DOI:
10.1039/D4VA00109E
(Critical Review)
Environ. Sci.: Adv., 2024,
3, 1197-1216
A review on waste biomass-to-energy: integrated thermochemical and biochemical conversion for resource recovery
Received
8th April 2024
, Accepted 27th May 2024
First published on 29th May 2024
Abstract
Improving energy security and lowering greenhouse gas emissions need the utilization of renewable resources like biomass. The production of power, heat, and biofuels from biomass has gained significant attention in the current energy scenario. The current study highlights the developments, advancements, and future possibilities of merging thermochemical and biochemical conversion processes for the manufacture of value-added chemicals and green fuels. While biological processes have extensive processing times and low product yields, thermochemical methods are limited by high processing costs and temperature requirements. The integration of thermochemical and biochemical conversion processes facilitates the circular economy and improves resource usage. Despite the wide range of feasible integration scenarios, the majority of research that is now accessible in the literature concentrates on the developments in thermochemical or biochemical processes as a standalone conversion pathway. The present review aids in gaining a basic understanding of potential routes to unlock pathways for complete biomass conversion. Pyrolysis, as well as hybrid conversion techniques, are the most appealing methods from an economic evaluation standpoint. In this work, a techno-economic analysis of the significant conversion processes has also been presented. Examining the environmental impact and costs of alternative waste conversion processes is necessary when obtaining energy from garbage or biomass. So, life cycle assessment (LCA) is a useful method for comparing the environmental effects of various waste-to-energy options. To increase the production of biofuels, further research is required in the areas of feedstock pretreatment, catalyst development, and total production system optimization.
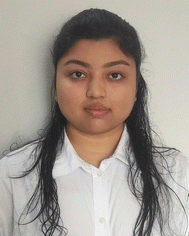 Yasmin Ara Begum | Ms Yasmin Ara Begum, an aspiring scholar, is currently pursuing her Master of Technology in Environmental Engineering at Amity University, Noida, Uttar Pradesh. Her academic journey showcases a relentless pursuit of knowledge and excellence. With a prior accomplishment of a Master of Science in Environmental Sciences and a Bachelor of Vocation in Renewable Energy Management from Tezpur University, Assam, she has consistently displayed remarkable dedication and proficiency in her field. Her commitment to environmental sustainability and her academic prowess have been evident throughout her educational endeavours, reflecting her passion for making a positive impact on the world through her expertise. |
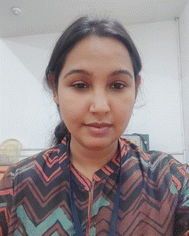 Sheetal Kumari | Ms Sheetal Kumari is currently pursuing her PhD at the Amity Institute of Environmental Science, Amity University, Noida, India. Ms Sheetal has completed her MSc (Environmental Science) from Maharishi Dayanand University, Rohtak and her BSc (Life Science) from the University of Delhi, New Delhi, India. Her areas of interest are adsorption, wastewater treatment, biochar, pyrolysis, and artificial intelligence. She has published 7 articles in peer-reviewed journals. |
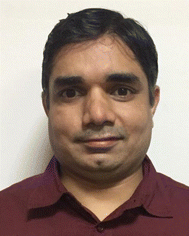 Shailendra Kumar Jain | Dr Shailendra Kumar Jain is currently an Associate Professor at the Department of Civil Engineering, Amity University Uttar Pradesh, Noida, UP. He holds an M.Tech. and PhD from the Indian Institute of Technology, Delhi. He has experience of working in teaching and research in the fields of water resources and environmental engineering and his major areas of research interest include municipal solid waste management, green buildings, and wastewater management. He has more than 18 years of experience in teaching and research. He has numerous experiences of attending and presenting technical papers in national and international conferences. |
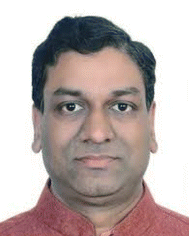 Manoj Chandra Garg | Dr Manoj Chandra Garg, an Assistant Professor at Amity Institute of Environmental Sciences, Amity University Uttar Pradesh, India, holds a PhD in Environmental Science and Technology from the Indian Institute of Technology, Roorkee. With over 10 years of teaching and research experience, he specializes in water quality, membrane filtration, solar desalination, and machine learning. Dr Garg has published extensively, with notable achievements including 40 journal publications and 5 patents. He has received prestigious awards such as international travel grants and academic accolades, demonstrating his commitment to environmental research and education. |
Environmental significance
The integration of thermochemical and biochemical conversion processes for biomass represents a crucial step in addressing energy security, greenhouse gas emissions, and sustainable resource management. By harmonizing these methodologies, this study delineates pathways for comprehensive biomass utilization, yielding value-added chemicals and green fuels. This approach not only tackles the inefficiencies of standalone processes but also promotes a circular economy model, optimizing resource usage. Advancing biofuel technologies and refining waste-to-energy systems emerge as pivotal strategies, crucial for mitigating environmental degradation and fostering sustainability. This research underscores the urgent need to address these challenges, emphasizing the potential for transformative change towards a greener, more resilient future.
|
1. Introduction
The need for a transition to sustainability is growing due to global climate change and the depletion of natural resources. This urgency is made even more pressing by the world's population growth and rapid industrialisation, which together present serious obstacles to the disposal of biowaste and rising energy consumption.1 Agriculture is one of the key contributors to the Indian economy. The accelerated growth in the human population in recent times has led to a rapid increase in the requirement of food for our survival. As a result, the demand for agricultural production has increased manifold.2 Global agriculture generates 23.7 million tons of food every day on average, according to a 2017 report released by the Food and Agricultural Organization.3 The increased agricultural production can be attributed to several factors and this growth has caused negative impacts on the environment. Depending on the kind of agricultural produce or product, processing methods, and intended use, every stage of production, processing, and consumption results in significant waste creation.4 However, due to a lack of a proper waste management system, these wastes consisting of husks, seeds, leaves, stalks, pulp, stem, bagasse etc. are left underutilized. This causes a lower return from the wastes as agricultural wastes have a highly nutritious composition and can be used for feeding animals, manufacturing fertilizers, soil improvement, production of biogas and bioethanol, as alternative substrates for fermentation and various other commercial purposes.5 If not managed properly, serious health issues are caused by these wastes through environmental pollution. Agricultural productivity needs to be improved due to the adverse impact of agricultural activities on the environment, aquatic life, and human health. Thus, the development of efficient and effective methods for managing agricultural solid wastes is much needed.6 Agricultural residues can be managed effectively by recycling them to produce useful products. The lignocellulosic biomass present in the natural environment is recognized as a highly important renewable resource due to its ability to generate biofuels and high-value materials.7 Pyrolysis is one of the methods for converting biomass that has attracted a lot of interest since it may directly transform lignocellulosic biomass into useful byproducts.1
Fuel for transportation is produced from biomass, which comes from renewable sources. Biomass components like cellulose, hemicellulose, and lignin which are found in lignocellulosic compounds can be used to generate transportation fuels that could replace fossil fuels to some extent.8 One feasible option to lessen the environmental effects of waste management and disposal is to generate bioenergy from biomass buildup and a variety of wastes, such as food waste, animal manure, urban garbage, and rural waste.9 The thermochemical conversion of waste into biochar ensures a dramatic reduction in waste volume. This in turn helps in creating a pollution-free environment.
A porous carbonaceous substance known as “biochar” is produced when biomass feedstock is thermochemically broken down in an oxygen deficient environment.10 The biomass feedstock encompasses a variety of organic sources like residues from crops and forests, sewage sludge, wood chips, algae, and municipal solid wastes that are organic.11,12 Various processes are used for the thermochemical breakdown of biomass like hydrothermal carbonization, pyrolysis, torrefaction, gasification, and microwave heating. All these methods differ in their thermochemical temperature and duration.13,14 So far, several research studies and reviews have been carried out on biochar based on its two distinct merits: firstly, through stable carbon storage, biochar can mitigate greenhouse gas emissions by halting the atmospheric release of greenhouse gases that arise from the breakdown of biomass; secondly, owing to its numerous surface functional groups and comparatively wide surface area, this adsorbent is cheap, effective and environmentally benign.15,16 Several review articles on biofuels and bioenergy have been published, as summarized in Table 1.
