DOI:
10.1039/C8QM00436F
(Research Article)
Mater. Chem. Front., 2018,
2, 2320-2326
Ni-modified Ti3C2 MXene with enhanced microwave absorbing ability
Received
29th August 2018
, Accepted 10th October 2018
First published on 10th October 2018
Abstract
In this work, we have demonstrated the enhancement of the microwave absorbing capability of Ni-modified MXene within a frequency range of 2–18 GHz. The Ni-modification makes MXene able to attenuate microwave energy through magnetic loss as well as a strong dielectric loss. The effective absorbing bandwidth is greatly broadened, especially at the lower frequency of 2–8 GHz. The synergistic effect of dielectric loss and magnetic loss and the structure effect are believed to be the main reasons behind the broadening of the effective microwave absorbing bandwidth.
1. Introduction
Materials with laminated, porous and 3D-network structures are proved to be broad band and tunable high-performance microwave absorbers, and the individual microstructures and abundant interfaces are thought to be the main reasons for the outstanding microwave absorbing ability.1–3 Among them, two dimensional (2D) materials are the most widely studied, owing to their low density and superior mechanical flexibility. Since the discovery and exploration of graphene in the past decade,4–8 interest in two-dimensional materials has greatly increased due to their unique microstructures and variety of applications, especially for microwave absorbing materials.
In 2011, a new 2D material labeled as MXene9 was synthesized by selectively etching Al atoms from Ti3AlC2, which is a representative of the famous MAX phase (a family of ternary transition metal carbides and/or nitrides with a general formula of Mn+1AXn, where n = 1, 2 or 3, M is an early transition metal, A is an A-group element, and X is carbon and/or nitrogen)10 The exact formula of MXene is Ti3C2Tx, where Ti3C2 is the inner skeleton, and Tx is the active surface terminations (–OH and/or –F). And because of its unique microstructure, MXene is highly hydrophilic with a good electrical conductivity. Besides, MXene possesses a tunable interlayer space, which can be adjusted by spontaneous ion and/or molecule intercalation.11,12
MXenes9,13 have already shown great potential in the fields of energy storage devices,14–18 supercapacitors,19 catalysts,20,21 smart sensors,22 sewage treatment,20,23 electromagnetic interference shielding materials,24,25 microwave absorbers26–30etc. Particularly for microwave absorbing materials, there exist several superior properties for microwave absorption. Firstly, the fierce chemical reaction of Ti3AlC2 with hydrofluoric acid in the synthesis process derives abundant intrinsic defects, which make the derived MXene capable as a microwave absorbing material for a strong dielectric relaxation and polarization at these defect sites. Secondly, the active –OH and/or –F terminations make it possible to graft MXene with electronegative atoms/groups/molecules, which capacitate a tunable microwave absorbing performance. Finally, the laminated microstructure provides abundant interfaces, which could offer enhanced multi-internal-reflections and enhanced microwave absorbing ability. In our previous work,31 we have already exhibited the capable microwave absorbing ability of Ti3C2 MXene within the frequency range of the C-band (4–8 GHz), X-band (8–12 GHz) and Ku-band (12–18 GHz), respectively, and the underlying mechanisms of the dielectric responses are intensively discussed.
Herein, we have presented a surface Ni-modification approach to enhance the microwave absorbing performance of Ti3C2 MXene. Ni-modification is conducted through the spontaneous chemical reaction between alkalized Ti3C2 MXene and Ni-ions. After the surface Ni-modification, MXene exhibits considerable magnetic responses and enhanced microwave attenuation performance with a broadened effective microwave absorption bandwidth. The derived magnetic responses from Ni-modification work together with the intrinsic dielectric loss and multi-internal-reflections to be responsible for this enhanced microwave absorbing performance.
2. Experimental
2.1 Sample preparation
Ti3C2 MXene used in this work is synthesized by immersing commercially available Ti3AlC2 powders (purity >95%, d50 = 30 μm, provided by Forsman Scientific (Beijing) Co. Ltd) in 49 wt% aqueous HF in an ultrasonic homogenizer for 4 h as reported in our previous work.32 The as-derived Ti3C2 MXene nanopowders are separated by centrifuge and washed with distilled water until pH ≈ 7.
