DOI:
10.1039/D4TC01750A
(Paper)
J. Mater. Chem. C, 2024, Advance Article
Synthesis, crystal structure, and physical properties of the Eu(II)-based selenide semiconductor: EuHfSe3†
Received
28th April 2024
, Accepted 7th July 2024
First published on 8th July 2024
Abstract
Black needle-shaped crystals of a novel Eu(II)-containing chalcogenide semiconductor, EuHfSe3, have been synthesized at high temperatures using sealed-tube solid-state reaction techniques. Single crystal X-ray diffraction data (XRD) was used to characterize its structure as forming in the orthorhombic space group Pnma (a = 8.887(1) Å, b = 3.9300(4) Å, c = 14.3827(14) Å, and Z = 4) with the NH4CdCl3 structure type. The extended structure is comprised of [HfSe3]2− chains which are charge balanced by Eu(II) cations. 151Eu Mössbauer spectroscopic measurements were consistent with the coordination of only Eu(II) cations in bicapped trigonal prisms of EuSe8. The latter polyhedra are condensed via face-sharing into corrugated [EuSe2Se2/2Se4/4] layers, yielding a magnetic substructure with Eu–Eu distances of ∼3.93 Å and a longer ∼4.91 Å. This structural feature of the NH4CdCl3-type structure has been probed for its impact on the magnetic and thermodynamic properties. Magnetic susceptibility and heat capacity measurements uncover two magnetic transitions at TN1 ≈ 8 K, with glassy character, and at TN1 ≈ 4 K implying a complex canted antiferromagnetic or ferrimagnetic ground state.
1. Introduction
Metal-chalcogenide semiconductors with the AMX3 stoichiometry and group-IV M-site cations (i.e., Ti, Zr and Hf) have attracted a recent surge of interest because of their promising optoelectronic properties.1–4 These include optical absorption coefficients of greater than 105 cm−1, relatively small effective masses of 0.3 to
, as well as small visible-light band gaps in the range of ∼1.5 to 2.5 eV. Coupled with their promising kinetic stability and intense luminescence, perovskite chalcogenides appear to satisfy many of the key prerequisites for development as high efficiency photovoltaics. Many new, hypothetical compositions are predicted to exhibit relatively limited thermodynamic stability and have been difficult to prepare in high purity owing to the refractory nature of the reactants and the high reaction temperatures that are required. As a result, relatively few have been reported. For example, SrHfCh3 (Ch = S or Se) and BaHfS3 in distorted perovskite-type structures have been recently investigated for their semiconducting properties.5–7 While much research has focused on the optoelectronic properties of perovskite-type chalcogenide structures, their favorable optoelectronic properties have recently been shown to extend to non-perovskite structure types.8,9
Eu(II)-based systems have been much less explored within the semiconducting metal chalcogenides, especially when considering the well-known similarity of its structural chemistry and ionic size with the Sr(II) cation.10,11 These have included reports of the synthesis and structure of EuMS3 (M = Zr or Hf) with the GdFeO3 distorted-perovskite structure type7,12 and EuZrSe3 with the NH4CdCl3 structure type.13 In addition to their potentially useful optical properties for solar energy conversion, the Eu(II)-containing chalcogenides have also received longstanding theoretical and experimental interest for their magnetic and magneto-optical properties, such as for their especially large Faraday and Kerr effects.14,15 For example, the known Eu(II) chalcogenides, e.g., EuS, EuSe and EuTe, have been investigated for their magneto-optical properties and complex, low-temperature, phase transitions (antiferromagnetic and ferromagnetic).16–18 More broadly, and in recent years, Eu(II)-containing semiconductors have drawn significant interest as potential topological magnetic materials, such as notably for EuCd2As2,19,20 Eu5In2Sb6,21 and EuCuAs.22 In these cases, the underlying entanglement of electronic and magnetic degrees-of-freedom can yield remarkable physical phenomena such as colossal magnetoresistance and a large topological Hall effect. It is clear that Eu(II)-based ternary semiconductors can exhibit a wide range of useful and remarkable physical properties arising from the large, localized spin moments (S = 7/2) and their dynamic impact on the electronic structure and optical absorption.
Recent synthetic efforts to prepare new Eu(II)-containing semiconducting materials have resulted in the new compound EuHfSe3 obtained as single crystals and in high purity using solid-state reaction conditions. It represents the missing member of the EuIIMCh3 (M = Zr or Hf; Ch = S or Se) family with the 1
:
1
:
3 stoichiometry. Its crystal structure has been characterized by single crystal X-ray diffraction and contains
chains that consist of distorted HfSe6 octahedra that condense via their edges along the [010] direction. These chains are separated by corrugated layers of EuSe8 polyhedra. The nature of the Eu(II) cations and their magnetic interactions has been probed by Mössbauer spectroscopy and temperature- and applied magnetic field-dependent magnetic susceptibility and heat capacity measurements.
2. Experimental
2.1. Materials used and synthetic procedures
The syntheses of single crystals and polycrystalline phase were carried out using the following elemental starting materials of Eu ingot (99.9% purity, Alfa Aesar), Hf powder (99.9% purity, Alfa Aesar), Sn chunk (99.99% purity, Alfa Aesar), and Se powder (99.999% purity, Alfa Aesar). Eu ingot and Hf powder are air sensitive, so all the chemicals were handled inside an Ar-filled dry glove box.
2.1.1. Synthesis of EuHfSe3 single crystals. Our attempt to synthesize the Eu analog of our recently reported compound, Ba9Hf3Sn2Se199 produced black needle-shaped crystals of EuHfSe3. The elemental starting materials of Eu (56.3 mg, 0.370 mmol), Hf (22.1 mg, 0.124 mmol), Sn (9.8 mg, 0.083 mmol), and Se (61.8 mg, 0.783 mmol) were loaded into a 6 mm outer diameter (OD) fused silica tube inside the Ar-filled glove box aiming at a composition of Eu9Hf3Sn2Se19. The tube was then evacuated for ten minutes under ∼10−4 torr pressure and sealed using a flame torch. The sealed reaction vessel was subjected to heat treatment inside a programmable muffle furnace and heated to 773 K in 10 h where it was annealed for 12 h before ramping to 1173 K in 10 h. The tube was then annealed for 72 h at 1173 K before cooling to 573 K using a cooling rate of 20 K h−1. Finally, the furnace was shut down and allowed to cool to room temperature. The product was analyzed in an ambient atmosphere under the optical microscope revealing the presence of black needle-shape crystals along with powdered product. The needle-shaped crystals were analyzed with the help of a JEOL SEM 6010LA energy-dispersive X-ray (EDX) spectroscope using a 20 kV accelerating voltage. The EDX analysis of the needle-shaped crystals showed the presence of Eu, Hf, and Se atoms in an approximate atomic ratio of ≈1
:
1
:
3 (Fig. S1, ESI†). The EuHfSe3 single crystals were further reproduced using the same heating profile from a stoichiometric amount of elemental starting materials with a loaded amount of 150 mg. The compositions of EuHfSe3 crystals were established using EDX analyses and unit cell determination using single crystal X-ray diffraction (SCXRD).