Table 1 Summary of review works on biofuels and bioenergy
Title |
Objectives |
Major revelations |
References |
A review on regeneration of biowaste into bio-products and bioenergy: life cycle assessment and circular economy |
A brief review of the various forms of biowaste, their sources, and composition was conducted. A detailed discussion of the various reaction mechanisms for the biowaste conversion process was held |
A significant portion of biowaste that can be used as a substrate for the production of bioenergy and bioproducts is made up of food and agro-industrial wastes. A lifecycle analysis of several biowaste conversion processes revealed them as environmentally and socially sustainable processes |
17
|
A critical review of biochemical pathways to the transformation of waste and biomass into bioenergy |
This study explores the most recent methods for producing bioethanol and bioenergy from lignocellulose biomass, including anaerobic digestion, microbial fuel cells, fermentation, and hydrolysis of enzymes |
Biological processes produce more volatile organic compounds (VOCs) than thermochemical ones, which need high temperatures and significant energy. Future energy demands can be fulfilled by utilizing nanoparticles, which have the potential to revolutionize biogas production in terms of both quality and quantity |
18
|
A review of biomass-to-bioenergy supply chain research using bibliometric analysis and visualization |
To encourage significant research in both expanding and underutilized fields of study, the literature on 1711 peer-reviewed articles that were published between January 1992 and August 2022 was analyzed using sophisticated bibliometric analysis and visualization |
The results suggest that there might be opportunities and research gaps in six important areas: the study of multi-feedstock systems; the use of inventory control techniques; the globalization of supply chain research; the inclusion of risk, uncertainty, and stochasticity in supply chain models; and the broader use of artificial intelligence (AI) and machine learning (ML) in this field |
19
|
Biogas (a promising bioenergy source): a critical review on the potential of biogas as a sustainable energy source for gaseous fuelled spark ignition engines |
The review focused on the use of biogas in gaseous-fuelled spark-ignited engines and how it's contributing to the attainment of sustainable development goals. The most recent developments in biogas generation and upgrading methods are also covered in detail |
Apart from being an appealing fuel for SI engines, biogas and biomethane will be crucial in creating the foundation for a sustainable energy future. Biogas is one of the more promising green and renewable energy-based solutions because, by using agricultural crop residues and other domestic biomass sources as raw materials, it can be used for power generation applications (engines driving generators and pump sets) at home and industrial scales with less capital investment and production costs. Nevertheless, the raw materials used to produce the biogas change its composition, and a higher carbon dioxide content in the biogas causes variations in combustion that shorten engine life |
20
|
Exploitation of lignocellulosic-based biomass biorefinery: a critical review of renewable bioresource, sustainability and economic views |
This review examines the benefits, drawbacks, and characteristics of pretreatment techniques, encompassing chemical, biological, physical, and physico-chemical approaches. This study offers a thorough examination of the biorefining of lignocellulosic biomass, and it also examines the use of green biorefinery technologies, which are thought to be sustainable and may have positive effects on the environment, the economy, and society |
Considering its inexpensive, renewable, high energy-density raw materials, lignocellulosic biomass is one of the most abundant sources and presents a viable substitute for fossil fuels |
21
|
A review on bio-slurry fuels derived from bio-oil and biochar: preparation, fuel properties and application |
The impact of biochar on the bio-slurry was examined, along with the effects of bio-oil and its fractions, methanol, glycerol, and crude glycerol, as well as loading level, adsorption capacity, and particle size |
Although bio-oil has the potential to replace fossil fuels in stationary applications, its poor heating value, corrosiveness, and ageing susceptibility prevent it from being widely used as a fuel. The most significant application of bio-slurry, whether for the manufacture of chemical compounds or effective electricity, is gasification. Because biochar is present, slurry has a higher density, viscosity, and heating value than bio-oil |
22
|
Environmental assessment of hydrothermal treatment of wet bio-residues from forest-based and agro-industries into intermediate bioenergy carriers |
This research uses LCA to quantify the environmental effects of a novel hydrothermal process for treating three wet biogenic residues (orange peel, paper bio-sludge, and olive pomace) into bioenergy carriers, such as solid pellets and biogas, on an industrial scale |
The environmental performance of the three residues varies, though, which implies that mitigation strategies might be required for particular environmental impact categories, such as human toxicity from olive pomace and orange peel and terrestrial acidification from orange peel. The pulp and paper and steel industries stand to gain the most, as they could, respectively, (i) avoid the disposal of biological sludge by producing solid fuels that serve the internal energy production system or the local (bio)fuel market using locally produced and currently unvalued residue streams, and (ii) benefit from the utilization of bio-based carbon sources that may substitute fossil cokes in the steel-making process |
23
|
A review on bioenergy and biofuel production |
This review compares the performance of homogeneous and heterogeneous catalysts |
It was discovered that while a heterogeneous catalyst operates at peak temperatures, a homogeneous catalyst performs better at lower temperatures and can be recycled. Many writers have discussed the use of homogenous catalysts; nevertheless, it has been noted that these catalysts are costly and difficult to reuse or recycle. Catalysts of the heterogeneous type generate results efficiently and can be reused. Utilizing nanocatalysts, which lie between homogeneous and heterogeneous catalysts, offers effective, contemporary techniques for producing biofuels with low energy content |
24
|
Optimizing biomass pathways to bioenergy and biochar application in electricity generation, biodiesel production, and biohydrogen production |
The review presents different methods for turning biomass into bioenergy and biochar, as well as how they can be used to make electricity, biodiesel, and biohydrogen |
Biofuels generated from biomass can lessen reliance on fossil fuels and greenhouse gas emissions. Currently, biochemical conversion accounts for around 80% of all biofuels generated globally. The two most common biofuels produced by biochemical conversion processes are ethanol and biodiesel. About 20% of the biofuels generated globally come via thermochemical conversion, which is less common than biochemical conversion. By 2050, biofuels derived from biomass might account for up to 27% of global transportation fuel consumption, resulting in a 3.7 billion metric ton annual reduction in greenhouse gas emissions |
25
|
Recent advances and sustainable development of biofuel production from lignocellulosic biomass |
Recent developments in pretreatment techniques for improved biofuel production are highlighted in this review. Comprehensive explanations of the biomass processing pathway's mechanism, optimization, and modelling studies have been covered |
The potential for effective biofuel production has been proved by recent advancements in lignocellulose bioconversion processes. With the use of contemporary synthetic biology technologies, it is possible to synthesize biofuel feedstock that is not produced naturally. However, the use of genetic and metabolic engineering techniques to modify and examine metabolic pathways to improve the production of biofuels will be regarded as an important and novel approach |
26
|
Recent advances in biodiesel production: challenges and solutions |
This article provides a comprehensive assessment of the various substrate issues, new developments, constraints, and difficulties associated with the manufacture of biodiesel |
A key to significant economic development may be the creation of a sustainable and efficient biodiesel production method. Therefore, intensive study on the feedstock supply barrier, process innovation (e.g., reactor layout that can endure extreme conditions), or the development of an enzyme that is affordable would be needed |
27
|
Mitigation of CO2 emissions by transforming to biofuels: optimization of biofuels production processes |
The primary parameters impacting the statistical optimization of transesterification processes and the optimization of biodiesel production are the focus of this article |
Water, alcohol, free fatty acids (FFA), and glycerol are contaminants found in biodiesel. Water cleansing, evaporation, or distillation may minimize these pollutants |
28
|
From waste to fuel: challenging aspects in sustainable biodiesel production from lignocellulosic biomass feedstocks and role of metal–organic framework as innovative heterogeneous catalysts |
This review offers a comparative analysis of several feedstocks about diverse heterogeneous catalysts for the transesterification reaction and their impact on the yield of biodiesel |
The need for liquid fuels for transportation is growing exponentially on a global scale, and biodiesel has shown promise in meeting this demand. Although lignocellulosic biomass is an inexpensive feedstock for the manufacture of biodiesel, the high cost of its processing makes large-scale production difficult. On the other hand, using alternative energy sources can assist in resolving this issue in an eco-friendly manner |
29
|
Utilization of bioresources for high-value bioproducts production: sustainability and perspectives in circular bioeconomy |
In the framework of the economy, this paper aims to provide an overview of the prospects for bioresources that could support the production of high-value products, progressing toward sustainable bio-products |
Harnessing bioresources to produce high-value bioproducts has a lot of potential as a sustainable and forward-thinking tactic within the context of the circular economy |
30
|
Influencing factors and environmental feasibility analysis of agricultural waste preprocessing routes towards biofuel production – a review |
This paper offers in-depth details on the chemical composition, potential availability of agricultural waste, and several preparation methods |
The composition of the lignocellulosic biomass, the process's pH, the operating temperature, and the duration of time significantly affect how effective preprocessing techniques are |
31
|
This highly porous and carbon-rich material can also be used to purify water by adsorbing metals/metalloids.32 Additionally, it can be applied on soils as a catalyst and an adsorbent for a wide range of pollutants to increase crop yield, soil productiveness, thus helping to minimize greenhouse gas emissions and partially replace fossil fuels.33–35 Consequently, biochar is gaining importance as a remedial solution for several worldwide issues, including soil degradation, pollution, and climate change.10Fig. 1 displays the publication trends from 2019 to 2024 and a word cloud showcasing the most frequently used terms. A publication analysis was conducted to examine research trends and identify areas lacking in the literature, thus providing the framework for future study.
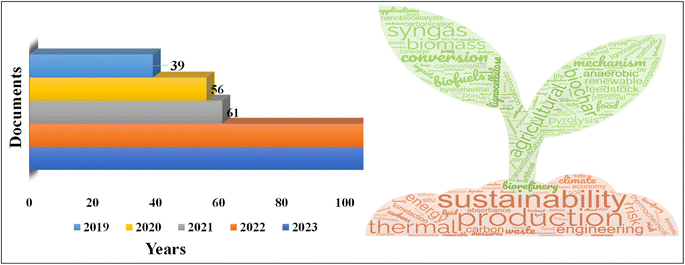 |
| Fig. 1 Number of publications from 2019–2024 and word cloud of most repeated keywords. | |
2. Sources of waste biomass/feedstock
The diverse categories of feedstocks that affect the physicochemical properties of biochar have been covered in several prior review publications.36 The main components of lignocellulosic biomass are lignin, cellulose, and hemicellulose. In addition to water in the form of moisture and ash, it also contains other plant elements in trace quantities like lipids, starch, sugars, oils, and acids. Worldwide crop residue production is millions of tons (5280 megatons in 2020–21).37 Lignocellulosic biomasses like rice straw, wheat straw, corn stover, and sugarcane bagasse dominate agricultural waste. WW is produced by processing wood resources such as leaves, branches, bark, sawdust, and shavings. WW includes wasted furniture, building materials, pallets, and wood packaging. Since timber harvesting eliminates 25% to 50% of the volume, waste wood is an important energy resource. Day-to-day FW production occurs in retail, food service, farming, post-harvesting, packing, processing, distribution, and disposal.38 To reduce waste and improve environmental sustainability, sustainable food processing techniques can be developed, cooking oil can be turned into biofuel, and food donation programs can be organized. Without treatment, animal wastes like manure and faeces can pollute water and eutrophicate surface water due to their high nitrogen and phosphate content.6
Following recommendations in the literature,40–42 biomass materials have been organized into groups as detailed in Fig. 2. Significant differences exist in the overall characteristics and molecular makeup of herbaceous and woody biomass, as seen in Table 2.
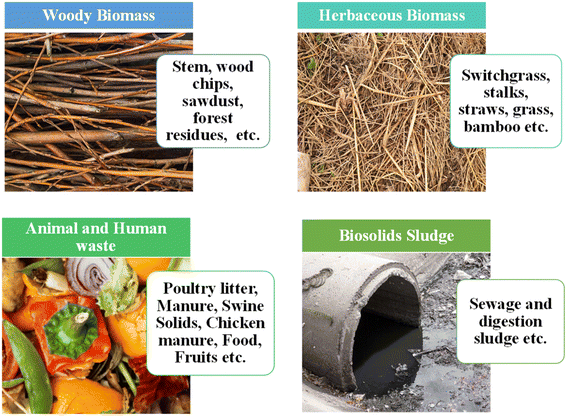 |
| Fig. 2 Biomass feedstock categories.39 | |
Table 2 Composition and properties of woody and herbaceous biomass groups43
Biomass type |
Volatiles (wt%) |
Ash (wt%) |
Fixed carbon (wt%) |
Cellulose (wt%) |
Hemicellulose (wt%) |
Lignin (wt%) |
Woody biomass |
84.0(2.1) |
1.3(0.9) |
14.7(1.6) |
51.2(8.7) |
21.0(8.7) |
26.1(5.3) |
Herbaceous biomass |
79.1(5.8) |
5.5(3.2) |
15.4(4.0) |
32.1(4.5) |
18.6(3.4) |
16.3(3.3) |
When choosing methods, these differences need to be carefully considered to guarantee the smooth manufacture of biochar with the appropriate qualities. The overall composition can vary significantly, even within the same biomass group.
3. Bioenergy
Biofuels represent a category of sustainable fuels sourced from abundant biological materials known as biomass. This biomass encompasses diverse resources, including forestry products, agricultural residues such as straw, husks, and animal fat byproducts from food processing, industrial waste from pulp production and sewage, waste wood from construction and demolition and biodegradable waste.44 Additionally, biofuels can be obtained from specific energy crops cultivated exclusively for energy or transport fuel purposes, as well as from algae, microalgae, and seaweed.45 Biofuels encompass a broad spectrum of fuel types, including biogas, biodiesel, bioethanol, biohydrogen, and others. Three primary categories of biofuels can be distinguished by their constituent parts and physical states: solid, liquid, and gaseous biofuels. Typically, processed biomass, raw biomass fuels, and leftovers from biomass conversion operations are all considered solid biofuels. However, gaseous and liquid biofuels—known as syngas and bio-oil, respectively—come from the conversion of biomass.46 Production of bioenergy has been considered a net zero carbon emission technique since carbon emitted during energy transformation is assumed to have been taken up by biomass during the process of photosynthesis. Thus, during energy conversion, the carbon dioxide taken from the atmosphere during photosynthesis is released.