1 M KOH solution is prepared by dissolving 5.6 g KOH in 50 ml deionized water, transferring it to a 100 ml volumetric flask, and adding water to the scale bar. The Ni2+ containing solution is made by dissolving 23.8 g NiCl2·6H2O in deionized water in a 100 ml volumetric flask (1 M Ni2+ containing solution).
To derive Ni-modified MXene, an alkalized MXene (alk-MXene) is firstly prepared by immersing Ti3C2 MXene in KOH solution for 24 h with stirring. The alk-MXene is then separated by centrifuge and added into the Ni2+ containing solution for 24 h. After this the as-derived Ni-modified MXene is separated by centrifuge and dried in a vacuum oven for 12 h. To evaluate the microwave absorbing ability, donut-shaped samples with 7.0 mm outer diameter and 3.0 mm inner diameter are prepared by mixing the as-prepared Ni-modified MXene powders with molten paraffin with a weight ratio of about 1
:
1.
2.2 Characterization
The phase composition and crystal structure analysis is conducted on an X-ray diffractometer (D/max-2400, Rigaku) using Cu Kα radiation (λ = 0.1546 nm) and a step scan of 0.02° with 1 s per step, operating at 40 kV and 20 mA, respectively, with data analysis performed with Jade software. The microstructure is characterized by Scanning Electron Microscopy (SEM) on a Quanta 400, FEI, America, and TEM operated at 200 kV on a JEM 2100, Japan; Energy-Dispersive Spectroscopy (EDS) is performed using this instrument (Oxford EDS, with INCA software). All the measurements are carried out at room temperature.
The electromagnetic (EM) parameters (relative permittivity εr and permeability μr) of each sample are determined using a coaxial line method with a vector network analyzer (Agilent AV3618). For accuracy of measurement, the system is carefully calibrated with a Short-Open-Load-Through (SOLT) approach. Based on theory of transmission lines,33,34 the reflection loss (RL) of a metal-backed absorber with εr and μr could be expressed as:
| 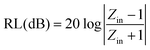 | (1) |
and
| 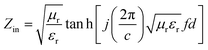 | (2) |
where
Zin refers to input impedance,
j is the imaginary unit (
i.e. equals to

),
c is the velocity of electromagnetic waves in free space,
f is the microwave frequency, and
d is the thickness of the samples.
3. Results and discussion
The typical diffraction peak (002) of Ti3C2 MXene appears at around 2θ = 8.98°, indicating that the interlayer distance of Ti3C2 is about 0.98 nm according to Bragg equation: 2d
sin
θ = nλ.35 After Ni modification, this peak shifts to a lower angle around 2θ = 6.72°, as shown in Fig. 1, indicating that the c-lattice parameter expanded to about 1.32 nm. This distinct downshift is usually caused by ions and/or small molecules intercalating into the interlayer of Ti3C2 MXene.9,11,12,36 Here the Ni ion intercalation into the MXene layers and the Ni modification with the active surface terminations are effectuated simultaneously. However, different from the single process of Ni-intercalation, the process of Ni-modification includes both the intercalations and modifications, and also the modification is irreversible, due to a stable chemical bond derived from the active alkalized surface terminations and nickel ions.
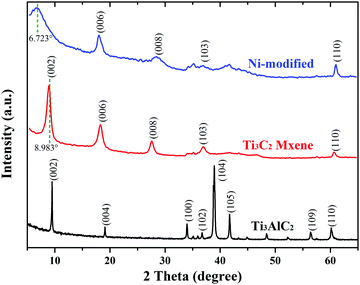 |
| Fig. 1 XRD pattern of standard Ti3AlC2, Ti3C2 MXene and the as-produced Ni-modified Ti3C2 MXene. | |
Fig. 2a shows the typical morphology of the cross-section of a Ti3C2 MXene particle with an accordion-like structure. Energy dispersive X-ray spectroscopy (Fig. 2b) reveals that the laminated materials are composed of Ti, C, O, F and a little Al. The excess O and F signal could be ascribed to the surface terminations (–F and/or –OH), and possibly the intercalated water and hydrofluoric acid between Ti3C2 layers.9,32 The remaining Al signal is believed to be originated from the reaction product, AlF3, which has not been fully washed away from the particles. After being treated in Ni2+ containing solution, the open accordion-like structure of Ti3C2 MXene seems to be filled with Ni ions as can be seen from Fig. 2c, and the EDS spectrum (Fig. 2d) indicates that the chemical compositions of Ni-modified MXene are Ti, C, O and Ni. The remaining F and H atoms in pristine Ti3C2 MXene are alkalized into KOH solution after the alkalization process. For this reason, they are not detected in the Ni-modified MXene. Since Ni-modification is a kind of surface chemical modification, the amount of Ni atoms is small compared to the base elements of Ti3C2 MXene.
