DOI:
10.1039/D4FO01059K
(Paper)
Food Funct., 2024,
15, 8644-8660
Improving insulin resistance by sulforaphane via activating the Bacteroides and Lactobacillus SCFAs–GPR–GLP1 signal axis†
Received
11th March 2024
, Accepted 2nd June 2024
First published on 8th June 2024
Abstract
Background: Insulin resistance (IR) is closely associated with non-alcoholic fatty liver disease (NAFLD), and the gut microbiome contributes to the development of NAFLD. Sulforaphane (SFN) is a phytochemical in cruciferous vegetables that could improve lipid metabolism disorder. However, whether SFN can alleviate IR in NAFLD by regulating the intestinal flora remains unclear. Methods: SFN was administered to high fat diet (HFD)-fed Wistar rats for 10 weeks. Gut microbiota was analysed by 16S rRNA sequencing and the short chain fatty acids (SCFAs) by gas chromatography. The expression of tight junction protein and the numbers of Lactobacillus, Bacteroides and Bifidobacterium were determined by qPCR. The expression of G-protein-coupled receptor 41/43 (GPR41/43) was determined by western blot. A randomized controlled trial (RCT) was conducted in NAFLD patients with broccoli seed tablets (rich in SFN, 42 mg d−1) as intervention for 12 weeks. Thirty-six volunteers with abnormal glucose before the broccoli seed tablet treatment were selected in the intervention group to analyze their blood glucose, insulin, homeostasis model assessment-insulin resistance index (HOMA-IRI), homeostasis model assessment-insulin sensitivity index (HOMA-ISI) and glucagon-like peptide (GLP-1). Results: SFN reduced blood glucose and HOMA-IRI while increasing insulin sensitivity in HFD rats. SFN reduced glycogen synthase kinase 3 (GSK-3), phosphoenolpyruvate carboxykinase (PEPCK) activity, and phosphorylation of serine residues of IRS-2 induced by HFD. SFN reshaped the gut microbiota composition of HFD-induced rats and, especially, increased the content of Bacteroidaceae, Lactobacillaceae and Bifidobacteriaceae, which are related to the improvement from SFN of the blood glucose and HOMA-IRI. The increased numbers of Bacteroides and Lactobacillus were the targets of SFN to enhance the expression of tight junction proteins ZO-1 and occludin, thereby lowering lipopolysaccharide content to reduce inflammation, ultimately alleviating IR. Bacteroides and Lactobacillus produced SCFAs, which activated GPR41/43 to secrete GLP1. Moreover, it was also confirmed in RCT that SFN intervention increased the level of GLP1 in NAFLD patients, which was positively correlated with the reduction of blood glucose and HOMA-IR. Conclusions: SFN alleviated IR in NAFLD via the Bacteroides and Lactobacillus SCFAs–GPR41/43-GLP1 axis and protected the intestinal mucosal barrier to decrease inflammation.
1. Introduction
The global prevalence of non-alcoholic fatty liver disease (NAFLD) has been as high as 30.05% (1990–2019), and in most Asian countries, including China, the prevalence is more than 28%.1 The total number of NAFLD patients in China is expected to be 314.58 million by 2030.2 About 5%–20% of NAFLD patients will develop nonalcoholic steatohepatitis (NASH); 10%–20% of them will develop liver fibrosis. Approximately 5% of liver fibrosis will progress to cirrhosis, eventually leading to hepatic carcinoma.3 About half of the NAFLD patients have accompanying metabolic disorders such as type 2 diabetes mellitus (T2DM), hyperlipidemia, hypertension, and cardiovascular and cerebrovascular diseases.4,5 NAFLD has become a major social health problem worldwide.
Insulin resistance (IR) plays pivotal role in NAFLD and seems to accelerate the progression of liver disease. The overall prevalence of NAFLD among patients with T2DM was 55.5%, while the prevalence of NASH among T2DM patients worldwide was 37.3%.1 T2DM was found to be an independent predictor of advanced liver fibrosis in NAFLD patients (OR 2.9, 95% CI 1.3–6.1, P = 0.007).6 Around 47.3% of 1365 biopsy-proven Japanese NAFLD patients suffered from T2DM (OR 2.39, 95% CI 1.60–3.55, P < 0.001).7 Liver biopsy of 94 T2DM patients showed that 56% of them had steatohepatitis, and 50% of them had hepatic fibrosis (as described in a Hong Kong report8). All these results suggest that IR is involved in the progression of steatosis and NASH, followed by hepatic carcinogenesis.