3.1. Production of bioenergy
Biomass can serve directly as a fuel or can be transformed into liquid or gaseous forms, making it an important source of energy for producing chemicals, heat, electricity, and liquid and gaseous fuels.45 There are two primary methods of converting biomass into biofuels: thermochemical conversion and biochemical conversion.47 In contrast, there are five subcategories of thermochemical conversion: gasification, pyrolysis, carbonization, liquefaction, and combustion. Thermochemical conversion technologies are generally considered more efficient and flexible than their biochemical counterparts.48 The global population hit 8.0 billion in mid-November 2022 and is expected to reach 9.7 billion by 2050, according to a reputable source.49 Population growth affects food, water, and energy supplies. The effective management of all waste, including biomass waste like sewage sludge, wood, food, municipal solid trash, and agricultural waste, is crucial. Landfilling, composting, fertilizing, and foddering are common waste management strategies.50 All of these technologies are unsustainable due to greenhouse gas emissions, surface and groundwater contamination, and disease growth. Composting uses nutrient-rich organic wastes like sewage sludge, animal manure, agricultural residues, and more to improve soil quality. Methane emissions, foul odours, and toxic leachate are released throughout the lengthy process. However, organic waste is dumped in landfills,50 harming the ecosystem and wasting resources. Modern sustainable waste treatment systems focus on waste conversion into energy, fuels, and goods to maximize biomass resources and create a circular economy around lignocellulosic biomass.7 This technology is thought to process biomass and organic wastes more efficiently. Depending on the organic waste feedstocks, biomass wastes can be used and handled in various ways. However, these approaches may not solve all issues. When handling inevitable biomass wastes, an integrated multi-technology strategy is crucial.
4. Waste-to-energy conversion routes
4.1. Thermochemical conversion processes
Chemical energy that has been stored during photosynthesis is released by the breakage of bonds between neighbouring molecules of carbon, hydrogen, and oxygen in thermochemical conversion pathways.51 The thermochemical conversion routes concentrate on transforming organic matter into solids (like hydrochar or biochar), liquid fuels (such as hydrocarbon fuels) and bio-oils or gases (such as syngas), as well as their intermediates.52 Thus, biochar, bitumen, bio-oils, and incompressible gases are the usual end products of thermochemical conversion processes.47
These are effective methods available to safely dispose of waste biomass and maximize its economic value. Through the use of scientific methods, these technologies can reduce the reliance on non-renewable resources such as fossil fuels by converting biomass into alternative fuels.53 All the conversion technologies that have been discussed previously have inherent limitations, regardless of their scientific approach.54 There are drawbacks to both thermochemical and biochemical approaches. Thermochemical methods often lead to the production of low-quality biofuel and come with high costs in terms of production and energy. Additionally, they contribute to greenhouse gas emissions and require gas purification.55 In contrast, the alternative method is less efficient in breaking down resistant biomass materials, takes longer to complete, and has a lower production rate.54
Biochar or pre-treated biomass correlates with torrefied biomass, pyrochar (derived from slow pyrolysis), and hydro chars (produced from hydrothermal carbonization). Heating rate, residence duration, temperature and pressure are among the factors that determine this transformation.20 There are three main groups of thermochemical conversion pathways based on the primary product they produce. The first group is biomass to solid processes which involve torrefaction and hydrothermal carbonisation. The second group is biomass to liquid processes which include hydrothermal liquefaction, pyrolysis (fast and flash), and slow pyrolysis. The third group is biomass to gas processes which include hydrothermal gasification and conventional gasification.56Table 3 contains a list of all thermochemical transformation processes along with their characteristics and reaction products. Temperature, residence time and heating rate play a crucial role in all thermochemical conversions. Fig. 3 represents the concept of thermochemical conversion processes of biomass.
Table 3 Thermochemical conversion processes and products57–59
Thermochemical conversions |
Temperature (°C) |
Heating rate (°C min−1) |
Aeration oxygen |
Residence time (min) |
Major product |
Bio-char yield (%) |
Byproduct |
Slow pyrolysis |
350–700 |
<10 |
Oxygen-free or limited |
>60 |
Biochar |
30–55 |
Bio-oil-combustible gas |
Fast pyrolysis |
400–600 |
>200 |
Oxygen-free |
∼0.02 |
Bio-oil |
10–26 |
Combustible gas |
Flash pyrolysis |
750–1000 |
>1000 |
Oxygen-free |
∼0.04 |
Bio-oil |
37 |
— |
Gasification |
800–1600 |
∼1000 |
Oxygen-free or limited |
0.2–0.4 |
Syngas |
14–25 |
Biochar-tars |
Torrefaction |
200–300 |
<50 |
Oxygen-free |
15–60 |
Torrefied biomass |
69–80 |
None |
Hydrothermal carbonization |
400–1000 |
<1 |
— |
5–240 |
Hydrochar |
50–79 |
— |
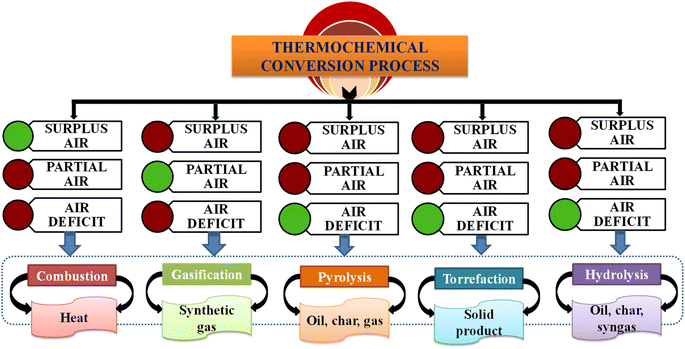 |
| Fig. 3 Concept of thermochemical conversion processes of biomass. | |
4.2. Biochemical conversion processes
Biochemical conversion methods, consisting of anaerobic digestion and syngas fermentation, involve the breakdown of non-woody organic materials in the absence of oxygen under mild conditions. The final products like methane and carbon dioxide are usually stable compounds.47 However, biochemical conversion processes have limitations, as they primarily utilize cellulose and hemicellulose components of biomass and tend to be time-consuming. The key challenges for biochemical conversion include the considerable cost and difficulty involved in breaking down the tough, complex structures of the cell walls in cellulosic biomass.53 Another key challenge is to more efficiently convert the sugars into biofuels and purify them. Anaerobic digestion is a biological conversion process which combines the processes of hydrolysis, acidogenesis, acetogenesis, and methanogenesis to convert organic biomass into methane-rich biogas.60 The process yields digestate, with potential uses such as as a fertilizer and value-added products.61 In comparison to aerobic systems, anaerobic systems are preferable because they can handle organic residues that are difficult to decompose, consume less energy, produce less sludge, remove pathogens, and have less odour.62,63 Syngas fermentation is a recently developed method that uses microorganisms to help transform gaseous mixtures (called syngas) that contain H2, CO, and CO2 into fuels and compounds with additional value in an oxygen-poor environment. Acetic acid, formic acid, methane, butanol, and ethanol are the main byproducts of SNF. Gas for SNF is produced via pyrolysis, electrochemical syngas production, biomass gasification, and industry exhaust gases.
4.3. Biochemical–thermochemical hybrid conversion
4.3.1. Pyrolysis–anaerobic digestion.
Anaerobic digestion produces digestate, which may be dehydrated and used as a fuel in pyrolysis processes to create biochar. This integrated method improves energy sustainability while lowering total expenses. The use of AD and pyrolysis together has been studied by researchers to enhance the recovery of resources from agricultural waste (Fig. 4). When the digestate from AD is dried and utilized as feedstock for pyrolysis, the electricity gain increases by 42% as compared to AD alone.64 The sale of extra electricity to the grid through this integration also brings in extra money. Comprehending the characteristics of pyrolysis products and digestate's thermal decomposition behaviour is essential for techno-economic research and process optimization.65 It has been investigated to enhance the quality of bio-oil by pretreating biomass before pyrolysis. Comparing solid products from AD with digestate pyrolysis, biochar performs better as a soil conditioner because of its higher potassium and phosphorus content, larger surface area, and improved ability to hold water.66 Several studies have been carried out on hybrid pyrolysis–anaerobic digestion processes using different feedstocks (Table 4).
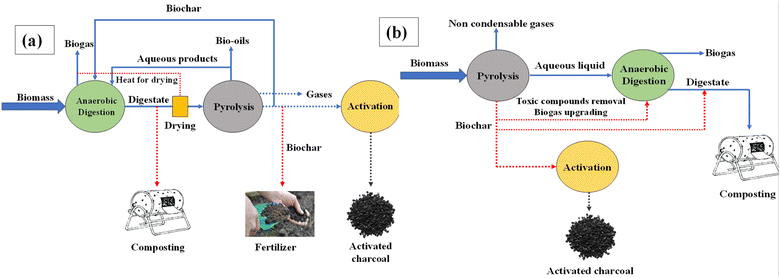 |
| Fig. 4 (a) Flow diagram for hybrid AD and pyrolysis digestion process; (b) flow diagram for hybrid pyrolysis and AD digestion process. | |
Table 4 Impact of different operating conditions on hybrid pyrolysis–AD technology
Pyrochar feedstock |
Operational conditions for pyrolysis (temperature and residence time) |
Operational conditions for AD (type, mode, temperature, substrate) |
Effect of pyrochar on anaerobic digestion |
References |
Saw dust |
500 °C, 60 min |
Thermophilic, batch, 55 °C, waste-activated sludge with food waste |
It reported a decline in the lag phase, an increase in the rate of CH4 synthesis, and the adaptability of microorganisms to highly volatile organic compounds through pH control using pyrochar |
71
|
Leaf waste |
200 to 400 °C, 180 min |
Mesophilic, N.A., N.A., biogas with 500 to 1300 ppm H2S |
It demonstrated a significant level of H2S adsorption for around 30 minutes before saturation |
72
|
Saw dust |
500 °C, 90 min |
Mesophilic, batch, 35 °C, food waste with dewatered activated sludge |
It showed a reduction in the lag phase, an increase in buffering capacity, and an improvement in CH4 formation, all of which helped to facilitate pH reduction |
73
|
Dairy manure, waste sludge |
400 to 800 °C, 90 min |
Mesophilic, batch, 37 °C, waste activated sludge |
It revealed the adsorption of heavy metals, particularly lead, on pyrochar |
74
|
Vermi-compost |
500 °C, 120 min |
Mesophilic, batch, 35 °C, chicken manure with kitchen waste |
It revealed a notable rise in CH4 production along with enhanced buffering capability |
75
|
Coconut shell, rice husk, wood |
450 °C |
Mesophilic, batch, 35 °C, citrus peel |
It revealed a minor rise in CH4 along with more methanogen colonies, a shorter lag time, and more removal η |
76
|
Studies that used digestate derived from the anaerobic digestion of food waste as fuel have also looked into how AD affects the yield of pyrolysis. While raw food waste can be pyrolyzed to produce more gas and bio-oil, digestate-derived bio-oils have decreased quantities of hydrocarbon derivatives, phenols and esters. Digestate pyrolysis produces bio-oils that have similar qualities to biodiesel and can be used as fuel for vehicles.67 Improved biofuel recovery results from syngas biomethanation, which uses anaerobic microorganisms to convert CO2, H2, and CO into CH4.68 Furthermore, liquid digestate and biochar from solid digestate pyrolysis can be mixed to improve soil.69
Overall, the integration of AD and pyrolysis presents a promising approach to enhance resource recovery, reduce waste, and promote sustainability in the energy and agricultural sectors.70