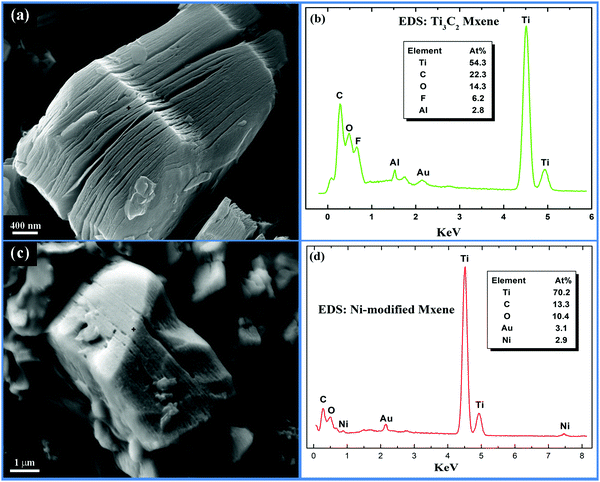 |
| Fig. 2 (a) SEM image and (b) EDS spectrum of pristine Ti3C2 MXene; (c) SEM image and (d) EDS spectrum of the as-derived Ni-modified MXene. | |
Based on the above results, the evolution process from Ti3C2 MXene to Ni-modified MXene can be described as follows (as showing in Fig. 3). When Ti3C2 MXene is immersed in KOH solution, the faintly acidic surface terminations (–OH and/or –F) of Ti3C2 MXene could react with KOH and get alkalized. After transferring the alkalized MXene into Ni-ion containing solution, Ni-ions could spontaneously sedimentate on the surface of MXene due to a simple chemical rule that Ni ions are only stable under acidic conditions. The chemical process can be described as the following equation:
|  | (3) |
Fig. 4 exhibits a comparison of the frequency dependencies of complex permittivity and permeability in the range of 2–18 GHz of pristine Ti
3C
2 MXene and the Ni-modified MXene. As can be seen from the picture, both the real and imaginary parts of the permittivity of pristine MXene and the Ni-modified MXene are fluctuated in the same trend within an examined frequency range. However, the Ni-modification makes a reduction of permittivity, both the real and imaginary part, for the reason that Ni-modification could improve the conductivity of MXene. This reduction could effectively help optimize the impedance matching with microwaves, which is beneficial for microwave absorption. What is more, the permeability of Ni-modified MXene shows a relatively considerable magnetic response compared to that of pristine MXene. It is reasonable to speculate that this obvious enhancement of complex permeability of Ni-modified MXene compared with that of pure MXene (
i.e. μr ≈ 1) is originated from surface modification of Ni-ions. Furthermore, this synergistic effect of decreased permittivity and enhanced permeability brings them closer, which is quite essential to improve impedance the matching and microwave absorbing ability.