2.1.2. Synthesis of EuHfSe3 polycrystalline phase. A two-step high-temperature solid-state synthesis method was used to synthesize pure EuHfSe3 polycrystalline phase at 1173 K. At first, a stoichiometric amount of Eu (133.9 mg, 0.881 mmol), Hf (157.3 mg, 0.881 mmol), and Se (208.8 mg, 2.644 mmol) was loaded into a 12 mm outer-diameter (OD) tube inside an Ar-filled glove box. The tube was then evacuated to 10−4 torr and sealed using a flame torch. The sealed ampoule was then heated to 823 K in 8 h inside a muffle furnace. The temperature was held for 12 h at 823 K before ramping the temperature to 1173 K. The reaction ampoule was then annealed for 60 h before switching off the furnace and allowed to cool to room temperature. The tube was opened inside the Ar-filled glove box and the product was homogeneously ground to polycrystalline powder. The polycrystalline product was then pelletized under ∼10 MPa pressure using a hydraulic press. Next, the pelletized product was transferred into a 12 mm OD fused silica tube and flame-sealed under 10−4 torr pressure. In the second step, the sealed tube was heat-treated for 72 h inside a muffle furnace. The product was ground homogeneously inside the Ar-filled glove box and the phase purity of the EuHfSe3 polycrystalline phase was established using a powder X-ray diffraction (PXRD) study. Rietveld refinement of the PXRD data is provided in the ESI.†
2.2. Single crystal X-ray diffraction
The crystal structure of EuHfSe3 was established using a single crystal X-ray diffraction data set at room temperature (300(2) K). A Bruker D8 Venture diffractometer equipped with a Photon III mixed mode detector was used for the intensity data collection using a graphite monochromatized Mo-Kα (λ = 0.71073 Å) radiation source. A black needle-shaped EuHfSe3 crystal was mounted onto a transparent loop under Paratone-N oil and fixed onto the goniometer of the diffractometer. The crystal quality was assessed, and the unit cell was determined by a fast scan of 180 frames utilizing a frame width and exposure time of 1° and 1 s per frame, respectively. A full-intensity dataset was recorded using a working voltage of 50 kV, a current of 1.4 mA, an exposure time of 3 s per frame, a frame width of 0.5°, and a crystal-to-detector distance of 50 mm. The APEX4 software23 was used to integrate the collected data and refine the unit cell parameters. The absorption correction was employed using the multi-scan method of the SADABS program.24
The XPREP program suggested a primitive (P) orthorhombic unit cell for the EuHfSe3 crystal. Based on the extinction conditions, XPREP25 suggested two space groups: Pnma (centrosymmetric) and Pna21 (non-centrosymmetric). The |E2 − 1| (mean intensity statistics) value of 0.882 indicated the centrosymmetric nature of the space group. So, the space group Pnma was used to solve the crystal structure using the direct methods of the SHELXS program of the SHELX-14 suite of programs.26 The initial solution showed peak heights, bond distances, and coordination environments of five crystallographically-independent atomic sites that were analyzed and further assigned to the respective atoms. The full-matrix least-squares on the F2 method of the SHELXL program27 was used to further refine the atomic positions, anisotropic displacement parameters, weight corrections, and scale factors. The final model provided a composition of EuHfSe3 (see Table 2).
The ADDSYM program of the PLATON software package28 suggested no missing symmetry, implying that the correct space group was found. The atomic positions of the solved structure were standardized using the STRUCTURE TIDY program.29 The crystal structure refinement and metric details are provided in Tables 1 and 2, and the ESI.†
Table 1 Crystallographic refinement and structural data for a EuHfSe3 single crystala
Refinement parameter |
EuHfSe3 |
The λ = 0.71073 Å, T = 300(2) K. R(F) = ∑‖Fo| − |Fc ‖/∑|Fo| for Fo2 > 2σ(Fo2). Rw(Fo2) = {∑[w(Fo2 − Fc2)2]/∑wFo4}1/2. For Fo2 < 0, w = 1/[σ2(Fo2)]; where P = (Fo2 + 2Fc2)/3. |
a (Å) |
8.8865(10) |
b (Å) |
3.9300(4) |
c (Å) |
14.3827(14) |
V |
502.30(9) |
Z |
4 |
ρ (g cm−3) |
7.50 |
μ (mm−1) |
54.6 |
R(F)b |
0.019 |
Rw(Fo2)c |
0.040 |
S |
1.00 |
No. of measured reflections |
8451 |
No. of independent reflections |
683 |
No. of refined parameters |
31 |
Reflections with I > 2σ(I) |
594 |
Residual electron density (min/max) |
2.1/−1.4 |
Table 2 Refined atomic coordinates within the EuHfSe3 crystal structure
Atom |
Wyckoff position |
Site symmetry |
x |
y |
z |
Ueq |
Eu(1) |
4c |
.m. |
0.43903(5) |
0.250000 |
0.67748(3) |
0.0164(1) |
Hf(1) |
4c |
.m. |
0.16993(3) |
0.250000 |
0.44303(2) |
0.0114(1) |
Se(1) |
4c |
.m. |
0.02057(8) |
0.250000 |
0.60822(5) |
0.0096(2) |
Se(2) |
4c |
.m. |
0.15979(8) |
0.250000 |
0.01233(6) |
0.0109(2) |
Se(3) |
4c |
.m. |
0.29091(9) |
0.250000 |
0.28171(6) |
0.0120(2) |
A room-temperature powder X-ray diffraction (PXRD) data set of the polycrystalline powder of EuHfSe3 was measured using Cu-Kα radiation (λ = 1.54 Å) of a PANalytical Empyrean X-ray diffractometer. The PXRD data was collected over the 2θ range of 5° to 75° using a step size of 0.013°.