4.3.2. Hydrothermal liquefaction–anaerobic digestion.
There is an opportunity to improve the energy output from organic waste by combining HTL with AD. Some research work has been carried out utilising this hybrid technology as in Table 5. Anaerobic digestate management and disposal provide difficulties from an environmental and economic standpoint, with possible problems relating to odour, pollution of the environment, and the presence of pathogens.77 Utilizing digestate as liquid fertilizer in agriculture may lead to excess dosage concerns and contribute to overall process costs.78 Due to its high organic content, anaerobic digestate can be used to produce biocrude, a valuable liquid fuel, through HTL.79 This biocrude can subsequently undergo upgrading to produce green transportation fuels. One benefit of HTL is that it may be used with feedstocks that have a high moisture content, which means it doesn't need to be dried and is especially good for anaerobic digestate. Post-HTL wastewater is a viable feedstock for biological processes since it preserves up to 40% of organic matter and at least 80% of the nutrients from the original feedstock.80 Additionally, because of its high nutritional concentration, this effluent may be used in the development of algae.79 Anaerobic bacteria are more resilient to the diverse chemical compounds in post-HTL effluent than other strains.81,82 An inventive method for supplying nutrients and recovering energy from post-HTL wastewater combines algae farming with AD. As a detoxifying stage, the AD process gets rid of organic contaminants that can prevent algae from growing. To cleanse wastewater and produce a reduced post-hydrothermal liquefaction wastewater dilution ratio appropriate for the cultivation of algae, the study uses a detoxification approach that combines ozone and granular activated carbon. Methane output and overall energy recovery are greatly increased by the AD process's inclusion of activated carbon. Effective material usage and resource recovery are two benefits of the integrated HTL–AD process.80Fig. 5(a) represents the flow diagram for the hybrid hydrothermal liquefaction and AD process.
Table 5 Methane yields from anaerobic digestion of the HTL aqueous phase under different conditions
HTL feedstock |
HTL temperature |
HTL-AP COD (g L−1) |
AD operation mode |
AD loading (gCOD L−1 for batch, gCOD L−1 d−1 for continuous/semi-continuous) |
Co-treatment or pretreatment |
Highest methane yield observed |
References |
Granulated activated carbon.
|
Chlorella 1067 |
300 |
— |
Batch |
2 to 5 |
Zeolite adsorption before AD |
84% at 4 g L−1 |
83
|
Dewatered sewage sludge |
140–320 |
|
Batch |
0.75 |
— |
82% at 170 °C |
84
|
Swine manure |
270 |
— |
Batch |
0, 5, 10, 20 |
GACa addition, ozone pretreatment, and a combination of GAC and ozone |
62% at 20 gCOD L−1 with GAC addition |
|
Algae Chlorella and Tetraselmis |
— |
75 and 85 |
Semi-continuous |
0.8–1.5 |
Co-digestion with clarified manure |
69% at 26% v/v Chlorella, 89% at 22% v/v Tetraselmis |
85
|
Cornstalk |
260 |
— |
Continuous |
8 |
|
N.A. (67–70% COD removal rate) |
86
|
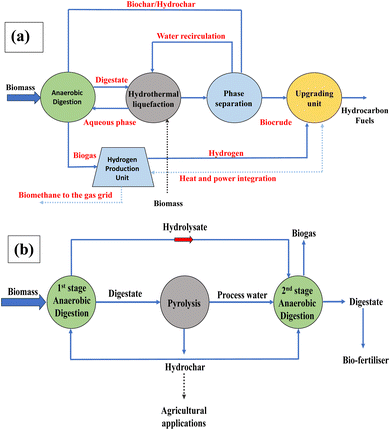 |
| Fig. 5 (a) Flow diagram for the hybrid hydrothermal liquefaction and AD process; (b) flow diagram for the hybrid AD and hydrothermal carbonization process. | |
4.3.3. Hydrothermal carbonization–anaerobic digestion.
Anaerobic digestate can be efficiently combined with anaerobic digestion downstream to create hydrochars, which can be used as fuel or in a variety of industrial processes. Numerous studies have looked into the possibility of HTC to improve anaerobic digestate. In addition to creating carbon-rich hydrochar, the HTC process yields organic-dissolved process water that aids in chemical and nutrient recovery.87 This integrated approach demonstrates the versatility of HTC in maximizing the utilization of anaerobic digestate while promoting resource recovery and sustainability.88,89 It is crucial to understand that the chemical structure and composition of pyrochar and hydrochar are different.90 Because of the higher temperatures utilised in pyrolysis, pyrochar is formed with a strong carbon structure which is less prone to microbial destruction. HTC hydrochars, however, don't have these characteristics. The energy efficiency of combined HTC and AD processes has been acknowledged, yet process stability may be hampered by the repetitive input of aqueous liquid of HTC back into the AD system. This is explained by the constant changes in the content of digestate and aqueous liquids and microbial sensitivity.91 Consequently, a preferable approach involves the integration of a two-stage AD.92 Many studies have been carried out using different feedstocks for combined HTC-AD conversion technology (Table 6).
Table 6 COD removal and methane production in anaerobic digestion of process water from HTC
|
AD of process water from HTC |
AD feedstock |
|
Biomass waste |
Temperature (°C) |
Time (min) |
COD removal (%) |
Methane yield (mL CH4 g−1 COD added) |
Energy recovery (%) |
Methane yield (mL CH4 g−1 COD) |
Energy recovery (%) |
References |
Primary sewage sludge |
160 |
30 |
61 |
259 |
13.4 |
137 |
13.2 |
93
|
Secondary sewage sludge |
160 |
30 |
51 |
258 |
15.3 |
120 |
4.3 |
93
|
Mixed sewage sludge |
160 |
30 |
66 |
280 |
20.1 |
253 |
8.1 |
93
|
Digestate |
200 |
60 |
50 |
297 |
9.1 |
— |
— |
94
|
Water hyacinth |
200 |
60 |
61 |
213 |
13.9 |
103 |
28 |
95
|
Microalgae |
150 |
60 |
— |
230 |
18.8 |
200 |
34.2 |
96
|
Cattle manure |
160 |
60 |
49 |
294 |
18.8 |
111 |
25.6 |
97
|
Because of the constant addition of HTC aqueous liquid, which introduces dynamic variations, it is believed that this two-stage AD design is a superior option for preserving process stability. Separating acidification activities (acetogenesis and hydrolysis) from methanation processes (acetogenesis and methanogenesis) is a key component of the two-stage AD process. After the first stage of anaerobic digestion is completed, it is feasible to separate the substrates into solid and liquid portions i.e. digestate and hydrolysate respectively. Extra conditioning or drying steps are no longer necessary since the digestate, consisting of an unmodified organic material, is subjected to hydrothermal carbonization to generate hydrochar and carbon-rich process water.98 To produce biogas, the second stage of anaerobic digestion may include the hydrolysate and aqueous liquid from HTC, which are high in soluble carbon and organic acids.92 The two-step approach improves overall process stability by offering the best conditions for bacterial growth at each stage. Additionally, it makes it possible to operate at larger rates of organic loading, which raises the amount of biogas produced per reactor volume.91 This integrated strategy guarantees the effective use of various substrates and improves the AD system's overall performance (Fig. 5(b)).
4.3.4. Gasification–syngas fermentation.
In comparison to other techniques, hybrid gasification–SNF (syngas fermentation) systems are more robust as shown in Fig. 6. The more stable hybrid gasification–SNF processes result from SNF's softer operating conditions. SNF biocatalysts have several benefits, including resistance to sulfur-based contaminants in syngas, lack of need for a certain CO/H molar ratio, and good selectivity for the intended end product with minimal byproducts.99 The optimal option for turning softwood and wheat straw into second-generation bioethanol was determined by integrating three different gasification technologies (bubbling fluidized bed, updraft fixed bed and indirect gasification) with SNF. According to the findings, the gasification technology selection affected the composition of the syngas. Wheat straw's lower tar concentration and H2 molar ratio made indirect gasification the most successful method.100 Carbon fixation was enhanced by 55% when Clostridium was utilized as an inoculum after downstream processing was employed to remove methane (CH4) and saturated C2–C4 hydrocarbons from syngas.101 It is recommended to use hydrochar as a porous material in SNF to improve mass transfer rates by immobilizing germs. Because hydrochar is high in macronutrients, it is also suggested that it be used as a sustainable fertilizer and soil amendment, supporting a circular economy.7
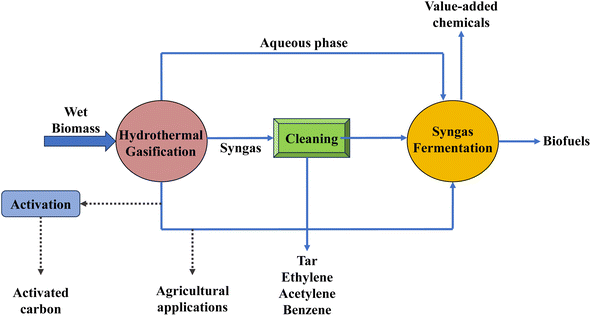 |
| Fig. 6 Flow chart illustrating the process of hybrid gasification and syngas fermentation. | |
4.3.5. Hydrothermal liquefaction–fermentation.
Hydrothermal liquefaction is a potential thermochemical conversion method that can produce biocrude oils from biomass with high moisture content. One of the characteristics of this process is the production of a significant volume of aqueous phase, or HTL-AL. Along with some number of organics, HTL-AL also contains C and N, which are transported via a variety of chemical routes.102 HTL-AL is commonly considered a waste liquid; however, it has the potential to be utilized as an energy source due to its high concentration of both organic and inorganic components, presence of heavy metal ions, and changeable chemical composition. Anaerobic fermentation is one biological conversion technology that could be utilized to value-add HTL-AL and create environmentally friendly chemicals and fuels. However, the liquid may contain potential inhibitors which might inhibit the biological process. Therefore, pretreatment methods including extraction, partial oxidation, and adsorption are employed to change the inhibitors into substances that the body can easily metabolize.102,103 Because it is more intensive and takes less time, aerobic fermentation is a practical biological conversion technique that can help HTL-AL develop value-added products when oxygen is present.104
5. Potential of bioenergy
The need for clean and renewable energy sources is becoming more pressing due to the growing demand for resources and energy, as well as worries about climate change and the finite supply of fossil fuels.105 A variety of energy sources are included in the category of renewable energy, such as solar, geothermal, hydropower, tidal, wind, and bioenergy. Among them, biomass is thought to be the best carbon source for creating fuels, chemicals, and renewable heat and power without harming the environment.106 Instead of drawing from subterranean carbon reserves, biofuels made from biomass provide a unique benefit by harnessing atmospheric carbon. A special role in improving the economy is played by biofuels, which have the potential to be sustainable and completely renewable.37 Since they are produced using a variety of renewable carbon sources and have the potential to be inexpensive, they are also very important in the renewable energy industry.107 In the next 30 years, it is anticipated that the production of biofuel from biomass will rise regardless of changes in the price of fossil fuels.108
6. Techno-economic and environmental assessment
Growing costs associated with energy sources and inappropriate disposal of waste materials are increasing public interest in improving environmental quality in the modern period. The economics of biomass conversion pathways are significantly influenced by the end products. Latent release of hazardous gases and substances like SOx, CO, and NOx as well as unstable chemical compounds generated from combustion and insufficient oxidation are the key hazards.109 All these dangerous gases are bad for the environment and human health. Therefore, seepage should be avoided during waste-to-energy conversion processes; a suitable conditioning system and an efficient gas clean-up are essential for this. Reducing the likelihood of incomplete oxidation during the conversion process is another effective strategy to lessen the risk of hazardous gases.