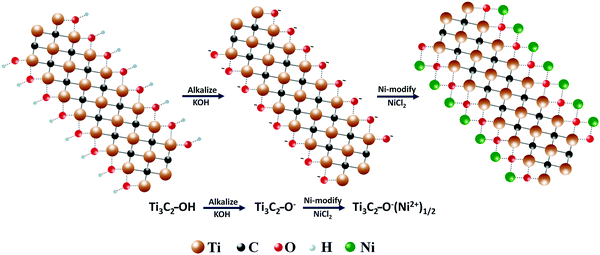 |
| Fig. 3 A schematic illustration of the process of alkalization and Ni-modification of Ti3C2 MXene. (This figure is prepared by author Wanlin Feng using the photoshop software). | |
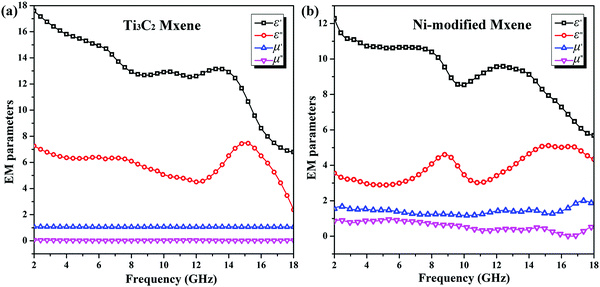 |
| Fig. 4 Electromagnetic parameters of (a) pristine Ti3C2 MXene and (b) as-produced Ni-modified MXene. | |
As demonstrated in Fig. 5a, Ti3C2 MXene, a typical dielectric loss microwave absorber,31 shows good microwave absorbing performance at six typical values of thickness within a frequency range of 12.3–18 GHz, 9.2–12.1 GHz, 7.2–9.5 GHz, 4.8–6.2 GHz, 3.6–4.8 GHz, and 2.9–3.8 GHz, where reflection loss (RL) is below −10 dB, which suggests that more than 90% of incident microwave energy has been absorbed. However, Fig. 5b shows the enhancement of microwave absorbing performance of Ni-modified MXene at the same thicknesses. To have a better visual view of the enhancement of microwave absorbing performance, a histogram is prepared in Fig. 5c. The black column represents the effective absorbing bandwidth (where RL <−10 dB) of pristine Ti3C2 MXene at five typical thicknesses, while the red column is that of Ni-modified MXene (Fig. 5c). It can be observed from the histogram that Ni-modification leads to a great improvement of microwave absorption performance. The effective absorbing bandwidth is greatly enhanced from 5.7 GHz, 2.5 GHz, 1.5 GHz, 1.07 GHz and 0.84 GHz to 6.3 GHz, 5.5 GHz, 2.5 GHz, 1.75 GHz and 1.6 GHz, respectively.
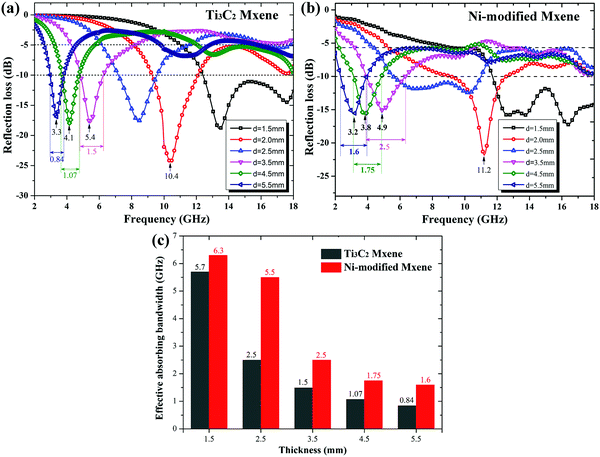 |
| Fig. 5 Reflection loss of (a) Ti3C2 MXene and (b) Ni-modified MXene at six typical values of thickness, and (c) a comparison of the effective absorbing bandwidth (RL < −10 dB) between Ti3C2 MXene and Ni-modified MXene. | |
To figure out the underlying mechanism of the enhanced microwave absorbing performance, the dielectric loss tangent (tan
δE = ε′′/ε′) and magnetic loss tangent (tan
δM = μ′′/μ′) are calculated based on the permittivity and permeability shown in Fig. 4. As can be clearly seen from Fig. 6a, Ti3C2 MXene is a typical dielectric loss microwave absorber with a strong dielectric loss ability (dielectric loss tangent keeps over 0.4 during the examined frequency range), especially in a high frequency range of 13–18 GHz, and with no magnetic loss ability (magnetic loss tangent keeps around 0). However, due to the magnetic responses ascribed to Ni-modified MXene, the magnetic loss tangent keeps over 0.5 during the frequency range of 2–8 GHz, suggesting a strong microwave energy attenuation capability of magnetic loss (Fig. 6b). Although the magnetic loss tangent decreased to 0.25 within a frequency range of 8–15 GHz, it also means a considerable magnetic loss capability compared to that of pristine Ti3C2 MXene. What is more, the Ni-modified MXene still keeps a strong dielectric loss capability over the whole examined frequency range (2–18 GHz). As a result, we can come to the conclusion that the synergistic effect of dielectric loss and magnetic loss is supposed to be responsible for the broadening of the effective microwave absorbing bandwidth. In addition, the laminar structure provides abundant interfaces, which could offer enhanced multi-internal-reflections and could help for the enhanced microwave absorbing ability.