Diacylglycerols (DAGs), the lipid intermediates of triglyceride synthesis from non-esterified fatty acids and glycerol, activate protein kinase C (PKC) isoforms which inhibit insulin signaling and induce hepatic IR.5 The liver does not respond properly to insulin, resulting in abnormally high glucose levels (hyperglycemia) and leading to the production and secretion of excess insulin to maintain glucose levels in the blood (hyperinsulinemia).9 Both hyperglycaemia and hyperlipidaemia stimulate de novo synthesis of lipids, which further aggravates lipid accumulation in the liver.10,11 Overgrowth of detrimental intestinal bacteria could change the permeability of intestinal mucosa, which leads to elevated levels of lipopolysaccharide (LPS) in blood, promoting the production of inflammatory cytokines and further causing IR.12 In one study, Enterococcus faecalis was significantly associated with changes in lipid metabolites and caused hepatic IR.13 Short chain fatty acids (SCFAs), small molecules produced by intestinal flora fermentation, inhibit the growth of harmful bacteria and reduce the production of pro-inflammatory factors, so they are conducive to the repair of mucosal inflammation.14 In addition, SCFAs could increase G-protein-coupled receptor (GPR) production to secrete glucagon-like peptide (GLP-1) and peptide YY (PYY), which could protect islet cells.15
Sulforaphane (SFN), an isothiocyanate rich in cruciferous vegetables, has shown benefits in the disorders of glucose and lipid metabolism. A double-blind RCT on T2DM patients showed that consumption of 5 or 10 g day−1 of broccoli containing nearly 22.5 μmol g−1 SFN could decrease fasting blood glucose, IR, cholesterol, and low-density lipoprotein levels, while increasing total anti-oxidant capacity.16 SFN could activate PI3K/AKT to mediate the interaction of insulin with its receptor.17 SFN also reduced IR in a high-fat diet (HFD) model via the AMPK–Nrf2–glutathione peroxidase 4 (GPx4) axis.18 Our studies found that SFN could improve lipid metabolism in hepatocytes by inhibiting endoplasmic reticulum stress (ERS) and decreasing lipid droplet (LD) peripheral protein expression to prevent LD formation.19,20 In addition, SFN balanced the Ca2+ homeostasis in the mitochondria-associated membrane (MAM) through regulating the Ca2+ channels.21 However, an RCT in male subjects with fatty liver showed that levels of ALT, AST, and γ-GTP in serum decreased by dietary supplementation with 30 mg of SFN precursor glucosinolates per day, while no remarkable impact on the markers of glucose metabolism.22
Multiple clinical trials emphasized the glucose-ameliorating effects of SFN in T2DM patients. However, the results of SFN intervention in the NAFLD population with IR are uncertain, and studies on the hidden mechanism are absent. We previously found that SFN could improve the imbalance of intestinal flora in mice with BBN-induced bladder cancer and inhibit inflammatory injury by LPS.23 Nrf2 and AMPK are classical targets of SFN to regulate oxidative stress and inflammation-induced IR.18 However, whether the ameliorating effect of SFN on IR during the NAFLD process in both patients and the rodent model is achieved by changing the structure of intestinal flora has not been reported. Furthermore, whether the specific mechanism involves activating GPR or secreting GLP-1 through SCFAs produced by intestinal flora is another important question we ask.
