units. Magnetic characterisations indicated that adjacent GdIII ions show weak magnetic exchange in 1. Because of the integration of the large isotropic spin of GdIII, the dense structure and weak magnetic couplings, the observed maximum entropy change (−ΔSmaxm) for 1 was 53.5 J kg−1 K−1 for ΔH = 7 T and T = 2 K and 38.3 J kg−1 K−1 for T = 2 K and ΔH = 3 T, which already surpasses the commercial gadolinium gallium garnet Gd3Ga5O12 (GGG) (38.4 J kg−1 K−1 with ΔH = 7 T and about 24 J kg−1 K−1 with ΔH = 3 T), indicating it to be an excellent cryogenic magnetic cooler. This work highlights the role of inorganic ligands in the generation of excellent molecular cryogenic magnetic coolers. Further work will focus on utilising other known results to explore brilliant molecular cryogenic magnetic coolers under the guidance of molecular design strategies.
Conflicts of interest
There are no conflicts to declare.
Acknowledgements
This work was supported by the Natural Science Foundation of China (21571111, 21601099 and 21601100), the China Postdoctoral Science Foundation (2016M592137), the Young Scientist Foundation of Shandong Province of China (ZR2016BB25), and the project of applied and fundamental research of Qingdao City of China (16-5-1-87-jch).
Notes and references
-
(a) E. Warburg, Ann. Phys., 1881, 249, 141–164 CrossRef;
(b) P. Debye, Ann. Phys., 1926, 386, 1154–1160 CrossRef;
(c) W. F. Giauque, J. Am. Chem. Soc., 1927, 49, 1864–1870 CrossRef CAS.
-
(a) M. Evangelisti and E. K. Brechin, Dalton Trans., 2010, 39, 4672–4676 RSC;
(b) R. Sessoli, Angew. Chem., Int. Ed., 2012, 51, 43–45 CrossRef CAS PubMed;
(c) O. Gutfleisch, M. A. Willard, E. Brück, C. H. Chen, S. G. Sankar and J. P. Liu, Adv. Mater., 2011, 23, 821–842 CrossRef CAS PubMed;
(d) X.-Y. Zheng, X.-J. Kong, Z. Zheng, L.-S. Long and L.-S. Zheng, Acc. Chem. Res., 2018, 51, 517–525 CrossRef CAS PubMed;
(e) S. Fan, S.-H. Xu, X.-Y. Zheng, Z.-H. Yan, X.-J. Kong, L.-S. Long and L.-S. Zheng, CrystEngComm, 2018, 20, 2120–2125 RSC;
(f) Y. Zhou, X.-Y. Zheng, J. Cai, Z.-F. Hong, Z.-H. Yan, X.-J. Kong, Y.-P. Ren, L.-S. Long and L.-S. Zheng, Inorg. Chem., 2017, 56, 2037–2041 CrossRef CAS PubMed;
(g) X.-Y. Zheng, Y.-H. Jiang, G.-L. Zhuang, D.-P. Liu, H.-G. Liao, X.-J. Kong, L.-S. Long and L.-S. Zheng, J. Am. Chem. Soc., 2017, 139, 18178–18181 CrossRef CAS PubMed;
(h) X.-Y. Zheng, S.-Q. Wang, W. Tang, G.-L. Zhuang, X.-J. Kong, Y.-P. Ren, L.-S. Long and L.-S. Zheng, Chem. Commun., 2015, 51, 10687–10690 RSC.