For the generation of ethanol, a hybrid method of gasification coupled with fermentation has been compared to traditional enzymatic hydrolysis coupled with fermentation technology. The cost of producing ethanol through gasification and fermentation is significantly higher than that of producing ethanol through enzymatic hydrolysis and fermentation.110 The primary reasons for this increased cost are the comparatively low ethanol yield, the high energy recovery costs, and the high capital cost. The use of land, the demand for equipment, and the amount of feed required all affect cost analysis. The labour and maintenance costs are included in the process's operational fee. These costs are all estimates, but the precise cost depends on the area's administrative requirements, incentives, feedstock accessibility, and skilled labour. However, gasification is not a good method for feedstocks with high moisture concentrations because it increases the cost of the entire process and needs the adoption of a pretreatment technology to boost processing efficiency.111 But when it comes to reducing incineration-related process costs, techniques like pyrolysis or gasification are more beneficial. Among all the other thermal and biochemical processes, pyrolysis, as well as hybrid conversion techniques, are the most appealing methods from an economic evaluation standpoint.
7. Life cycle assessment
It is crucial to evaluate the environmental and economic impacts of combining different processes to make informed decisions regarding the commercial feasibility of thermochemical and biological conversion methods.112 Using computer-based LCA is a valuable approach for comparing the environmental impacts of different waste-to-energy options.113 In many cases, an LCA can tackle various challenges associated with biomass conversion processes. These challenges can include economic considerations, limited resources, concerns about food security, health and safety issues, water usage, land use concerns, and potential environmental impacts on nearby communities.114 Using LCA, it is possible to determine the environmental impacts of different biomass and municipal solid waste conversion methods, including acidification and global warming, throughout the entire life cycle.115 To determine the most environmentally friendly or superior approach in terms of LCA, it is necessary to conduct a comparative LCA study for each specific case. This study should consider factors such as the specific feedstock, process parameters, and intended products.114 A comprehensive analysis should consider a wide range of environmental factors, such as energy consumption, carbon emissions, water usage, waste production, pollutant emissions, and resource utilization efficiency. It is recommended to conduct a comprehensive and site-specific LCA for each WtE process and its intended products. This analysis should consider the specific conditions and environmental priorities of the site. This analysis will provide a strong basis for evaluating environmental performance and making informed decisions regarding the most effective waste and biomass treatment methods.116
A comprehensive analysis was conducted using the LCA technique to compare a novel integrated HTC-AD technology for FW management with a reference AD process, both designed to be energetically self-sufficient.115 By utilizing hydrochar as a biofuel, the combined process presents an opportunity to enhance energy recovery from FW. The improved energy and resource recovery of the innovative FW management system leads to positive environmental effects and a better environmental life cycle compared to the reference AD system. In addition, the combination of AD and pyrolysis has the potential to enhance productivity, sustainability, and the exploration of innovative applications for byproducts in the future.116 One potential solution for managing SSW in a cost-effective and eco-friendly manner could involve combining AD with plasma pyrolysis.116 Therefore, it is crucial to utilize LCA analysis to evaluate the environmental performance and sustainability of different WtE processes. These studies provide valuable insights into the environmental impacts and help identify the most eco-friendly methods for utilizing biomass and other waste materials. Existing research on LCA evaluating biomass pyrolysis mainly emphasizes the assessment of GWP, while giving less consideration to other environmental impact categories. Therefore, it is important to prioritize a thorough environmental assessment study to fully evaluate the environmental impact of the biomass conversion system.
The evaluation of waste utilization has established a reputation for equating most of the criteria within the various treatment options and end-product creation. It has also produced the main decision support tool for policymakers at all levels to make the best decisions about waste management.
8. Environmental application of advanced biomass conversion techniques
The industrial sector faces numerous obstacles because of the shortcomings of current technologies, which are gradually being replaced by more sophisticated developing technologies. The most common conversion process, pyrolysis, produces large amounts of heat energy. However, with the application of microwave stimulation and additional chemicals, heat energy is now changed to produce a high yield rate.50 The result is mesoporous biochar, which has a unique high surface area. A new technology called solar energy-assisted pyrolysis is used in place of the traditional method. With this technique, the system is exposed to a variety of solar heat temperatures. This process yields a phenol content of roughly 44% while maintaining the hydrocarbon's properties, and it is utilized to produce a variety of biofuels.117
After undergoing various treatments, biomass derived from the lignocellulose components yields a variety of high-value products, including bio-oil, biogas, and other energy sources. After being treated, feed biomass from forests, agriculture, and food waste is produced as pellets and charcoal that are enhanced with hydrocarbon components.118 They generate products for bioremediation, wastewater treatment, and pollution control at elevated production rates of up to 134%. Because biochar has a large pore opening and can adsorb dye particles, it is used to remove environmental colours from water. It works well to remove dyes connected to leather and textiles since it can be carbonated or decarbonated.119 The bioconversion of waste into useful goods has led to the development of a new and innovative approach. This conversion is now carried out with the assistance of a high-range designed protein-infused membrane. Today, pectinolytic enzymes have been found to significantly increase lignin breakdown by 58.4% compared to the chemical enzymes currently in use. Metagenomic research was utilized to investigate this phenomenon.120 Pre-treatment is a common technique for producing bioethanol and other liquid fuels because it improves pore size, breaks down biomass, and makes cellulases available for conversion.
9. Future perspectives
To maximize resource recovery, this study investigates different thermochemical and biochemical conversion process combinations. The techno-economic aspects of hybrid thermochemical–biochemical conversion processes, which include pyrolysis–anaerobic digestion, hydrothermal liquefaction–anaerobic digestion, gasification–syngas fermentation, and hydrothermal carbonization–anaerobic digestion, have been thoroughly examined.121 More research is necessary to fully comprehend residue recycling and efficient utilization, even if the hybrid pyrolysis–AD method exhibits promise from an economic and environmental standpoint. It is crucial to investigate and compare the pyro-liquid's composition with that of aqueous liquids resulting from various thermochemical conversion processes. It takes interdisciplinary understanding to combine thermochemical and biochemical processes for the valorization of waste biomass. Research on the chemical composition of waste biomass and behaviour under different conversion processes is necessary for optimization. It is necessary to thoroughly investigate the behaviour of microbes in a variety of aerobic and anaerobic environments as well as the conversion pathways. Although most research on gasification concentrates on conventional gasification and ignores hydrothermal gasification, hybrid gasification–SNF is a viable integrated method. A detailed investigation of the environmental consequences and economic viability of coupling hydrothermal gasification with SNF is necessary.122 Given that the process of hybrid gasification–AD is the least known, experimental methods and simulation studies must be included in future research to achieve complete knowledge and optimization. For global adoption, decision-making and forecasting of the number of biofuels generated must take feedstock supply into account. Chemical process modelling and optimization have been tackled through the application of ML, which offers precise forecasting and financial benefits. Through integrated processes, ML technology might monitor and control the production of green chemicals and biofuels. Future research must focus on machine learning models for process prediction, control, optimization, and real-time monitoring, as evidenced by the dearth of ML studies regarding integrated processes. It is difficult and complex to model integrated systems using phenomenological and mathematical models. Because of their resilience to the extreme complexity, heterogeneity, and dynamic character of integrated processes, machine learning models are highly valued. Researchers have conducted TEA on the combination of thermochemical and biochemical conversion processes; however, an integrated TEA that takes into account different production routes and products is still necessary.123 A thorough analysis of the effects on the economy and environment of alternative manufacturing routes for different products is also necessary. To enhance the yield of biofuels, additional investigation is necessary in the domains of feedstock pretreatment, catalyst creation, life-cycle evaluation, and overall production system enhancement.
10. Conclusion
Bioenergy is a type of fuel that is derived from a range of biomass sources, including manures, sewage sludge, forestry residues, agricultural residues, and solid organic municipal wastes. It is both economically viable and environmentally sustainable. The promise of biofuels, characterized by their low carbon emissions and ample availability of raw materials, is widely recognized as a key component for achieving a sustainable future. This study's efforts have primarily concentrated on biofuel production, specifically addressing difficulties such as feedstock pretreatment, catalyst development, life-cycle assessment, and overall system optimization. The waste-to-energy industry, which supports a circular economy, has advanced with methods that can convert different types of garbage into energy and useful products via thermochemical and biochemical processes. While these technologies help reduce the need for non-renewable resources, they also have their drawbacks. Thermochemical conversion generally yields biofuel of inferior quality and incurs substantial production expenses. In contrast, biochemical conversion is less efficient in breaking down resilient biomass materials and has a slower production rate. Integrating several treatment methods not only promotes the development of a circular economy concept but also mitigates the limitations of a single conversion pathway by leveraging the benefits of both approaches. The integration of many technologies not only mitigates the difficulties associated with each technology but also reduces the total cost of the process and enhances the energy sustainability of the entire process. This study recognizes the highly advanced waste-to-energy industry and its significant role in promoting sustainability, managing waste, and facilitating the transition to a circular economy. Nevertheless, hybrid systems necessitate additional investigation to comprehensively comprehend their benefits and limitations.
Abbreviations
AD | Anaerobic digestion |
AW | Agricultural waste |
COD | Chemical oxygen demand |
FAO | Food and agricultural organization |
FW | Food waste |
GWP | Global warming potential |
HTC | Hydrothermal carbonization |
HTL | Hydrothermal liquefaction |
HTL-AL | Hydrothermal liquefaction-aqueous liquid |
LCA | Life cycle assessment |
ML | Machine learning |
SF | Syngas fermentation |
TEA | Techno-economic assessment |
WtE | Waste-to-energy |
WW | Wood waste |
W% | Weight% |
Author contributions
Sheetal Kumari: conceptualization, methodology, investigation, writing – original draft; Yasmin Ara Begum: conceptualization, writing – original draft, and visualization; Shailendra K Jain: writing – review & editing, conceptualization; Manoj Chandra Garg: conceptualization, writing – review & editing, supervision, and funding acquisition.
Conflicts of interest
The authors declare that they have no known competing financial interests or personal relationships that could have appeared to influence the work reported in this work.
Acknowledgements
Authors thank the Amity Institute of Environmental Sciences, Amity University Uttar Pradesh, Noida, India for valuable support.
References
- D. Haldar and M. K. Purkait, A review on the environment-friendly emerging techniques for pretreatment of lignocellulosic biomass: Mechanistic insight and advancements, Chemosphere, 2021, 264, 128523 CrossRef CAS PubMed.