2. Materials and methods
2.1. Reagent and preparation
Broccoli seed tablets were donated by Shenzhen Fushan Biotechnology Co., Ltd. The main active ingredient was broccoli seed water extract, and the conversion rate within 2 h was as high as 55%; the converted SFN content could reach 30 mg g−1. D,L-Sulforaphane (1-isothiocyanato-4-methylsulphinylbutane, purity ≥97%) was purchased from Toronto Research Chemicals (Toronto, ON, Canada). Stock solution (100 mmol L−1) was prepared in dimethyl sulfoxide (DMSO) and stored at −20 °C. Triglyceride (TG), total cholesterol (TC), LPS and D-lactic acid (D-Lac) assay kits were purchased from Nanjing Jiancheng Biotechnologies (China). Insulin, GLP1 and PYY were quantified by ELISA kits purchased from Biotopped Life Sciences Biotechnologies (China). Tissue RNA extraction kit, TransScript One-Step gDNA Removal and cDNA Synthesis SuperMix kit, and TransStart Top Green qPCR SuperMix kit were purchased from TransGen Biotech (Beijing, China). TIANamp Stool DNA Kit (Tiangen, Beijing, China) was used to extract total DNA from fecal bacteria. Antibodies against GPR41, GPR43 and GAPDH were from Proteintech Biotechnologies (USA). Animal diet was purchased from Keaoxieli Feed Co., Ltd (Beijing, China).
2.2. Establishment of high fat diet (HFD)–induced animal model
Male Wistar rats (4–5 weeks old, weighing 150–200 g) were divided into 4 groups (n = 10). The detailed treatments are summarized in the ESI Fig. S1.† Rats in the control group were given chow diet (Con). Two groups of rats on high fat diet (HFD) were administered SFN (10 and 20 mg kg−1 respectively) by oral gavage three times a week for 10 weeks. The formula of the diet is described in a previous study.20 All rats were placed under a 12 h-light/12 h-dark cycle in a pathogen-free barrier facility with free access to water and food. All the procedures were conducted in conformity with the guidelines of the Institutional Animal Care Use Committee, Heilongjiang province, China. The protocols were approved by the Ethics Committee of Experimental Animals (Qualified number: SCXK (Hei) 2016-0010) at Heilongjiang University of Chinese Medicine.
2.3. Intraperitoneal injections for glucose tolerance test (IPGTT) and insulin tolerance test (IPITT)
At the beginning of the 8th week, rats were fasted for 12 hours. Glucose (20%) was injected intraperitoneally at a dose of 2 g kg−1. Tail blood samples were collected at 0, 30, 60, 90, and 120 min after glucose loading, and blood glucose concentrations were measured using a glucometer (Roche Diagnostics, Germany). The area under the curve of glucose (AUC glucose) values for 0–120 min post glucose load were calculated. At the beginning of the 9th week, rats were fasted for 4 hours. The insulin injection dose was 0.75 mu g−1. Tail blood samples were collected at 0, 30, 60, 90 and 120 min after insulin load, and blood glucose concentrations were measured by a glucometer (Roche Diagnostics, Germany). Calculation formulas for the homeostasis model assessment-insulin resistance index (HOMA-IRI) and homeostasis model assessment-insulin sensitivity index (HOMA-ISI) are as follows: HOMA-IRI = fasting blood glucose (mmoL L−1) × fasting insulin (μU mL−1)/22.5; HOMA-ISI = 1/HOMA-IRI.24
2.4. Measurement of serological indicators and pathological examination of cecum
Blood samples were collected from the abdominal aorta, centrifuged at 3000g for 15 min to obtain serum, which was stored at −80 °C. TG, TC, LPS and D-Lac were measured by detection kits according to the manufacturer's protocols. HK, GSK-3, PEPCK, insulin, GLP1 and PYY were quantified by ELISA kits according to the manufacturer's protocols. Cecum sections were fixed in 4% paraformaldehyde solution for 24 h and sent to the Department of Pathology, Heilongjiang University of Chinese Medicine, for slide preparation and hematoxylin–eosin staining. The slides were reviewed in a blinded fashion by two pathologists from Heilongjiang University of Chinese Medicine.