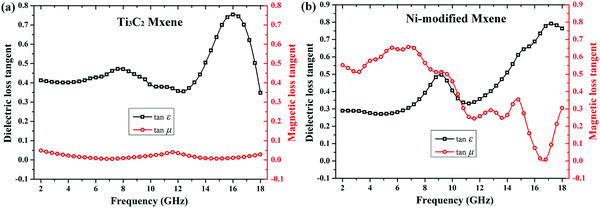 |
| Fig. 6 The dielectric loss tangent and magnetic loss tangent of Ti3C2 MXene and Ni-modified MXene. | |
4. Conclusions
Samples of Ni-modified MXene are successfully prepared by simple alkalization and surface Ni-ion modification of Ti3C2 MXene. XRD, FESEM and EDS characterization confirms the modification of Ni elements. The Ni-modification makes Ti3C2 MXene able to attenuate microwave energy through magnetic loss as well as a strong dielectric loss. The effective absorbing bandwidth is greatly enhanced from 5.7 GHz, 2.5 GHz, 1.5 GHz, 1.07 GHz and 0.84 GHz to 6.3 GHz, 5.5 GHz, 2.5 GHz, 1.75 GHz and 1.6 GHz, respectively. Furthermore, the synergistic effect of decreased permittivity and enhanced permeability caused by Ni-modification brings them closer, which is quite essential to improve impedance matching. Finally, the collaboration of dielectric loss and magnetic loss working together with multi-reflections ascribed to laminar structures, is supposed to be responsible for broadening the microwave absorbing bandwidth.
Conflicts of interest
The authors declare no competing interests.
Acknowledgements
Haibin Zhang is grateful to the foundation by the Recruitment Program of Global Youth Experts and the Youth Hundred Talents Project of Sichuan Province. This work was supported by the National Natural Science Foundation of China (Grant No. 91326102), China National Science and Technology Major Special Project ‘Research on Accident Tolerant Fuels Key Technology’ (Grant No. 2015ZX06004-001), and China Postdoctoral Science Foundation (Grant No. 2015M580798 and 2017M612996). Lianwen Deng is grateful to the foundation by the National Key Research and Development Program of China (Grant No. 2017YFA0204600) and the Provincial Science and Technology Program (Grant No. 2015JC3041) of Hunan.
References
- Y. H. Chen, Z. H. Huang, M. M. Lu, W. Q. Cao, J. Yuan and D. Q. Zhang,
et al., 3D Fe3O4 nanocrystals decorating carbon nanotubes to tune electromagnetic properties and enhance microwave absorption capacity, J. Mater. Chem. A, 2015, 3(24), 12621–12625 RSC.
- Y. Zhang, Y. Huang, T. Zhang, H. Chang, P. Xiao and H. Chen,
et al., Broad Band and tunable high-performance microwave absorption of an ultralight and highly compressible graphene foam, Adv. Mater., 2015, 27(12), 2049–2053 CrossRef CAS PubMed.
- C. Zongping, X. Chuan, M. Chaoqun, R. Wencai and C. Hui-ming, Lightweight and Flexible Graphene Foam Composites for High-Performance Electromagnetic Interference Shielding, Adv. Mater., 2013, 25, 1296–1300 CrossRef PubMed.
- A. K. Geim and K. S. Novoselov, The rise of graphene, Nat. Mater., 2007, 6(3), 183–191 CrossRef CAS PubMed.
- K. S. Novoselov, A. K. Geim, S. V. Morozov, D. Jiang, Y. Zhang and S. V. Dubonos,
et al., DISCOVER of graphene: electric field effect in atomically thin carbon films, Science, 2004, 306(5696), 666–669 CrossRef CAS PubMed.
- S. Stankovich, D. A. Dikin, R. D. Piner, K. A. Kohlhaas, A. Kleinhammes and Y. Jia,
et al., Synthesis of graphene-based nanosheets via chemical reduction of exfoliated graphite oxide, Carbon, 2007, 45(7), 1558–1565 CrossRef CAS.
- C. Wang, X. Han, P. Xu, X. Zhang, Y. Du and S. Hu,
et al., The electromagnetic property of chemically reduced graphene oxide and its application as microwave absorbing material, Appl. Phys. Lett., 2011, 98(7), 217 Search PubMed.