- J. Fang, L. Zhan, Y. S. Ok and B. Gao, Minireview of potential applications of hydrochar derived from hydrothermal carbonization of biomass, J. Ind. Eng. Chem., 2018, 57, 15–21 CrossRef CAS.
- B. Bilska, M. Tomaszewska, D. Kołożyn-Krajewska, K. Szczepański, R. Łaba and S. Łaba, Environmental aspects of food wastage in trade – a case study, Environ. Prot. Nat. Resour., 2020, 31(2), 24–34 Search PubMed.
- C. Maraveas, Production of Sustainable and Biodegradable Polymers from Agricultural Waste, Polymers, 2020, 12(5), 1127 CrossRef CAS PubMed.
-
FAO, Food Agricultural Commodities Production, Food and Agriculture Organisation of the United Nations, 2017 Search PubMed.
- S. Jha, J. A. Okolie, S. Nanda and A. K. Dalai, A Review of Biomass Resources and Thermochemical Conversion Technologies, Chem. Eng. Technol., 2022, 45(5), 791–799 CrossRef CAS.
- C. D. Pinales-Márquez, R. M. Rodríguez-Jasso, R. G. Araújo, A. Loredo-Treviño, D. Nabarlatz and B. Gullón,
et al., Circular bioeconomy and integrated biorefinery in the production of xylooligosaccharides from lignocellulosic biomass: A review, Ind. Crops Prod., 2021, 162, 113274 CrossRef.
- S. V. Godvin, V. Kumar Tyagi, S. Varjani and J. Rajesh Banu, A review on the lignocellulosic derived biochar-based catalyst in wastewater remediation: Advanced treatment technologies and machine learning tools, Bioresour. Technol., 2023, 387, 129587 CrossRef PubMed.
- J. A. Okolie, S. Nanda, A. K. Dalai, F. Berruti and J. A. Kozinski, A review on subcritical and supercritical water gasification of biogenic, polymeric and petroleum wastes to hydrogen-rich synthesis gas, Renew. Sustain. Energy Rev., 2020, 119, 109546 CrossRef CAS.
- W. Xiang, X. Zhang, J. Chen, W. Zou, F. He and X. Hu,
et al., Biochar technology in wastewater treatment: A critical review, Chemosphere, 2020, 252, 126539 CrossRef CAS PubMed.
- A. Colantoni, N. Evic, R. Lord, S. Retschitzegger, A. R. Proto and F. Gallucci,
et al., Characterization of biochars produced from pyrolysis of pelletized agricultural residues, Renew. Sustain. Energy Rev., 2016, 64, 187–194 CrossRef CAS.
- X. Xiong, I. K. M. Yu, D. C. W. Tsang, N. S. Bolan, Y. Sik Ok and A. D. Igalavithana,
et al., Value-added chemicals from food supply chain wastes: State-of-the-art review and future prospects, Chem. Eng. J., 2019, 375, 121983 CrossRef CAS.
- D. Mohan, A. Sarswat, Y. S. Ok and C. U. Pittman, Organic and inorganic contaminants removal from water with biochar, a renewable, low cost and sustainable adsorbent – A critical review, Bioresour. Technol., 2014, 160, 191–202 CrossRef CAS PubMed.
- M. E. González, M. Cea, D. Reyes, L. Romero-Hermoso, P. Hidalgo and S. Meier,
et al., Functionalization of biochar derived from lignocellulosic biomass using microwave technology for catalytic application in biodiesel production, Energy Convers. Manag., 2017, 137, 165–173 CrossRef.
- J. S. Cha, S. H. Park, S. C. Jung, C. Ryu, J. K. Jeon and M. C. Shin,
et al., Production and utilization of biochar: A review, J. Ind. Eng. Chem., 2016, 40, 1–15 CrossRef CAS.
- B. Wang, B. Gao and J. Fang, Recent advances in engineered biochar productions and applications, Crit. Rev. Environ. Sci. Technol., 2017, 47(22), 2158–2207 CrossRef CAS.
- A. Saravanan, S. Karishma, P. Senthil Kumar and G. Rangasamy, A review on regeneration of biowaste into bio-products and bioenergy: Life cycle assessment and circular economy, Fuel, 2023, 338, 127221 CrossRef CAS.
- S. Manikandan, S. Vickram, R. Sirohi, R. Subbaiya, R. Y. Krishnan and N. Karmegam,
et al., Critical review of biochemical pathways to transformation of waste and biomass into bioenergy, Bioresour. Technol., 2023, 372, 128679 CrossRef CAS PubMed.
- M. A. Helal, N. Anderson, Y. Wei and M. Thompson, A Review of Biomass-to-Bioenergy Supply Chain Research Using Bibliometric Analysis and Visualization, Energies, 2023, 16(3), 1187 CrossRef.
- P. R. Yaashikaa, P. S. Kumar, S. Varjani and A. Saravanan, A critical review on the biochar production techniques, characterization, stability and applications for circular bioeconomy, Biotechnol. Rep., 2020, 28, e00570 CrossRef CAS PubMed.
- Z. Chen, L. Chen, K. S. Khoo, V. K. Gupta, M. Sharma and P. L. Show,
et al., Exploitation of lignocellulosic-based biomass biorefinery: A critical review of renewable bioresource, sustainability and economic views, Biotechnol. Adv., 2023, 69, 108265 CrossRef CAS PubMed.
- H. Chen, H. Xu, H. Zhu, S. Yan, S. Zhang and H. Zhang,
et al., A review on bioslurry fuels derived from bio-oil and biochar: Preparation, fuel properties and application, Fuel, 2024, 355, 129283 CrossRef CAS.
- M. Ugolini, L. Recchia, H. E. Wray, J. W. Dijkstra and P. Nanou, Environmental Assessment of Hydrothermal Treatment of Wet Bio-Residues from Forest-Based and Agro-Industries into Intermediate Bioenergy Carriers, Energies, 2024, 17(3), 560 CrossRef CAS.
- S. A. Raj, S. Kumar Singh and S. Jain, A review on bioenergy and biofuel production, Mater. Today Proc., 2022, 49, 510–516 CrossRef.
- A. I. Osman, Z. Y. Lai, M. Farghali, C. L. Yiin, A. M. Elgarahy and A. Hammad,
et al., Optimizing biomass pathways to bioenergy and biochar application in electricity generation, biodiesel production, and biohydrogen production, Environ. Chem. Lett., 2023, 21(5), 2639–2705 CrossRef CAS.
- A. Saravanan, P. Senthil Kumar, S. Jeevanantham, S. Karishma and D. V. N. Vo, Recent advances and sustainable development of biofuels production from lignocellulosic biomass, Bioresour. Technol., 2022, 344, 126203 CrossRef CAS PubMed.
- G. M. Mathew, D. Raina, V. Narisetty, V. Kumar, S. Saran and A. Pugazhendi,
et al., Recent advances in biodiesel production: Challenges and solutions, Sci. Total Environ., 2021, 794, 148751 CrossRef CAS PubMed.
- A. Mukhtar, S. Saqib, M. Mubashir, S. Ullah, A. Inayat and A. Mahmood,
et al., Mitigation of CO2 emissions by transforming to biofuels: Optimization of biofuels production processes, Renew. Sustain. Energy Rev., 2021, 150, 111487 CrossRef CAS.
- R. Garg, R. Sabouni and M. Ahmadipour, From waste to fuel: Challenging aspects in sustainable biodiesel production from lignocellulosic biomass feedstocks and role of metal organic framework as innovative heterogeneous catalysts, Ind. Crops Prod., 2023, 206, 117554 CrossRef CAS.
- P. Swaminaathan, A. Saravanan and P. Thamarai, Utilization of bioresources for high-value bioproducts production: Sustainability and perspectives in circular bioeconomy, Sustain. Energy Technol. Assessments, 2024, 63, 103672 CrossRef.
- S. Periyasamy, A. Asefa Adego, P. S. Kumar, G. G. Desta, T. Zelalem and V. Karthik,
et al., Influencing factors and environmental feasibility analysis of agricultural waste preprocessing routes towards biofuel production – A review, Biomass Bioenergy, 2024, 180, 107001 CrossRef CAS.
- K. N. Palansooriya, Y. Yang, Y. F. Tsang, B. Sarkar, D. Hou and X. Cao,
et al., Occurrence of contaminants in drinking water sources and the potential of biochar for water quality improvement: A review, Crit. Rev. Environ. Sci. Technol., 2020, 50(6), 549–611 CrossRef CAS.
- J. C. Yoo, J. Beiyuan, L. Wang, D. C. W. Tsang, K. Baek and N. S. Bolan,
et al., A combination of ferric nitrate/EDDS-enhanced washing and sludge-derived biochar stabilization of metal-contaminated soils, Sci. Total Environ., 2018, 616–617, 572–582 CrossRef CAS PubMed.
- L. Cao, I. K. M. Yu, D. W. Cho, D. Wang, D. C. W. Tsang and S. Zhang,
et al., Microwave-assisted low-temperature hydrothermal treatment of red seaweed (Gracilaria lemaneiformis) for production of levulinic acid and algae hydrochar, Bioresour. Technol., 2019, 273, 251–258 CrossRef CAS PubMed.
- X. Xiong, I. K. M. Yu, L. Cao, D. C. W. Tsang, S. Zhang and Y. S. Ok, A review of biochar-based catalysts for chemical synthesis, biofuel production, and pollution control, Bioresour. Technol., 2017, 246, 254–270 CrossRef CAS PubMed.
-
W. Elbersen, T. M. Lammens, E. A. Alakangas, B. Annevelink, P. Harmsen and B. Elbersen. Lignocellulosic Biomass Quality, in Modeling and Optimization of Biomass Supply Chains, Elsevier, 2017, pp. 55–78 Search PubMed.
- R. Shinde, D. K. Shahi, P. Mahapatra, C. S. Singh, S. K. Naik and N. Thombare,
et al., Management of crop residues with special reference to the on-farm utilization methods: A review, Ind. Crops Prod., 2022, 181, 114772 CrossRef.
-
S. Arora, L. K. Sarao and A. Singh, Bioenergy from Cellulose of Woody Biomass, in Agroindustrial Waste for Green Fuel Application, 2023, pp. 89–120 Search PubMed.
- J. Wang and S. Wang, Preparation, modification and environmental application of biochar: A review, J. Clean. Prod., 2019, 227, 1002–1022 CrossRef CAS.
- A. Tomczyk, Z. Sokołowska and P. Boguta, Biochar physicochemical properties: pyrolysis temperature and feedstock kind effects, Rev. Environ. Sci. Biotechnol., 2020, 19(1), 191–215 CrossRef CAS.
- J. Wang and S. Wang, Preparation, modification and environmental application of biochar: A review, J. Clean. Prod., 2019, 227, 1002–1022 CrossRef CAS.
- S. X. Zhao, N. Ta and X. D. Wang, Effect of Temperature on the Structural and Physicochemical Properties of Biochar with Apple Tree Branches as Feedstock Material, Energies, 2017, 10(9), 1293 CrossRef.
-
C. L. Williams, R. M. Emerson and J. S. Tumuluru, Biomass Compositional Analysis for Conversion to Renewable Fuels and Chemicals, in Biomass Volume Estimation and Valorization for Energy, InTech, 2017 Search PubMed.