2.3. Thermodynamic and magnetic property measurements
DC magnetization and AC susceptibility measurements were performed in a quantum design physical properties measurement system (PPMS) from T = 2–400 K in applied fields up to μ0H
=
14
T. AC susceptibility was measured with a 1.5 Oe drive current at frequencies greater than 1500
Hz and with a 5 Oe drive current at frequencies lower than 1500 Hz. Heat capacity measurements were performed on pressed pellets of powder in applied fields up to μ0H
=
14
T. Apiezon N grease was used to adhere the pellet to the puck. Three data points were collected at each temperature; the value used was the average of the second and third data point. At μ0H = 0 T, data were collected up to T = 150 K; this curve was fit above T = 40 K to the Debye model with an electron contribution (eqn (1)) as a background (Cbg). The fit parameters were A = 0.1606 J (mol K2)−1, B = 317.7 J (mol K)−1, and TD = 212.8 K. |
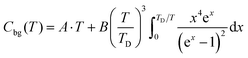 | (1) |
2.4. 151Eu Mössbauer spectroscopy
For the 151Eu Mössbauer spectroscopic investigation of the EuHfSe3 sample the 21.53 keV transition of 151Eu with an activity of 55 MBq (1% of the total activity of a 151Sm:EuF3 source) was used. The experiment was conducted in the usual transmission geometry. The measurement was performed with a commercial nitrogen-bath cryostat. The absorber was cooled to 78 K (liquid nitrogen), while the source was kept at room temperature. The sample was diluted with α-quartz and placed within a thin-walled PMMA container at a thickness corresponding to about 7 mg Eu cm−2. Fitting of the spectrum was performed with the WinNormos for Igor7 program package30 and the graphical editing with the program CorelDraw 2017.31
3. Results and discussion
3.1. Syntheses and crystal structure of EuHfSe3
Initially, single crystals of EuHfSe3 were serendipitously synthesized at 1173 K using a high-temperature solid-state synthesis method. The yield of the EuHfSe3 crystals was ∼60% based on the loaded amount of Hf. Further, the EuHfSe3 crystals were later reproduced at the nominal stoichiometry with a yield of ∼80% starting from the elements and using an identical heating profile. A black-colored polycrystalline sample of EuHfSe3 was prepared at 1173 K using a two-step solid-state synthetic procedure. The phase purity of the EuHfSe3 polycrystalline powder was studied using room temperature PXRD data, as provided in the ESI† in Fig. S2. Rietveld refinement of the PXRD data confirmed the formation of polycrystalline EuHfSe3 with a secondary phase of EuSe (11 wt%) present in the sample (Fig. S2, ESI†).
Room temperature single-crystal XRD data of EuHfSe3 showed that it crystallizes in the orthorhombic space group Pnma with the NH4CdCl3 structure type (keeping in mind that the isotype relies only in the position of the nitrogen atom, neglecting the orientation of the ammonium group).32 The unit cell contains five crystallographically unique atomic sites (one Eu, one Hf, and three Se atoms), as listed in Table 2. A unit cell view of the EuHfSe3 crystal structure is shown in Fig. 1a. The EuHfSe3 crystal structure is comprised of [HfSe3]2− chains which are condensed along the [010] direction. The [HfSe3]2− chains are charge balanced and separated from each other by isolated Eu atoms. Each of the Hf(1) atoms is connected to three Se(1), two Se(2), and one Se(3) making distorted Hf(1)Se6 octahedra units. The Eu coordination environments are shown in Fig. 1(b) and (c), respectively. Each of the Hf(1)Se6 units shares four edges with adjacent four Hf(1)Se6 units. Condensation of the Hf(1)Se6 units leads to the formation of
chains. In EuHfSe3, the Hf(1)–Se distances range from 2.5572(9) Å to 2.7214(9) Å (Table 3), which can be compared with the Hf–Se distances in the previously reported compounds such as Ba8Hf2Se11(Se2) (2.6249(4)–2.7732(4) Å),8 SrHfSe3 (2.559(2)–2.720(9) Å),5 Tl2PbHfSe4 (2.6804(5)–2.7296(5) Å),33 and NaCuHfSe3(2.608(1)–2.788(1) Å).34
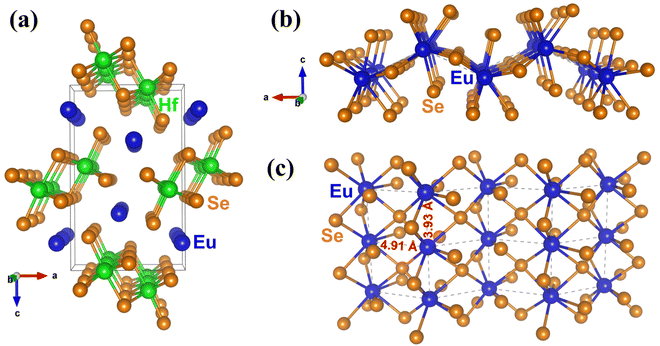 |
| Fig. 1 A unit cell view of the EuHfSe3 structure oriented approximately along the b-axis (a), and views of the [EuSe2Se2/2Se4/4] corrugated layers in the same orientation with Eu–Eu nearest-neighbor distances shown as dashed lines (b), and the same layer oriented down the c-axis (c). | |
Table 3 Selected interatomic distances in the EuHfSe3 crystal structure
Atom pair |
Distance in Å |
Atom pair |
Distance in Å |
Hf(1)–Se(3) |
2.5572(9) |
Eu(1)–Se(1) |
3.1664(9) |
Hf(1)–Se(2) |
2.6729(6) × 2 |
Eu(1)–Se(2) |
3.2053(8) × 2 |
Hf(1)–Se(1) |
2.6963(6) × 2 |
Eu(1)–Se(3) |
3.2069(8) × 2 |
Hf(1)–Se(1) |
2.7214(9) |
Eu(1)–Se(2) |
3.3617(9) |
Eu(1)–Se(3) |
3.1568(7) × 2 |
Eu(1)⋯Eu(1) |
3.9300(4) |
The magnetic substructure is comprised of Eu(II) cations coordinated within bicapped trigonal prismatic geometries, i.e., EuSe8 polyhedra in Fig. 1b. The Eu–Se distances (3.1568(7)–3.3617(9) Å) are listed in Table 3 and are consistent with similar distances reported in the isostructural EuZrSe3 (3.147(2)–3.338(2) Å).13 The bicapped trigonal prisms of EuSe8 are further bridged into chains down the b-axis via their shared faces, illustrated in Fig. 1(b) and (c), yielding relatively short Eu–Eu distances of 3.9300(4) Å labeled with dashed lines. These chains are further bridged via edge sharing, with longer Eu–Eu distances of ∼4.91 Å, into a corrugated layer that is charge balanced and separated by the Hf(IV) cations. The connectivity of the magnetic substructure is thus given by [EuSe2Se2/2Se4/4], and it forms a corrugated layer with the dimensions of a rectangular net. This structural feature of the NH4CdCl3-type structure has not previously been investigated for its impact on the magnetic and thermodynamic properties, as discussed below.