- X. Bai, Y. Zhai and Y. Zhang, Green Approach To Prepare Graphene-Based Composites with High Microwave Absorption Capacity, J. Phys. Chem. C, 2011, 115(23), 11673–11677 CrossRef CAS.
- M. Naguib, M. Kurtoglu, V. Presser, J. Lu, J. Niu and M. Heon,
et al., Two-dimensional nanocrystals produced by exfoliation of Ti3AlC2, Adv. Mater., 2011, 23(37), 4248–4253 CrossRef CAS PubMed.
- M. W. Barsoum, The MAX Phases: A New Class of Solids, Prog. Solid State Chem., 2000, 28, 201–281 CrossRef CAS.
- O. Mashtalir, M. R. Lukatskaya, M. Q. Zhao, M. W. Barsoum and Y. Gogotsi, Amine-Assisted Delamination of Nb2C MXene for Li-Ion Energy Storage Devices, Adv. Mater., 2015, 27(23), 3501–3506 CrossRef CAS PubMed.
- M. R. Lukatskaya, O. Mashtalir, C. E. Ren, Y. Dall'Agnese, P. Rozier and P. L. Taberna,
et al., Cation Intercalation and High Volumetric Capacitance of Two-Dimensional Titanium Carbide, Science, 2013, 341, 1502–1505 CrossRef CAS PubMed.
- B. Anasori, Y. Xie, M. Beidaghi, J. Lu, B. C. Hosler and L. Hultman,
et al., Two-Dimensional, Ordered, Double Transition Metals Carbides (MXenes), ACS Nano, 2015, 9(10), 9507–9516 CrossRef CAS PubMed.
- M. Ghidiu, M. R. Lukatskaya, M. Q. Zhao, Y. Gogotsi and M. W. Barsoum, Conductive two-dimensional titanium carbide ‘clay’ with high volumetric capacitance, Nature, 2014, 516(7529), 78–81 CAS.
- Q. Tang, Z. Zhou and P. Shen, Are MXenes promising anode materials for Li ion batteries: Computational studies on electronic properties and Li storage capability of Ti3C2 and Ti3C2X2 (X = F, OH) monolayer, J. Am. Chem. Soc., 2012, 134(40), 16909–16916 CrossRef CAS PubMed.
- M. Naguib, J. Halim, J. Lu, K. M. Cook, L. Hultman and Y. Gogotsi,
et al., New two-dimensional niobium and vanadium carbides as promising materials for Li-ion batteries, J. Am. Chem. Soc., 2013, 135(43), 15966–15969 CrossRef CAS PubMed.
- D. Er, J. Li, M. Naguib, Y. Gogotsi and V. B. Shenoy, Ti3C2 MXene as a high capacity electrode material for metal (Li, Na, K, Ca) ion batteries, ACS Appl. Mater. Interfaces, 2014, 6(14), 11173–11179 CrossRef CAS PubMed.
- J. Hu, B. Xu, S. A. Yang, S. Guan, C. Ouyang and Y. Yao, 2D Electrides as Promising Anode Materials for Na-Ion Batteries from First-Principles Study, ACS Appl. Mater. Interfaces, 2015, 7(43), 24016–24022 CrossRef CAS PubMed.
- R. B. Rakhi, B. Ahmed, M. N. Hedhili, D. H. Anjum and H. N. Alshareef, Effect of Postetch Annealing Gas Composition on the Structural and Electrochemical Properties of Ti2CTx MXene Electrodes for Supercapacitor Applications, Chem. Mater., 2015, 27, 5314–5323 CrossRef CAS.
- Y. Ying, Y. Liu, X. Wang, Y. Mao, W. Cao and P. Hu,
et al., Two-dimensional titanium carbide for efficiently reductive removal of highly toxic chromium(VI) from water, ACS Appl. Mater. Interfaces, 2015, 7(3), 1795–1803 CrossRef CAS PubMed.
- Y. Liu, H. Du, X. Zhang, Y. Yang, M. Gao and H. Pan, Superior catalytic activity derived from a two-dimensional Ti3C2 precursor towards the hydrogen storage reaction of magnesium hydride, Chem. Commun., 2015, 52(4), 705–708 RSC.
- J. Chen, K. Chen, D. Tong, Y. Huang, J. Zhang and J. Xue,
et al., CO2 and temperature dual responsive “Smart” MXene phases, Chem. Commun., 2015, 51(2), 314–317 RSC.