2.5. Gut microbiome sampling and sequencing
TIANamp Stool DNA Kit (Tiangen, Beijing, China) was used to extract bacterial DNA according to the manufacturer's instructions. Prokaryotic 16S rDNA V3 and V4 hypervariable regions were selected for generating amplicons, followed by taxonomic analysis. The specific primers targeting the 16S rDNA V3 and V4 hypervariable regions of bacteria were designed by GENEWIZ. The sequences of the forward primers and reverse primers are 3′ CCTACGGRRBGCASCAGKVRVGAAT 5′ and 5′ GGACTACNVGGGTWTCTAATCC 3′, respectively. DNA libraries were validated by an Agilent 2100 Bioanalyzer and quantified by a Qubit 2.0 Fluorometer. Image analysis and base calling were performed using MiSeq Control Software (MCS).
2.6. Analysis of intestinal flora of feces in rats
Faecal microbiota stool samples were randomly collected from rats that were fasted for 20 h. Stools were consecutively and aseptically collected for 3 days at the 10th week (the end of experiment), then mixed evenly into three samples for each group and stored at −80 °C for the extraction of DNA. The DNA was extracted by a stool DNA kit according to the manufacturer's instructions. The specific primers for Lactobacillus, Bifidobacteria and Bacteroides were designed according to the 16srRNA V3 sequence from the BLAST gene library. The sequences of primers are shown in Table 1. The primers were synthesized by Dalian Bao Biotechnology Co., Ltd. DNA was then subjected to quantitative PCR using the QuantiFast SYBR Green PCR kit. Results are expressed as number of bacteria per g of stool.
Table 1 Primers for Lactobacillus, Bifidobacteria and Bacteroides
Gene |
Primers (5′-3′) |
Lactobacillus
|
F AGCAGTAGGGAATCTTCCA |
R ATTTCACCGCTACACATG |
Bifidobacteria
|
F GGGTGGTAATGCCGGATG |
R CCACCGTTACACCGGGAA |
Bacteroides
|
F CTGAACCAGCCAAGTAGCG |
R CCGCAAACTTTCACAACTGACTTA |
2.7. RNA extraction and real-time quantitative PCR
After isolation of total RNA using TRIZOL reagent kit (TransGen Biotech, China) and quantification by measuring the optical density at 260 nm and 280 nm, cDNA was synthesized using the Transcript One-Step gDNA Removal and cDNA Synthesis SuperMix kit (TransGen Biotech, China) according to the manufacturer's protocol. The first strand obtained was quantified by real-time quantitative PCR using a Passive Reference Dye I (50) assay on the ABI Prism 7300 System (Applied Biosystems, CA). The sequences of primers for tight junction proteins are shown in Table 2. The PCR cycle conditions consisted of 94 °C for 31 s, then 94 °C for 5 s, and 60 °C for 30 s (40 cycles).
Table 2 Primers for tight junction proteins
Gene |
Primers (5′-3′) |
ZO-1 |
F CCATCTTTGGACCGATTGCTG |
R TAATGCCCGAGCTCCGATG |
Occludin |
F TTGGGAGCCTTGACATCTTGTTC |
R GCCATACATGTCATTGCTTGGTG |
GAPDH |
F CCCCCAATGTATCCGTTGTG |
R TAGCCCAGGATGCCCTTTAGT |
2.8. SCFA concentrations quantified by gas chromatography (GC)
SCFAs, including acetate, propionate, and butyrate in the feces, were analyzed by GC. Fecal samples (200 mg) were collected directly into sterile tubes from live rats, homogenized in 1 mL of sterile water, and then oscillated for 10 min. The suspension was centrifuged at 18
800g for 10 min, and the supernatant was again centrifuged at 18
800g for 15 min. The supernatant was subjected to 0.45 μm membrane filtration. The filtrate (1 mL) and formic acid (0.1 mL) were taken into a gas-phase flask for the analysis of the SCFAs.
2.9. Extraction of protein and western blot analysis
Total protein extraction and western blot analysis for the total lysate were performed as described previously.21
2.10. Randomized control trial for NAFLD patients
2.10.1. Ethical approval.
This randomized controlled trial (RCT) was approved by the Ethics Committee of the 1st Affiliated Hospital of Wenzhou Medical University, China (KY2021-114), and registered at the Chinese Clinical Trial Registry (https://www.chictr.org.cn) as ChiCTR2100050687. The intervention trial was performed in accordance with the principles of the Declaration of Helsinki and referred to the report as appropriate. All participants signed the informed consent before the trial.