Given the EuHfSe3 composition, the compound is found to be semiconducting with a direct transition of ∼1.5 eV, Fig. S3 in the ESI.† The nominal oxidation states of the cations can be expected to be Eu(II) and Hf(IV). Bond valence sum (BVS)35 calculations were carried out using the EXPO 2013 software36 to estimate the oxidation states of the elements in the EuHfSe3 crystal structure, and the BVS values of respective atoms are given in Table 4. The BVS values of +4 for Hf(1) and −2 for the Se atoms are in good agreement with the BVS values of these respective elements. So, the oxidation states of the Eu, Hf, and Se atoms are +2, +4, and −2, respectively, giving a charge balanced formula of (Eu2+)(Hf4+)(Se2−)3.
Table 4 Bond-valence sums compared to expected oxidation states in EuHfSe3
Atom |
BVS value |
Expected oxidation state |
Eu(1) |
1.84 |
+2 |
Hf(1) |
4.05 |
+4 |
Se(1) |
2.08 |
−2 |
Se(2) |
1.93 |
−2 |
Se(3) |
1.88 |
−2 |
3.2. 151Eu Mössbauer spectroscopy
The 151Eu Mössbauer spectrum of EuHfSe3 at 78 K is plotted in Fig. 2 together with a transmission integral fit. EuHfSe3 shows a single signal at an isomer shift of δ = −12.33(1) mm s−1 indicating Eu(II) with an experimental line width of Γ = 2.25(6) mm s−1. The Eu(II) ions in EuHfSe3 occupy the Wyckoff site 4c with the non-cubic site symmetry.m. and are coordinated by eight short (3.157–3.362 Å Eu–Se) and one longer (3.850 Å Eu–Se) Se atoms arranged in the form of a significantly distorted multi-capped trigonal prism. Because of the electronic configuration, the quadrupole splitting in Eu(II) compounds can only arise from the lattice contribution to the electric field gradient.37 The comparatively small lattice contribution results in an electric quadrupole splitting of ΔEQ = 2.4(1) mm s−1 in EuHfSe3.
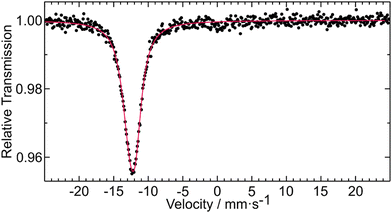 |
| Fig. 2 Experimental (data points) and simulated (red line) 151Eu Mössbauer spectrum of EuHfSe3 measured at 78 K. | |
The isomer shift of EuHfSe3 is comparable with values of −12.10(2) and −12.18(2) mm s−1 that have been observed for EuSbSe3 and EuBiSe3, respectively.38 In a prior report,39 Berkooz investigated the isomer shifts of divalent europium compounds: oxides, sulfides and selenides. The values of the selenides were in a range between −12.2 (Eu0.6Gd0.4Se) to −13.8 mm s−1 (EuHo2Se4 and EuY2Se4) relative to Eu2O3 (δ ≈ 1.05 mm s−1 of Eu2O3 relative to 151Sm:EuF340). The results were correlated with the crystal structure of the Eu(II) compounds and a monotonic increase of the isomer shifts as the mean ionic distances decrease was observed for each one of the series. The new EuHfSe3 with its isomer shift (δ ≈ −13.38 mm s−1 relative to Eu2O3) and a mean ionic distance (Eu2+–Se2−) of 3.21 Å for the eight nearest neighbors fits well into the series of the selenides studied by Berkooz.39 The average Eu–Se distance including the ninth selenium atom at 3.85 Å (8 + 1 coordination) of 3.28 Å is much larger and out of the range of the Berkooz plot. Thus, the 151Eu Mössbauer spectroscopic data is sensitive to the Eu–Se distances and the ninth neighbor can only be considered as loosely bound.
3.3. Magnetic and thermodynamic properties
To study the magnetic properties of EuHfSe3, DC magnetization and AC susceptibility were measured on a polycrystalline sample, as the single crystals were too small to attempt these measurements. Magnetization (M) as a function of applied magnetic field was measured at several temperatures, shown in Fig. 3a. At T = 2 K, a very small net moment is observed, and the curve has two distinct slopes in the low-field region, suggestive of multiple magnetic phenomena. At T = 6 K, the net moment is reduced, and only one slope is observed. DC susceptibility (χ) as a function of temperature in low applied fields (Fig. 3b and Fig. S4–S6, ESI†) exhibits two peaks that appear antiferromagnetic, at approximately TN2 ≈ 4 K and TN1 ≈ 8 K. Splitting between the zero-field-cooled (ZFC) and field-cooled (FC) data is observed below the TN1 ≈ 8 K transition. Above these transitions, the field sweep is linear and consistent with paramagnetism, Fig. 3a at T = 20 K. At higher fields, both transitions are suppressed, as shown in Fig. 3b. We note that the sample used in the magnetization measurements contains ∼11 wt% EuSe, which has transitions at ∼4.6 K and ∼2.8 K.41–43 Thus, it is possible that the transition corresponding to TN2 might arise in part from this impurity, although the fact that no peak at ∼2.8 K is observed in our data (see Fig. 3b, 4a, 5 and Fig. S4–S6, ESI†) implies that the magnetic contribution from EuSe is likely minimal. A Curie–Weiss fit was performed on the inverse susceptibility data collected at μ0H = 1 T between 51–400 K in Fig. 3c with a diamagnetic correction χ0 of −6.3 × 10−4. It yielded a Weiss temperature (θ) of 3.8(2) K, consistent with weak correlations. The extracted Curie constant is 8.47(2) K emu per mol, yielding an effective moment (μeff) of 8.23(1)μB per f.u.−1, consistent with the expected moment for Eu(II) of 7.94μB. Overall, the antiferromagnetic character of the susceptibility (Fig. 3b) with the small observed net moment (Fig. 3a) and small, positive Weiss temperature suggest a canted antiferromagnetic or ferrimagnetic ground state.