-
(a) J.-L. Liu, Y.-C. Chen and M.-L. Tong, Chem. Rec., 2016, 16, 825–834 CrossRef CAS PubMed;
(b) S. Zhang and P. Cheng, Chem. Rec., 2016, 16, 2077–2126 CrossRef CAS PubMed;
(c) M. Evangelisti, F. Luis, L. J. de Jongh and M. Affronte, J. Mater. Chem., 2006, 16, 2534–2549 RSC;
(d) Y.-Z. Zheng, G.-J. Zhou, Z. Zheng and R. E. P. Winpenny, Chem. Soc. Rev., 2014, 43, 1462–1475 RSC;
(e) J. W. Sharples and D. Collison, Polyhedron, 2013, 54, 91–103 CrossRef CAS;
(f) J.-L. Liu, Y.-C. Chen, F.-S. Guo and M.-L. Tong, Coord. Chem. Rev., 2014, 281, 26–49 CrossRef CAS;
(g) J.-P. Zhao, S.-D. Han, X. Jiang, S.-J. Liu, R. Zhao, Z. Chang and X.-H. Bu, Chem. Commun., 2015, 51, 8288–8291 RSC;
(h) J.-P. Zhao, R. Zhao, Q. Yang, B.-W. Hu, F.-C. Liu and X.-H. Bu, Dalton Trans., 2013, 42, 14509–14515 RSC.
-
(a) S.-J. Liu, X.-R. Xie, T.-F. Zheng, J. Bao, J.-S. Liao, J.-L. Chen and H.-R. Wen, CrystEngComm, 2015, 17, 7270 RSC;
(b) T.-Q. Song, J. Dong, A.-F. Yang, X.-J. Che, H.-L. Gao, J.-Z. Cui and B. Zhao, Inorg. Chem., 2018, 57, 3144–3150 CrossRef CAS PubMed;
(c) D. Peng, L. Yin, P. Hu, B. Li, Z.-W. Ouyang, G.-L. Zhuang and Z. Wang, Inorg. Chem., 2018, 57, 2577–2583 CrossRef CAS PubMed;
(d) W. Huang, S. Huang, M. Zhang, Y. Chen, G.-L. Zhuang, Y. Li, M.-L. Tong, J. Yong, Y. Li and D. Wu, Chem. Commun., 2018, 54, 4104–4107 RSC;
(e) S. Fan, S.-H. Xu, X.-Y. Zheng, Z.-H. Yan, X.-J. Kong, L.-S. Long and L. Zheng, CrystEngComm, 2018, 20, 2120–2125 RSC;
(f) K. Wang, Z.-L. Chen, H.-H. Zou, K. Hu, H.-Y. Li, Z. Zhang, W.-Y. Sun and F.-P. Liang, Chem. Commun., 2016, 52, 8297–8300 RSC.
-
(a) K. Wang, Z.-L. Chen, H.-H. Zou, S.-H. Zhang, Y. Li, X.-Q. Zhang, W.-Y. Sun and F.-P. Liang, Dalton Trans., 2018, 47, 2337–2343 RSC;
(b) H.-M. Chen, W.-M. Wang, X.-Q. Li, X.-Y. Chu, Y.-Y. Nie, Z. Liu, S.-X. Huang, H.-Y. Shen, J.-Z. Cui and H.-L. Gao, Inorg. Chem. Front., 2018, 5, 394–402 RSC;
(c) P.-F. Shi, C.-S. Cao, C.-M. Wang and B. Zhao, Inorg. Chem., 2017, 56, 9169–9176 CrossRef CAS PubMed;
(d) S.-D. Han, J.-H. Li, H.-H. Liu and G.-M. Wang, Dalton Trans., 2017, 46, 10023–10028 RSC;
(e) S.-D. Han, X.-H. Miao, S.-J. Liu and X.-H. Bu, Chem. – Asian J., 2014, 9, 3116–3120 CrossRef CAS PubMed.