- Q. Peng, J. Guo, Q. Zhang, J. Xiang, B. Liu and A. Zhou,
et al., Unique lead adsorption behavior of activated hydroxyl group in two-dimensional titanium carbide, J. Am. Chem. Soc., 2014, 136(11), 4113–4116 CrossRef CAS PubMed.
- F. Shahzad, M. Alhabeb, C. B. Hatter, B. Anasori, H. S. Man and C. M. Koo,
et al., Electromagnetic interference shielding with 2D transition metal carbides (MXenes), Science, 2016, 353(6304), 1137–1140 CrossRef CAS PubMed.
- X. Y. Meikang Han, L. Xinliang, A. Babak, Z. Litong, C. Laifei and G. Yury, Laminated and Two-Dimensional Carbon-Supported Microwave Absorbers Derived from MXenes, ACS Appl. Mater. Interfaces, 2017, 9, 20038–20045 CrossRef PubMed.
- M. Han, X. Yin, H. Wu, Z. Hou, C. Song and X. Li,
et al., Ti3C2 MXenes with Modified Surface for High-Performance Electromagnetic Absorption and Shielding in the X-Band, ACS Appl. Mater. Interfaces, 2016, 8(32), 21011–21019 CrossRef CAS PubMed.
- X. Li, X. Yin, M. Han, C. Song, X. Sun and H. Xu,
et al., A controllable heterogeneous structure and electromagnetic wave absorption properties of Ti2CTx MXene, J. Mater. Chem. C, 2017, 5(30), 7621–7628 RSC.
- Y. Jiang, X. Xie, Y. Chen, Y. Liu, R. Yang and G. Sui, Hierarchically structured cellulose aerogels with interconnected MXene networks and their enhanced microwave absorption properties, J. Mater. Chem. C, 2018, 6(32), 8679–8687 RSC.
- X. Li, X. Yin, M. Han, C. Song, H. Xu and Z. Hou,
et al., Ti3C2 MXenes modified with in situ grown carbon nanotubes for enhanced electromagnetic wave absorption properties, J. Mater. Chem. C, 2017, 5(16), 4068–4074 RSC.
- H. Yang, J. Dai, X. Liu, Y. Lin, J. Wang and L. Wang,
et al., Layered PVB/Ba3Co2Fe24O41/Ti3C2 MXene Composite: Enhanced Electromagnetic Wave Absorption Properties with High Impedance Match in a Wide Frequency Range, Mater. Chem. Phys., 2017, 200, 179–186 CrossRef CAS.
- W. Feng, H. Luo, Y. Wang, S. Zeng, L. Deng and X. Zhou,
et al., Ti3C2 MXene: a promising microwave absorbing material, RSC Adv., 2018, 8(5), 2398–2403 RSC.
- W. Feng, H. Luo, Y. Wang, S. Zeng, Y. Tan and H. Zhang,
et al., Ultrasonic assisted etching and delaminating of Ti3C2 MXene, Ceram. Int., 2018, 44(6), 7084–7087 CrossRef CAS.
- M. S. Cao, W. L. Song, Z. L. Hou, B. Wen and J. Yuan, The effects of temperature and frequency on the dielectric properties, electromagnetic interference shielding and microwave-absorption of short carbon fiber/silica composites, Carbon, 2010, 48(3), 788–796 CrossRef CAS.
- R. C. Che, L. M. Peng, X. F. Duan, Q. Chen and X. L. Liang, Microwave Absorption Enhancement and Complex Permittivity and Permeability of Fe Encapsulated within Carbon Nanotubes, Adv. Mater., 2004, 16(5), 401–405 CrossRef CAS.
- C. G. Pope, X-Ray Diffraction and the Bragg Equation, J. Chem. Educ., 1997, 74(1), 129–131 CrossRef CAS.
- P. Lian, Y. Dong, Z.-S. Wu, S. Zheng, X. Wang and W. Sen,
et al., Alkalized Ti3C2 MXene nanoribbons with expanded interlayer spacing for high-capacity sodium and potassium ion batteries, Nano Energy, 2017, 40, 1–8 CrossRef CAS.
Footnote |
† Wanlin Feng and Heng Luo contributed equally to this work. |
|
This journal is © the Partner Organisations 2018 |
Click here to see how this site uses Cookies. View our privacy policy here.