- A. Saravanakumar, P. Vijayakumar, A. T. Hoang, E. E. Kwon and W. H. Chen, Thermochemical conversion of large-size woody biomass for carbon neutrality: Principles, applications, and issues, Bioresour. Technol., 2023, 370, 128562 CrossRef CAS PubMed.
- C. Liu, H. Wang, A. M. Karim, J. Sun and Y. Wang, Catalytic fast pyrolysis of lignocellulosic biomass, Chem. Soc. Rev., 2014, 43(22), 7594–7623 RSC.
- X. Zhang, Essential scientific mapping of the value chain of thermochemically converted second-generation bio-fuels, Green Chem., 2016, 18(19), 5086–5117 RSC.
-
J. Zhang and X. Zhang, The thermochemical conversion of biomass into biofuels, in Biomass, Biopolymer-Based Materials, and Bioenergy, Elsevier, 2019, pp. 327–368 Search PubMed.
- M. K. Awasthi, T. Sar, S. C. Gowd, K. Rajendran, V. Kumar and S. Sarsaiya,
et al., A comprehensive review on thermochemical, and biochemical conversion methods of lignocellulosic biomass into valuable end product, Fuel, 2023, 342, 127790 CrossRef CAS.
- S. A. A. Taqvi, B. Kazmi, S. R. Naqvi, D. Juchelková and A. Bokhari, State-of-the-Art Review of Biomass Gasification: Raw to Energy Generation, ChemBioEng Rev., 2024 DOI:10.1002/cben.202400003.
- K. Wang and J. W. Tester, Sustainable management of unavoidable biomass wastes, Green Energy Resour., 2023, 1(1), 100005 CrossRef.
- S. G. Nkuna, T. O. Olwal, S. D. Chowdhury and J. M. Ndambuki, A review of wastewater sludge-to-energy generation focused on thermochemical technologies: An improved technological, economical and socio-environmental aspect, Cleaner Waste Systems, 2024, 7, 100130 CrossRef.
- P. R. Yaashikaa, K. P. Senthil, S. J. Varjani and A. Saravanan, Advances in production and application of biochar from lignocellulosic feedstocks for remediation of environmental pollutants, Bioresour. Technol., 2019, 292, 122030 CrossRef CAS PubMed.
- N. Banerjee, Biomass to Energy—an Analysis of Current Technologies, Prospects, and Challenges, BioEnergy Res., 2023, 16(2), 683–716 CrossRef.
-
S. Aishwarya, G. Sruthi, M. N. Aditya, K. Sivagami and S. Chakraborty, Biomass Energy Conversion Using Thermochemical and Biochemical Technologies, in Sustainable and Clean Energy Production Technologies Clean Energy Production Technologies, 2022, pp. 93–131 Search PubMed.
- S. Manikandan, S. Vickram, R. Sirohi, R. Subbaiya, R. Y. Krishnan and N. Karmegam,
et al., Critical review of biochemical pathways to transformation of waste and biomass into bioenergy, Bioresour. Technol., 2023, 372, 128679 CrossRef CAS PubMed.
- J. Ahmad, F. Patuzzi, U. Rashid, M. Shahabz, C. Ngamcharussrivichai and M. Baratieri, Exploring untapped effect of process conditions on biochar characteristics and applications, Environ. Technol. Innovat., 2021, 21, 101310 CrossRef CAS.
- D. Pandey, A. Daverey and K. Arunachalam, Biochar: Production, properties and emerging role as a support for enzyme immobilization, J. Clean. Prod., 2020, 255, 120267 CrossRef CAS.
- A. V. Bridgwater, The production of biofuels and renewable chemicals by fast pyrolysis of biomass, Int. J. Global Energy Issues, 2007, 27(2), 160 CrossRef.
- W. J. DeSisto, N. Hill, S. H. Beis, S. Mukkamala, J. Joseph and C. Baker,
et al., Fast Pyrolysis of Pine Sawdust in a Fluidized-Bed Reactor, Energy Fuels, 2010, 24(4), 2642–2651 CrossRef CAS.
- B. Singh, Z. Szamosi and Z. Siménfalvi, Impact of mixing intensity and duration on biogas production in an anaerobic digester: a review, Crit. Rev. Biotechnol., 2020, 40(4), 508–521 CrossRef CAS PubMed.
- A. Cesaro, The valorization of the anaerobic digestate from the organic fractions of municipal solid waste: Challenges and perspectives, J. Environ. Manage., 2021, 280, 111742 CrossRef CAS PubMed.
- B. Gunes, J. Stokes, P. Davis, C. Connolly and J. Lawler, Optimisation of anaerobic digestion of pot ale after thermochemical pre-treatment through Response Surface Methodology, Biomass Bioenergy, 2021, 144, 105902 CrossRef CAS.
- B. Gunes, J. Stokes, P. Davis, C. Connolly and J. Lawler, Pre-treatments to enhance biogas yield and quality from anaerobic digestion of whiskey distillery and brewery wastes: A review, Renew. Sustain. Energy Rev., 2019, 113, 109281 CrossRef CAS.
- F. Monlau, C. Sambusiti, N. Antoniou, A. Barakat and A. Zabaniotou, A new concept for enhancing energy recovery from agricultural residues by coupling anaerobic digestion and pyrolysis process, Appl. Energy, 2015, 148, 32–38 CrossRef.
- J. Liang, Y. Lin, S. Wu, C. Liu, M. Lei and C. Zeng, Enhancing the quality of bio-oil and selectivity of phenols compounds from pyrolysis of anaerobic digested rice straw, Bioresour. Technol., 2015, 181, 220–223 CrossRef CAS PubMed.
- F. Monlau, M. Francavilla, C. Sambusiti, N. Antoniou, A. Solhy and A. Libutti,
et al., Toward a functional integration of anaerobic digestion and pyrolysis for a sustainable resource management. Comparison between solid-digestate and its derived pyrochar as soil amendment, Appl. Energy, 2016, 169, 652–662 CrossRef CAS.
- S. Xiu, A. Shahbazi and R. Li, Characterization, Modification and Application of Biochar for Energy Storage and Catalysis: A Review, Trends Renew. Energy, 2017, 3(1), 86–101 CrossRef.
- Z. Yang, Y. Liu, J. Zhang, K. Mao, M. Kurbonova and G. Liu,
et al., Improvement of biofuel recovery from food waste by integration of anaerobic digestion, digestate pyrolysis and syngas biomethanation under mesophilic and thermophilic conditions, J. Clean. Prod., 2020, 256, 120594 CrossRef CAS.
- S. Tayibi, F. Monlau, F. Marias, G. Cazaudehore, N. E. Fayoud and A. Oukarroum,
et al., Coupling anaerobic digestion and pyrolysis processes for maximizing energy recovery and soil preservation according to the circular economy concept, J. Environ. Manage., 2021, 279, 111632 CrossRef CAS PubMed.
- J. A. Okolie, E. I. Epelle, M. E. Tabat, U. Orivri, A. N. Amenaghawon and P. U. Okoye,
et al., Waste biomass valorization for the production of biofuels and value-added products: A comprehensive review of thermochemical, biological and integrated processes, Process Saf. Environ. Prot., 2022, 159, 323–344 CrossRef CAS.
- Q. Li, M. Xu, G. Wang, R. Chen, W. Qiao and X. Wang, Biochar assisted thermophilic co-digestion of food waste and waste activated sludge under high feedstock to seed sludge ratio in batch experiment, Bioresour. Technol., 2018, 249, 1009–1016 CrossRef CAS PubMed.
- S. Sahota, V. K. Vijay, P. M. V. Subbarao, R. Chandra, P. Ghosh and G. Shah,
et al., Characterization of leaf waste based biochar for cost effective hydrogen sulphide removal from biogas, Bioresour. Technol., 2018, 250, 635–641 CrossRef CAS PubMed.
- G. Wang, Q. Li, X. Gao and X. C. Wang, Synergetic promotion of syntrophic methane production from anaerobic digestion of complex organic wastes by biochar: Performance and associated mechanisms, Bioresour. Technol., 2018, 250, 812–820 CrossRef CAS PubMed.
- S. H. Ho, Y. d. Chen, Z. k. Yang, D. Nagarajan, J. S. Chang and N. q. Ren, High-efficiency removal of lead from wastewater by biochar derived from anaerobic digestion sludge, Bioresour. Technol., 2017, 246, 142–149 CrossRef CAS PubMed.
- D. Wang, J. Ai, F. Shen, G. Yang, Y. Zhang and S. Deng,
et al., Improving anaerobic digestion of easy-acidification substrates by promoting buffering capacity using biochar derived from vermicompost, Bioresour. Technol., 2017, 227, 286–296 CrossRef CAS PubMed.
- M. O. Fagbohungbe, B. M. J. Herbert, L. Hurst, H. Li, S. Q. Usmani and K. T. Semple, Impact of biochar on the anaerobic digestion of citrus peel waste, Bioresour. Technol., 2016, 216, 142–149 CrossRef CAS PubMed.
- N. Kassem, J. Hockey, C. Lopez, L. Lardon, L. T. Angenent and J. W. Tester, Integrating anaerobic digestion, hydrothermal liquefaction, and biomethanation with in a power-to-gas framework for dairy waste management and grid decarbonization: a techno-economic assessment, Sustain. Energy Fuels, 2020, 4(9), 4644–4661 RSC.
- M. Shahabuddin, E. Italiani, A. R. Teixeira, N. Kazantzis and M. T. Timko, Roadmap for Deployment of Modularized Hydrothermal Liquefaction: Understanding the Impacts of Industry Learning, Optimal Plant Scale, and Delivery Costs on Biofuel Pricing, ACS Sustain. Chem. Eng., 2023, 11(2), 733–743 CrossRef CAS.
- N. Khan, K. Sudhakar and R. Mamat, Thermogravimetric Analysis of Marine Macroalgae Waste Biomass as Bio-Renewable Fuel, J. Chem., 2022, 2022, 1–9 Search PubMed.
- L. Yang, B. Si, X. Tan, H. Chu, X. Zhou and Y. Zhang,
et al., Integrated anaerobic digestion and algae cultivation for energy recovery and nutrient supply from post-hydrothermal liquefaction wastewater, Bioresour. Technol., 2018, 266, 349–356 CrossRef CAS PubMed.
- F. Xu, Y. Li, X. Ge, L. Yang and Y. Li, Anaerobic digestion of food waste – Challenges and opportunities, Bioresour. Technol., 2018, 247, 1047–1058 CrossRef CAS PubMed.
- J. Xu, A. M. Mustafa, H. Lin, U. Y. Choe and K. Sheng, Effect of hydrochar on anaerobic digestion of dead pig carcass after hydrothermal pretreatment, Waste Manage., 2018, 78, 849–856 CrossRef CAS PubMed.
- R. Li, D. Liu, Y. Zhang, J. Zhou, Y. F. Tsang and Z. Liu,
et al., Improved methane production and energy recovery of post-hydrothermal liquefaction waste water via integration of zeolite adsorption and anaerobic digestion, Sci. Total Environ., 2019, 651, 61–69 CrossRef CAS PubMed.