-
(a) S.-J. Liu, C. Cao, S.-L. Yao, T.-F. Zheng, Z.-X. Wang, C. Liu, J.-S. Liao, J.-L. Chen, Y.-W. Li and H.-R. Wen, Dalton Trans., 2017, 46, 64–70 RSC;
(b) X.-Y. Zheng, J.-B. Peng, X.-J. Kong, L.-S. Long and L.-S. Zheng, Inorg. Chem. Front., 2016, 3, 320–325 RSC;
(c) S.-J. Liu, C. Cao, C.-C. Xie, T.-F. Zheng, X.-L. Tong, J.-S. Liao, J.-L. Chen, H.-R. Wen, Z. Chang and X.-H. Bu, Dalton Trans., 2016, 45, 9209–9215 RSC;
(d) W.-P. Chen, P.-Q. Liao, Y. Yu, Z. Zheng, X.-M. Chen and Y.-Z. Zheng, Angew. Chem., Int. Ed., 2016, 55, 9375–9379 CrossRef CAS PubMed;
(e) J.-B. Peng, X.-J. Kong, Q.-C. Zhang, M. Orendáč, J. Prokleška, Y.-P. Ren, L.-S. Long, Z. Zheng and L.-S. Zheng, J. Am. Chem. Soc., 2014, 136, 17938–17941 CrossRef CAS PubMed;
(f) S.-J. Liu, J.-P. Zhao, J. Tao, J.-M. Jia, S.-D. Han, Y. Li, Y.-C. Chen and X.-H. Bu, Inorg. Chem., 2013, 52, 9163–9165 CrossRef CAS PubMed;
(g) J.-B. Peng, Q.-C. Zhang, X.-J. Kong, Y.-Z. Zheng, Y.-P. Ren, L.-S. Long, R.-B. Huang, L.-S. Zheng and Z. Zheng, J. Am. Chem. Soc., 2012, 134, 3314–3317 CrossRef CAS PubMed;
(h) J.-B. Peng, Q.-C. Zhang, X.-J. Kong, Y.-P. Ren, L.-S. Long, R.-B. Huang, L.-S. Zheng and Z. Zheng, Angew. Chem., Int. Ed., 2011, 50, 10649–10652 CrossRef CAS PubMed.
-
(a) G. Lorusso, J. W. Sharples, E. Palacios, O. Roubeau, E. K. Brechin, R. Sessoli, A. Rossin, F. Tuna, E. J. L. McInnes, D. Collison and M. Evangelisti, Adv. Mater., 2013, 25, 4653–4656 CrossRef CAS PubMed;
(b) R. Sibille, E. Didelot, T. Mazet, B. Malaman and M. François, APL Mater., 2014, 2, 124402 CrossRef;
(c) Y. Meng, Y.-C. Chen, Z.-M. Zhang, Z.-J. Lin and M.-L. Tong, Inorg. Chem., 2014, 53, 9052–9057 CrossRef CAS PubMed;
(d) G. Lorusso, M. A. Palacios, G. S. Nichol, E. K. Brechin, O. Roubeau and M. Evangelisti, Chem. Commun., 2012, 48, 7592–7594 RSC.
-
(a) Y. Yang, Q.-C. Zhang, Y.-Y. Pan, L.-S. Long and L.-S. Zheng, Chem. Commun., 2015, 51, 7317–7320 RSC;
(b) S.-D. Han, X.-H. Miao, S.-J. Liu and X.-H. Bu, Inorg. Chem. Front., 2014, 1, 549–552 RSC;
(c) Y.-L. Hou, G. Xiong, P.-F. Shi, R.-R. Cheng, J.-Z. Cui and B. Zhao, Chem. Commun., 2013, 49, 6066–6068 RSC;
(d) Y.-C. Chen, L. Qin, Z.-S. Meng, D.-F. Yang, C. Wu, Z. Fu, Y.-Z. Zheng, J.-L. Liu, R. Tarasenko, M. Orendac, J. Prokleska, V. Sechovsky and M.-L. Tong, J. Mater. Chem. A, 2014, 2, 9851–9858 RSC.