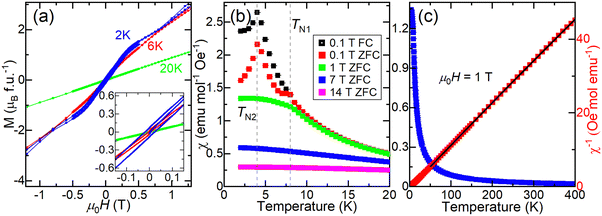 |
| Fig. 3 DC magnetization and susceptibility of EuHfSe3. (a) Magnetization (M) as a function of applied magnetic field at several temperatures. The inset shows the low-field region. (b) Magnetic susceptibility (χ) as a function of temperature at several applied fields. The dashed lines denote the transition temperatures. (c) Susceptibility and inverse susceptibility (χ−1) collected in an applied field of μ0H = 1 T. The Curie-Weiss fit is the black line. | |
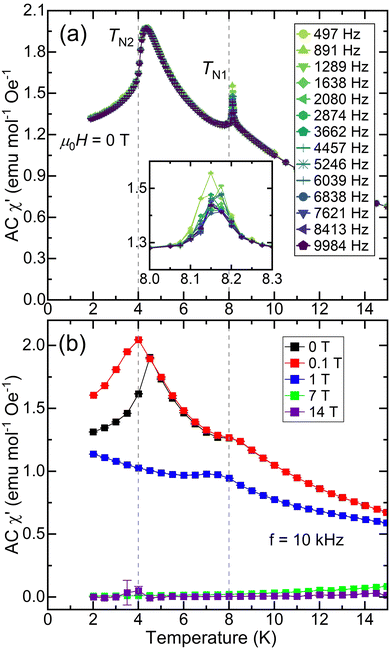 |
| Fig. 4 AC susceptibility of EuHfSe3. (a) Frequency dependence of the real part of the AC susceptibility (χ′) measured in zero applied field. The inset shows the data near the TN1 transition. (b) Real part of the AC susceptibility measured at several applied fields. The dashed lines denote the transition temperatures. | |
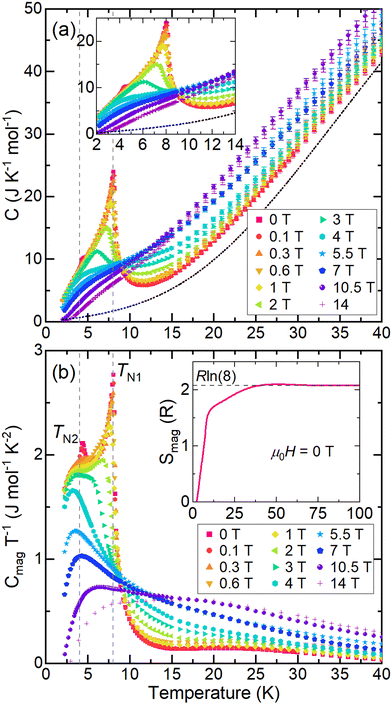 |
| Fig. 5 Heat capacity of EuHfSe3. (a) Molar heat capacity (Cp) at several applied fields; the dashed line indicates the background (Cbg) for the μ0H = 0 T curve extrapolated to T = 0 K; see methods and Fig. S11 (ESI†) for details. The inset shows the low-temperature region. (b) Heat capacity related to the low-temperature peaks (Cmag) calculated by subtracting Cbg from the molar Cp. The inset shows the entropy of the low-temperature peaks (Smag) normalized per formula unit for the μ0H = 0 T curve. The vertical dashed lines denote the transition temperatures. | |
To probe the dynamics of the magnetic response, AC susceptibility was measured as a function of frequency in zero applied field; the real part (χ′) is shown in Fig. 4a, and the imaginary part (χ′′), which displays a peak at TN1, is shown in Fig. S9 (ESI†). The real part of the AC susceptibility measured at high frequency, Fig. 4b, is suppressed at high fields, like the behavior seen in DC susceptibility in Fig. 3b. In the zero-field χ′ data in Fig. 4a, the TN1 transition shifts to higher temperature with higher frequency, consistent with spin glassiness. Additionally, temperature-dependent ZFC DC susceptibility data collected at very low field (μ0H = 0.005 T) are diamagnetic at 2 K before increasing sharply as a function of time (Fig. S7, ESI†), saturating only after approximately 40 minutes. Taken together with the observation of ZFC-FC splitting in Fig. 3b, these data suggest interesting relaxation dynamics in the magnetic ground state of EuHfSe3, which likely has spin glass character.
To examine the entropy changes associated with the two low-temperature transitions, heat capacity measurements were collected on a polycrystalline sample of EuHfSe3 in applied fields from 0–14 T. The background of the molar heat capacity collected at μ0H = 0 T was fit above T = 40 K to the Debye model with an electron contribution (eqn (1); see methods and Fig. S11, ESI†); this fit is shown in Fig. 5a as a dashed line. The molar heat capacity (Cp) shown in Fig. 5a exhibits two peaks at approximately 4.2 K and 8.0 K, consistent with the magnetic measurements. These transitions shift to lower temperature when a magnetic field is applied, confirming their magnetic character, and are nearly fully suppressed at 14 T. The heat capacity related to the magnetic transitions (Cmag = Cp − Cbg) divided by temperature is shown in Fig. 5b. As the applied field increases, the peak at TN2 is suppressed quickly, while the peak at TN1 broadens and shifts to lower temperature. We hypothesize that long-range ordering of EuHfSe3 occurs at TN1. The calculated entropy per formula unit released by these transitions for the zero-field curve by integrating Cmag/T is plotted as a function of temperature, in Fig. 5b (inset). The released entropy plateaus at approximately R
ln(8), as shown as a dashed line in Fig. 5c, above TN1 ≈ 8 K, which is consistent with the entropy expected for a Eu(II) cation (R
ln(8) or 17.29 J per K per mol).