- H. Chen, Y. Rao, L. Cao, Y. Shi, S. Hao and G. Luo,
et al., Hydrothermal conversion of sewage sludge: Focusing on the characterization of liquid products and their methane yields, Chem. Eng. J., 2019, 357, 367–375 CrossRef CAS.
- S. Fernandez, K. Srinivas, A. J. Schmidt, M. S. Swita and B. K. Ahring, Anaerobic digestion of organic fraction from hydrothermal liquefied algae wastewater byproduct, Bioresour. Technol., 2018, 247, 250–258 CrossRef CAS PubMed.
- B. Si, J. Li, Z. Zhu, M. Shen, J. Lu and N. Duan,
et al., Inhibitors degradation and microbial response during continuous anaerobic conversion of hydrothermal liquefaction wastewater, Sci. Total Environ., 2018, 630, 1124–1132 CrossRef CAS PubMed.
- K. R. Parmar and A. B. Ross, Integration of Hydrothermal Carbonisation with Anaerobic Digestion; Opportunities for Valorisation of Digestate, Energies, 2019, 12(9), 1586 CrossRef CAS.
- X. Lu, X. Ma, X. Chen, Z. Yao and C. Zhang, Co-hydrothermal carbonization of polyvinyl chloride and corncob for clean solid fuel production, Bioresour. Technol., 2020, 301, 122763 CrossRef CAS PubMed.
- M. Śliz and M. Wilk, A comprehensive investigation of hydrothermal carbonization: Energy potential of hydrochar derived from Virginia mallow, Renew. Energy, 2020, 156, 942–950 CrossRef.
- V. S. Sikarwar, M. Pohořelý, E. Meers, S. Skoblia, J. Moško and M. Jeremiáš, Potential of coupling anaerobic digestion with thermochemical technologies for waste valorization, Fuel, 2021, 294, 120533 CrossRef CAS.
- Z. Cao, B. Hülsemann, D. Wüst, L. Illi, H. Oechsner and A. Kruse, Valorization of maize silage digestate from two-stage anaerobic digestion by hydrothermal carbonization, Energy Convers. Manag., 2020, 222, 113218 CrossRef CAS.
- P. P. Ravi, J. Lindner, H. Oechsner and A. Lemmer, Effects of target pH-value on organic acids and methane production in two-stage anaerobic digestion of vegetable waste, Bioresour. Technol., 2018, 247, 96–102 CrossRef CAS PubMed.
- C. I. Aragón-Briceño, A. B. Ross and M. A. Camargo-Valero, Mass and energy integration study of hydrothermal carbonization with anaerobic digestion of sewage sludge, Renew. Energy, 2021, 167, 473–483 CrossRef.
- R. Z. Gaur, O. Khoury, M. Zohar, E. Poverenov, R. Darzi and Y. Laor,
et al., Hydrothermal carbonization of sewage sludge coupled with anaerobic digestion: Integrated approach for sludge management and energy recycling, Energy Convers. Manag., 2020, 224, 113353 CrossRef CAS.
- A. E. Brown, J. M. M. Adams, O. R. Grasham, M. A. Camargo-Valero and A. B. Ross, An Assessment of Different Integration Strategies of Hydrothermal Carbonisation and Anaerobic Digestion of Water Hyacinth, Energies, 2020, 13(22), 5983 CrossRef CAS.
- A. E. Brown, G. L. Finnerty, M. A. Camargo-Valero and A. B. Ross, Valorisation of macroalgae via the integration of hydrothermal carbonisation and anaerobic digestion, Bioresour. Technol., 2020, 312, 123539 CrossRef CAS PubMed.
- J. D. Marin-Batista, J. A. Villamil, S. V. Qaramaleki, C. J. Coronella, A. F. Mohedano and M. A. d. l. Rubia, Energy valorization of cow manure by hydrothermal carbonization and anaerobic digestion, Renew. Energy, 2020, 160, 623–632 CrossRef CAS.
- C. I. Aragón-Briceño, A. B. Ross and M. A. Camargo-Valero, Mass and energy integration study of hydrothermal carbonization with anaerobic digestion of sewage sludge, Renew. Energy, 2021, 167, 473–483 CrossRef.
- I. K. Stoll, N. Boukis and J. Sauer, Syngas Fermentation to Alcohols: Reactor Technology and Application Perspective, Chem. Ing. Tech., 2020, 92(1–2), 125–136 CrossRef CAS.
- E. T. Liakakou, B. J. Vreugdenhil, N. Cerone, F. Zimbardi, F. Pinto and R. André,
et al., Gasification of lignin-rich residues for the production of biofuels via syngas fermentation: Comparison of gasification technologies, Fuel, 2019, 251, 580–592 CrossRef CAS.
- E. T. Liakakou, A. Infantes, A. Neumann and B. J. Vreugdenhil, Connecting gasification with syngas fermentation: Comparison of the performance of lignin and beech wood, Fuel, 2021, 290, 120054 CrossRef CAS.
- J. Watson, T. Wang, B. Si, W. T. Chen, A. Aierzhati and Y. Zhang, Valorization of hydrothermal liquefaction aqueous phase: pathways towards commercial viability, Prog. Energy Combust. Sci., 2020, 77, 100819 CrossRef.
- D. Quispe-Arpasi, R. de Souza, M. Stablein, Z. Liu, N. Duan and H. Lu,
et al., Anaerobic and photocatalytic treatments of post-hydrothermal liquefaction wastewater using H2O2, Bioresour. Technol. Rep., 2018, 3, 247–255 CrossRef.
- Y. Gu, X. Zhang, B. Deal and L. Han, Biological systems for treatment and valorization of wastewater generated from hydrothermal liquefaction of biomass and systems thinking: A review, Bioresour. Technol., 2019, 278, 329–345 CrossRef CAS PubMed.
- D. A. Ruddy, J. A. Schaidle, J. R. Ferrell III, J. Wang, L. Moens and J. E. Hensley, Recent advances in heterogeneous catalysts for bio-oil upgrading via “ex situ catalytic fast pyrolysis”: catalyst development through the study of model compounds, Green Chem., 2014, 16(2), 454–490 RSC.
- S. R. Barman, P. Banerjee, P. Das and A. Mukhopadhayay, Urban wood waste as precursor of activated carbon and its subsequent application for adsorption of polyaromatic hydrocarbons, Int. J. Energy Water Resour., 2018, 2(1–4), 1–13 Search PubMed.
-
C. L. Williams, R. M. Emerson and J. S. Tumuluru, Biomass Compositional Analysis for Conversion to Renewable Fuels and Chemicals, in Biomass Volume Estimation and Valorization for Energy, InTech, 2017 Search PubMed.
- L. Dai, L. Fan, Y. Liu, R. Ruan, Y. Wang and Y. Zhou,
et al., Production of bio-oil and biochar from soapstock via microwave-assisted co-catalytic fast pyrolysis, Bioresour. Technol., 2017, 225, 1–8 CrossRef CAS PubMed.
- T. G. Ambaye, M. Vaccari, A. Bonilla-Petriciolet, S. Prasad, E. D. van Hullebusch and S. Rtimi, Emerging technologies for biofuel production: A critical review on recent progress, challenges and perspectives, J. Environ. Manage., 2021, 290, 112627 CrossRef CAS PubMed.
- S. Pati, S. De and R. Chowdhury, Integrated techno-economic, investment risk and life cycle analysis of Indian lignocellulosic biomass valorisation via co-gasification and syngas fermentation, J. Clean. Prod., 2023, 423, 138744 CrossRef CAS.
- Y. Wang, G. Li, Z. Liu, P. Cui, Z. Zhu and S. Yang, Techno-economic analysis of biomass-to-hydrogen process in comparison with coal-to-hydrogen process, Energy, 2019, 185, 1063–1075 CrossRef CAS.
- R. Naveenkumar, J. Iyyappan, R. Pravin, S. Kadry, J. Han and R. Sindhu,
et al., A strategic review on sustainable approaches in municipal solid waste management and energy recovery: Role of artificial intelligence, economic stability and life cycle assessment, Bioresour. Technol., 2023, 379, 129044 CrossRef CAS PubMed.
- H. Durak, Comprehensive Assessment of Thermochemical Processes for Sustainable Waste Management and Resource Recovery, Processes, 2023, 11(7), 2092 CrossRef CAS.
- Z. Yu, H. Ma, X. Liu, M. Wang and J. Wang, Review in life cycle assessment of biomass conversion through pyrolysis-issues and recommendations, Green Chem. Eng., 2022, 3(4), 304–312 CrossRef.
- A. Sarrion, E. Medina-Martos, D. Iribarren, E. Diaz, A. F. Mohedano and J. Dufour, Life cycle assessment of a novel strategy based on hydrothermal carbonization for nutrient and energy recovery from food waste, Sci. Total Environ., 2023, 878, 163104 CrossRef CAS PubMed.
- A. S. Giwa, N. J. Maurice, A. Luoyan, X. Liu, Y. Yunlong and Z. Hong, Advances in sewage sludge application and treatment: Process integration of plasma pyrolysis and anaerobic digestion with the resource recovery, Heliyon, 2023, 9(9), e19765 CrossRef CAS PubMed.
- Y. Li, B. Xing, Y. Ding, X. Han and S. Wang, A critical review of the production and advanced utilization of biochar via selective pyrolysis of lignocellulosic biomass, Bioresour. Technol., 2020, 312, 123614 CrossRef CAS PubMed.
- B. Ramesh, A. Saravanan, P. Senthil Kumar, P. R. Yaashikaa, P. Thamarai and A. Shaji,
et al., A review on algae biosorption for the removal of hazardous pollutants from wastewater: Limiting factors, prospects and recommendations, Environ. Pollut., 2023, 327, 121572 CrossRef CAS PubMed.
- I. Malico, R. Nepomuceno Pereira, A. C. Gonçalves and A. M. O. Sousa, Current status and future perspectives for energy production from solid biomass in the European industry, Renew. Sustain. Energy Rev., 2019, 112, 960–977 CrossRef.
- P. R. Yaashikaa, P. Senthil Kumar, V. P. Mohan Babu, R. Kanaka Durga, V. Manivasagan and K. Saranya,
et al., Modelling on the removal of Cr(VI) ions from aquatic system using mixed biosorbent (Pseudomonas stutzeri and acid treated Banyan tree bark), J. Mol. Liq., 2019, 276, 362–370 CrossRef CAS.
- A. Tshikovhi and T. E. Motaung, Technologies and Innovations for Biomass Energy Production, Sustainability, 2023, 15(16), 12121 CrossRef CAS.
- R. Mukti, M. T. A. P. Kresnowati and T. Setiadi, Challenges in Syngas Fermentation for Bioethanol Production: Syngas Composition, Eng. Chem., 2023, 1, 9–19 Search PubMed.
- I. G. Hakeem, A. Sharma, T. Sharma, A. Sharma, J. B. Joshi and K. Shah,
et al., Techno-economic analysis of biochemical conversion of biomass to biofuels and platform chemicals, Biofuels, Bioprod. Biorefin., 2023, 17(3), 718–750 CrossRef CAS.
|
This journal is © The Royal Society of Chemistry 2024 |
Click here to see how this site uses Cookies. View our privacy policy here.