-
(a) W. F. Giauque and D. P. MacDougall, Phys. Rev., 1933, 43, 768 CrossRef CAS;
(b) W. F. Giauque and D. P. MacDougall, J. Am. Chem. Soc., 1935, 57, 1175–1185 CrossRef CAS;
(c) Y.-C. Chen, J. Prokleska, W.-J. Xu, J.-L. Liu, J. Liu, W.-X. Zhang, J.-H. Jia, V. Sechovsky and M.-L. Tong, J. Mater. Chem. C, 2015, 3, 12206–12211 RSC;
(d) E. Palacios, J. A. Rodríguez-Velamazán, M. Evangelisti, G. J. McIntyre, G. Lorusso, D. Visser, L. J. de Jongh and L. A. Boatner, Phys. Rev. B: Condens. Matter Mater. Phys., 2014, 90, 214423 CrossRef;
(e) M. Xia, S. Shen, J. Lu, Y. Sun and R. Li, Chem. – Eur. J., 2018, 24, 3147–3150 CrossRef CAS PubMed.
- B. Baudun, R. Lagnier and B. Salce, J. Magn. Magn. Mater., 1982, 27, 315–322 CrossRef.
- R. A. Zehnder, C. S. Wilson, H. T. Christy, K. S. Harris, V. Chauhan, V. Schutz, M. Sullivan, M. Zeller, F. R. Fronczek, J. A. Myers, K. Dammann, J. Duck, P. M. Smith, A. Okuma, K. Johnson, R. Sovesky, C. Stroudt and R. A. Renn, Inorg. Chem., 2011, 50, 836–846 CrossRef CAS PubMed.
- G. M. Sheldrick, Acta Crystallogr., Sect. C: Struct. Chem., 2015, 71, 3–8 Search PubMed.
- R. A. Coxall, S. G. Harris, D. K. Henderson, S. Parsons, P. A. Tasker and R. E. P. Winpenny, J. Chem. Soc., Dalton Trans., 2000, 2349–2356 RSC.
- L.-Y. Xu, J.-P. Zhao, T. Liu and F.-C. Liu, Inorg. Chem., 2015, 54, 5249–5256 CrossRef CAS PubMed.
-
(a) K. A. Gschneidner Jr., V. K. Pecharsky and A. O. Tsokol, Rep. Prog. Phys., 2005, 68, 1479–1539 CrossRef;
(b) K. A. Gschneidner Jr. and V. K. Pecharsky, Annu. Rev. Mater. Sci., 2000, 30, 387–429 CrossRef.
-
(a) F.-S. Guo, J.-D. Leng, J.-L. Liu, Z.-S. Meng and M.-L. Tong, Inorg. Chem., 2012, 51, 405–413 CrossRef CAS PubMed;
(b) R. Sibille, T. Mazet, B. Malaman and M. François, Chem. – Eur. J., 2012, 18, 12970–12973 CrossRef CAS PubMed;
(c) S. Biswas, H. S. Jena, A. Adhikary and S. Konar, Inorg. Chem., 2014, 53, 3926–3928 CrossRef CAS PubMed;
(d) S.-J. Liu, C.-C. Xie, J.-M. Jia, J.-P. Zhao, S.-D. Han, Y. Cui, Y. Li and X.-H. Bu, Chem. – Asian J., 2014, 9, 1116–1122 CrossRef CAS PubMed;
(e) M. Evangelisti, O. Roubeau, E. Palacios, A. Camón, T. N. Hooper, E. K. Brechin and J. J. Alonso, Angew. Chem., Int. Ed., 2011, 50, 6606–6609 CrossRef CAS PubMed;
(f) J.-M. Jia, S.-J. Liu, Y. Cui, S.-D. Han, T.-L. Hu and X.-H. Bu, Cryst. Growth Des., 2013, 13, 4631–4634 CrossRef CAS.
- F.-S. Guo, Y.-C. Chen, J.-L. Liu, J.-D. Leng, Z.-S. Meng, P. Vrábel, M. Orendáč and M.-L. Tong, Chem. Commun., 2012, 48, 12219–12221 RSC.
Footnote |
† Electronic supplementary information (ESI) available: Additional property characterization, and selected bond lengths and angles. See DOI: 10.1039/c8qm00439k |
|
This journal is © the Partner Organisations 2018 |
Click here to see how this site uses Cookies. View our privacy policy here.