Overall, the magnetic susceptibility and thermodynamic results are consistent with the 151Eu Mössbauer spectrum and confirm the presence of Eu(II) cations in the structure, i.e., the effective moment is consistent with the value expected for Eu(II). An enhancement in the magnetic moment is analogous to many compounds with isoelectronic rare earth cations, such as the Gd(III) cation. The increased moment is well known in rare-earth magnetochemistry to arise from gadolinium 5d electrons that induce 4f–5d exchange interactions.44 Eu(II) compounds have also been reported to show enhanced moments.45 If Eu(III) cations were present, the effective moment would be suppressed, which is not observed. The magnetic susceptibility and heat capacity data indicate magnetism beyond free Eu(II) moments. Two ordering transitions are observed at TN1 ≈ 8 K and TN2 ≈ 4 K, although the possibility of TN2 arising from EuSe is not fully ruled out. The small net moment observed below TN1 and the small positive Weiss temperature suggests that EuHfSe3 has competing interactions and a canted antiferromagnetic or ferrimagnetic ground state. In many rare-earth magnetic systems similar competing interactions have been observed as a function of temperature and magnetic field.46–50 Typically free rare-earth ion magnetism bundled with either RKKY interactions, dipole–dipole interactions, or geometric frustration are responsible, though more complicated indirect exchange interactions stemming from f-electron and spd hybridization have been proposed.51 In addition, recent work has demonstrated the occurrence of spin frustration in the rare-earth Yb(III)-containing oxyhalides in
square lattice antiferromagnets.52 This work extends these investigations to the Eu(II)-containing EuHfSe3, wherein the Eu(II) cations form a corrugated rectangular net (Fig. S12, ESI†) with nearest (∼3.93 Å) and next-nearest (∼4.91 Å) neighbors connected by Se2− anions, naturally identifying an anisotropy that could be responsible for two unique magnetic correlations as shown in Fig. 1(b), (c) and in Fig. S12 (ESI†). Nonetheless, EuHfSe3 orders at TN1 into a likely canted antiferromagnetic or ferrimagnetic structure followed by a secondary transition at TN2 that slightly increases the net moment. TN2 is suppressed with the application of a small magnetic field (∼1 T) which likely bolsters the ordering at TN1. Further study, particularly on single crystals to disentangle the contributions from EuHfSe3 and EuSe, will be necessary to better understand the low-temperature magnetic structure and ordering of EuHfSe3 as well as its relaxation dynamics and glassy character.
4. Conclusions
Single crystals and a pure polycrystalline phase of EuHfSe3 were synthesized at 1173 K using sealed-tube solid-state synthesis method. Single crystal X-ray diffraction showed that the black needle-shaped crystals of EuHfSe3 crystallize in the NH4CdCl3 structure type with orthorhombic Pnma symmetry and unit cell dimensions of a = 8.8865(10) Å, b = 3.9300(4) Å and c = 14.3827(14) Å. The edge-sharing Hf(1)Se6 units form
chains, which are separated by Eu(II) cations. Bond valence sums, a 151Eu Mössbauer spectrum, the effective magnetic moment extracted from a Curie–Weiss fit of magnetic susceptibility data, and magnetic entropy analysis indicate full consistency with the Eu(II) oxidation state. The Eu(II) cations are coordinated within EuSe8 bicapped trigonal prisms that are condensed via face-sharing into corrugated [EuSe2Se2/2Se4/4] layers, yielding a magnetic substructure with Eu–Eu distances of ∼3.93 Å and a longer ∼4.91 Å. Polycrystalline magnetic susceptibility and heat capacity measurements uncover two magnetic transitions at low temperature: the primary transition occurs at TN1 ≈ 8 K, along with a secondary transition at TN2 ≈ 4 K; some contribution arising from an EuSe impurity cannot be fully ruled out. EuHfSe3 has a complex magnetic ground state that is likely canted antiferromagnetic or ferrimagnetic, as there is a small net moment below TN1. In addition, the data suggest interesting magnetic relaxation dynamics and spin glass character at low temperature. Overall, EuHfSe3 is a novel Eu(II)-containing chalcogenide with intriguing and complex low-temperature magnetic behavior.
Author contributions
Formal analysis, investigation, visualization, and writing – all authors; project administration and supervision – RP, RWS and PAM.
Data availability
The data supporting this article have been included as part of the ESI.†
Conflicts of interest
There are no conflicts to declare.
Acknowledgements
The authors acknowledge primary support of this work from the National Science Foundation (DMR-2317605). This work was also authored in part by the National Renewable Energy Laboratory, operated by Alliance for Sustainable Energy, LLC, for the U.S. Department of Energy (DOE) under Contract No. DE-AC36-08GO28308. Single crystal X-ray characterization was performed in part by the Molecular Education, Technology and Research Innovation Center (METRIC) at NC State University, which is supported by the State of North Carolina. Funding for magnetometry and heat capacity provided by the U.S. Department of Energy, Office of Science, Basic Energy Sciences, Division of Materials Science, through the Office of Science Funding Opportunity Announcement (FOA) Number DE-FOA-0002676: Chemical and Materials Sciences to Advance Clean-Energy Technologies and Transform Manufacturing. The views expressed in the article do not necessarily represent the views of the DOE or the U.S. Government.
References
- P. Basera and S. Bhattacharya, Chalcogenide Perovskites (ABS3; A = Ba, Ca, Sr; B = Hf, Sn): An Emerging Class of Semiconductors for Optoelectronics, J. Phys. Chem. Lett., 2022, 13, 6439–6446 Search PubMed.
- R. Raj, R. Singh and M. Guin, Chalcogenide Perovskite, An Emerging Photovoltaic Material: Current Status and Future Perspectives, ChemistrySelect, 2023, 8, e202303550 Search PubMed.
- D. Tiwari, O. S. Hutter and G. Longo, Chalcogenide Perovskites for Photovoltaics: Current Status and Prospects, JPhys Energy, 2021, 3, 034010 Search PubMed.
- K. V. Sopiha, C. Comparotto, J. A. Márquez and J. S. Scragg, Chalcogenide Perovskites: Tantalizing Prospects, Challenging Materials, Adv. Opt. Mater., 2022, 10(1–27), 2101704 Search PubMed.
- N. A. Moroz, C. Bauer, L. Williams, A. Olvera, J. Casamento, A. A. Page, T. P. Bailey, A. Weiland, S. S. Stoyko, E. Kioupakis, C. Uher, J. A. Aitken and P. F. P. Poudeu, Insights on the Synthesis, Crystal and Electronic Structures, and Optical and Thermoelectric Properties of Sr1–xSbxHfSe3 Orthorhombic Perovskite, Inorg. Chem., 2018, 57, 7402–7411 Search PubMed.
- K. Hanzawa, S. Iimura, H. Hiramatsu and H. Hosono, Material Design of Green-Light-Emitting Semiconductors: Perovskite-Type Sulfide SrHfS3, J. Am. Chem. Soc., 2019, 141, 5343–5349 Search PubMed.
- R. Lelieveld and D. J. W. IJdo, Sulphides with the GdFeO3 Structure, Acta Crystallogr., Sect. B: Struct. Crystallogr. Cryst. Chem., 1980, 36, 2223–2226 Search PubMed.
- S. Jana, E. A. Gabilondo and P. A. Maggard, Two New Multinary Chalcogenides with (Se2)2− Dimers: Ba8Hf2Se11(Se2) and Ba9Hf3Se14(Se2), J. Solid State Chem., 2024, 329, 124376 Search PubMed.
- S. Jana, E. Gabilondo, S. McGuigan and P. A. Maggard, Syntheses, Crystal Structures, and Electronic Structures of Quaternary Group IV-Selenide Semiconductors, Inorg. Chem., 2024, 63, 6474–6482 Search PubMed.
- G. J. McCarthy and J. E. Greedan, Complex Oxides Containing Divalent Europium. I. Guidelines for the Prediction of New Phases. Application to the Phases of the Type EuMO3, Inorg. Chem., 1975, 14, 772–775 Search PubMed.
- J. E. Greedan, G. J. McCarthy and C. Sipe, Complex Oxides Containing Divalent Europium. II. Eu(M,M′)O3 Phases, Inorg. Chem., 1975, 14, 775–779 Search PubMed.
- S.-P. Guo, Y. Chi, J.-P. Zou and H.-G. Xue, Crystal and Electronic Structures, and Photoluminescence and Photocatalytic Properties of α-EuZrS3, New J. Chem., 2016, 40, 10219–10226 Search PubMed.
- A. Mar, and J. A. Ibers, Structure of Europium Zirconium Selenide, EuZrSe3, Acta Crystallogr., 1992, C48, 771–773 Search PubMed.
- J. C. Suits, B. E. Argyle and M. J. Freiser, Magneto-Optical Properties of Materials Containing Divalent Europium, J. Appl. Phys., 1966, 37, 1391–1397 Search PubMed.
- Y. Hasegawa, T.-a Adachi, A. Tanaka, M. Afzaal, P. O’Brien, T. Doi, Y. Hinatsu, K. Fujita, K. Tanaka and T. Kawai, Remarkable Magneto-Optical Properties of Europium Selenide Nanoparticles with Wide Energy Gaps, J. Am. Chem. Soc., 2008, 130, 5710–5715 Search PubMed.
- T. R. McGuire, B. E. Argyle, M. W. Shafer and J. S. Smart, Magnetic Properties of Some Divalent Europium Compounds, J. Appl. Phys., 1963, 34, 1345–1346 Search PubMed.
- W. Zinn, Microscopic Studies of Magnetic Properties, and Interactions Recent Results on Europium Monochalcogenides, J. Magn. Magn. Mater., 1976, 3, 23–36 Search PubMed.
- J. O. Dimmock, Optical Properties of the Europium Chalcogenides, IBM J. Res. Dev., 1970, 14, 301–308 Search PubMed.
- J.-Z. Ma, S. M. Nie, C. J. Yi, J. Jandke, T. Shang, M.
Y. Yao, M. Naamneh, L. Q. Yan, Y. Sun, A. Chikina, V. N. Strocov, M. Medarde, M. Song, Y.-M. Xiong, G. Xu, W. Wulfhekel, J. Mesot, M. Reticcioli, C. Franchini, C. Mudry, M. Müller, Y. G. Shi, T. Qian, H. Ding and M. Shi, Spin Fluctuation Induced Weyl Semimetal State in the Paramagnetic Phase of EuCd2As2, Sci. Adv., 2019, 5, eaaw4718 Search PubMed.
- I. Schellenberg, U. Pfannenschmidt, M. Eul, C. Schwickert and R. Pöttgen, A 121Sb and 151Eu Mössbauer Spectroscopic Investigation of EuCd2X2 (X = P, As, Sb) and YbCd2Sb2, Z. Anorg. Allg. Chem., 2011, 637, 1863–1870 Search PubMed.
- M. V. A. Crivillero, S. Röβler, S. Granovsky, M. Doerr, M. S. Cook, P. F. S. Rosa, J. Müller and S. Wirth, Magnetic and Electronic Properties Unveil Polaron Formation in Eu5In2Sb6, Sci. Rep., 2023, 13, 1597 Search PubMed.
- S. Roychowdhury, K. Samanta, P. Yanda, B. Malaman, M. Yao, W. Schnelle, E. Guilmeau, P. Constantinou, S. Chandra, H. Borrmann, M. G. Vergniory, V. Strocov, C. Shekhar and C. Felser, Interplay between Magnetism and Topology: Large Topological Hall Effect in an Antiferromagnetic Topological Insulator, EuCuAs, J. Am. Chem. Soc., 2023, 145, 12920–12927 Search PubMed.
- Bruker APEX4 Version 2009.5-1 Data Collection and Processing Software, Bruker Analytical X-Ray Instruments, Inc., Madison, WI, 2009 Search PubMed.
- G. M. Sheldrick, SADABS, 2008 Search PubMed.
- G. M. Sheldrick, XPREP Version 2008/2, Bruker AXS Inc., Madison, 2018 Search PubMed.
- G. M. Sheldrick, A Short History of SHELX, Acta Crystallogr., Sect. A: Found. Crystallogr., 2008, 64, 112–122 Search PubMed.
- G. M. Sheldrick, Crystal Structure and Refinement with SHELXL, Acta Crystallogr., Sect. C: Struct. Chem., 2015, 71, 3–8 Search PubMed.
- A. L. Spek, Single-Crystal Structure Validation with the Program PLATON, J. Appl. Crystallogr., 2003, 36, 7–13 Search PubMed.
- L. M. Gelato and E. Parthé, STRUCTURE TIDY – A Computer Program to Standardize Crystal Structure Data, J. Appl. Crystallogr., 1987, 20, 139–143 Search PubMed.
- R. A. Brand, WinNormos for Igor7 (version for Igor 7.010 or above: 01/03/2020), Universität Duisburg, Duisburg, Germany, 2020 Search PubMed.
- CorelDRAW Graphics Suite 2017 (version 19.0.0.328), Corel Corporation, Ottowa, Ontario, Canada, 2017 Search PubMed.
- H. Brasseur and L. Pauling, The Crystal Structure of Ammonium Cadmium Chloride, NH4CdCl3, J. Am. Chem. Soc., 1938, 60(12), 2886–2890 Search PubMed.
- C. R. Sankar, A. Assoud and H. Kleinke, New Layered-Type Quaternary Chalcogenides, Tl2PbMQ4 (M = Zr, Hf; Q = S, Se): Structure, Electronic Structure, and Electrical Transport Properties, Inorg. Chem., 2013, 52, 13869–13874 Search PubMed.
- K. O. Klepp and D. Sturmayr, Crystal Structure of Sodium Copper Triselenohafnate(IV), NaCuHfSe3, Z. Kristallogr. - New Cryst. Struct., 1997, 212, 75 Search PubMed.
- N. E. Brese and M. O’Keeffe, Bond-Valence Parameters for Solids, Acta Crystallogr., Sect. B: Struct. Sci., 1991, 47, 192–197 Search PubMed.
- A. Altomare, C. Cuocci, C. Giacovazzo, A. Moliterni, R. Rizzi, N. Corriero and A. Falcicchio, EXPO2013: A Kit of Tools for Phasing Crystal Structures from Powder Data, J. Appl. Crystallogr., 2013, 46, 1231–1235 Search PubMed.
- N. N. Greenwood and T. C. Gibb, Mössbauer Spectroscopy, Chapman & Hall, London, 1971 Search PubMed.
- F. M. Schappacher, R. Pöttgen, J. G. Bang and T. E. Albrecht-Schmitt, 151Eu and 121Sb Mössbauer Spectroscopy of EuSbSe3 and EuBiSe3, J. Solid State Chem., 2007, 180, 3035–3038 Search PubMed.
- O. Berkooz, Isomer Shifts of 151Eu in Divalent Europium Compounds, J. Phys. Chem. Solids, 1969, 30, 1763–1767 Search PubMed.
- N. R. Large, R. J. Bullock, P. Glentworth and D. A. Newton, Isomer Shift of the Mössbauer Spectrum of 151EuF3, Phys. Lett., 1969, 29A, 352–353 Search PubMed.
- P. Wachter, Handbook on the Physics and Chemistry of Rare Earths, Elsevier, Amsterdam, 1979, ch. 19, vol. 2, pp. 507–574 Search PubMed.
- D. X. Li, T. Yamamura, S. Nimori, Y. Homma, F. Honda and D. Aoki, Giant and isotropic low temperature magnetocaloric effect in magnetic semiconductor EuSe, Appl. Phys. Lett., 2013, 102(1–4), 152409 Search PubMed.
- R. Griessen, M. Landolt and H. R. Ott, A new antiferromagnetic phase in EuSe below 1.8 K, Solid State Commun., 1971, 9, 2219–2223 Search PubMed.
- G. Czjzek, V. Oestreich, H. Schmidt, K. Łątka and K. Tomalain, A study of compounds GdT2Si2 by Mössbauer Spectroscopy and by bulk magnetization measurements, J. Magn. Magn. Mater., 1989, 79, 42–56 Search PubMed.
- R. Pöttgen and D. Johrendt, Equiatomic intermetallic europium compounds: syntheses, crystal chemistry, chemical bonding, and physical properties, Chem. Mater., 2000, 12, 875–897 Search PubMed.
- I. A. Leahy, K. Feng, R. Dery, R. Baumbach and M. Lee, Field-induced magnetic states in the metallic rare-earth layered triangular antiferromagnet TbAuAl4Ge2, Phys. Rev. B, 2022, 106, 094426 Search PubMed.
- K. Feng, I. A. Leahy, O. Oladehin, K. Wei, M. Lee and R. Baumbach, Magnetic ordering in GdAuAl4Ge2 and TbAuAl4Ge2: Layered compounds with triangular lanthanide nets, J. Magn. Magn. Mater., 2022, 564, 170006 Search PubMed.
- M. O. Ajeesh, S. K. Kushwaha, S. M. Thomas, J. D. Thompson, M. K. Chan, N. Harrison, J. M. Tomczak and P. F. S. Rosa, Localized f-electron magnetism in the semimetal Ce3Bi4Au3, Phys. Rev. B, 2023, 108, 245125 Search PubMed.
- S. L. Bud’ko, P. C. Canfield, C. H. Mielke and A. H. Lacerda, Anisotropic magnetic properties of light rare-earth diantimonides, Phys. Rev. B: Condens. Matter Mater. Phys., 1998, 57, 13624 Search PubMed.
- S. Lucas, K. Grube, C.-L. Huang, A. Sakai, S. Wunderlich, E. L. Green, J. Wosnitza, V. Fritsch, P. Gegenwart, O. Stockert and H. V. Löhneysen, Entropy Evolution in the Magnetic Phases of Partially Frustrated CePdAl, Phys. Rev. Lett., 2017, 118, 107204 Search PubMed.
- L. Peters, S. Ghosh, B. Sanyal, C. van Dijk, J. Bowlan, W. de Heer, A. Delin, I. Di Marco, O. Eriksson, M. I. Katsnelson, B. Johansson and A. Kirilyuk, Magnetism and exchange interaction of small rare-earth clusters; Tb as a representative, Nat. Sci. Rep., 2016, 19676 Search PubMed.
- P. Park, G. Sala, T. Proffen, M. B. Stone, A. D. Christianson and A. F. May, Quantum magnetism in the frustrated square lattice oxyhalides YbBi2IO4 and YbBi2ClO4, Phys. Rev. B, 2024, 109, 014426 Search PubMed.
Footnote |
† Electronic supplementary information (ESI) available: The SEM data of the elemental composition, the atomic displacement parameters, the metric details of the final refined structure, and additional magnetic and thermodynamic data. CCDC 2328994. For ESI and crystallographic data in CIF or other electronic format see DOI: https://doi.org/10.1039/d4tc01750a |
|
This journal is © The Royal Society of Chemistry 2024 |