2.10.2. Participants.
Calculations of participant data were performed as follows: 45% of patients in the intervention group and 19% of patients in the control group were assumed to have remission of NAFLD, α = 0.05 (unilateral), β = 0.10. Less than 20% of patients were lost to follow-up, and the resulting sample size for each group was calculated using PASS version 15.0 as N1 = N2 = 70. NAFLD patients at 20–70 years of age were recruited in Wenzhou (a city in south China). Inclusion and exclusion criteria used to screen patients complied with the Guidelines for the Prevention and Treatment of Non-alcoholic Fatty Liver Disease (2018 Updated Edition) revised by the Hepatology Branch of the Chinese Medical Association.
Inclusion criteria were as follows: the patient has been diagnosed with NAFLD by a licensed physician; no history of alcohol consumption or a weekly consumption of ethanol (alcohol) less than 210 g for men and 140 g for women in the past 12 months; no specific diseases which could cause fatty liver such as viral hepatitis, drug-induced liver disease, total parenteral nutrition, hepatolenticular degeneration, or autoimmune liver disease; the liver imaging findings showed diffuse, focal, or homogeneous fatty liver. Exclusion criteria are patients with type 1 diabetes mellitus, severe kidney disease, cardiovascular disease, stroke, cancer, alcoholic liver disease, chronic hepatitis C, autoimmune liver disease, hepatolenticular degeneration, or psychological disorders; pregnant or lactating women; patients who recently took drugs that could cause liver aminotransferase to increase, or diet pill and lipid-lowering drugs in the past six months; patients who took broccoli or other SFN-rich nutritional supplements within the last 1 month; and patients who needed long-term systemic steroid therapy. According to the inclusion and exclusion criteria, 123 participants were finally selected for the following intervention study.
2.10.3. Study design.
Simple randomization method was used in this study, and patients were randomly grouped according to the order of enrollment. The blinding work of placebo and broccoli seed tablet was completed by personnel unrelated to this clinical trial, and the whole blinding process was supervised and controlled by special personnel. Patients and medical staff do not know the grouping situation. Only when the subject has an emergency situation can the investigator urgently unblind the subject to understand the experimental medication situation. Once unblinded, the subject is immediately withdrawn from the trial.
NAFLD patients meeting the inclusion criteria were randomly divided into control group and SFN intervention group. While maintaining their original diet and exercise habits, patients in the SFN group took six broccoli seed tablets (rich in SFN 42 mg d−1) three times a day, while the control group took placebos. Both groups of patients were instructed to take the drug before the formal start of the study, and the entire intervention process lasted for 12 weeks.
2.11. Collection and analysis of participant blood samples
Blood samples were collected and examined at baseline and at the end of the trial for biochemical indicators. Before collecting blood in the morning, all participants were asked to fast after 7 pm the night before. At the end of the trial, fasting venous blood samples from subjects (>12 h fast) were collected by uniformly trained nurses in the 1st Affiliated Hospital of Wenzhou Medical University at 7
:
00 am. Serum samples were obtained by centrifugation at 3000g for 10 min and stored at −80 °C (200 μL per tube) until analysis (within 1 month). Fasting glucose and insulin were tested by Adicon Medical Laboratory Center; GLP was quantified by ELISA kits according to the manufacturer's protocols.
2.12. Statistical analysis
All statistical analyses were performed on independent samples, and the results are expressed as mean standard deviation (mean ± SD). SPSS 19.0 and GraphPad Prism 6.0 were mainly used for the analysis and plotting. Data conforming to the normal distribution were tested by one-way ANOVA or Tukey's t-test, and non-conforming data were tested by Wilcoxon's signed rank test, with the level of significance defined as P < 0.05. Indicators of population GLP1 and diabetes mellitus diagnosis were analyzed using the GraphPad Prism 6.0 for correlation analysis. Pearson correlation test was performed for indicators that conformed to normal distribution; otherwise, Spearman correlation test was used. The method of correlation analysis between intestinal flora and LPS and SCFAs in animals was consistent with that of the population; the correlation analysis of intestinal flora, SCFAs, and biochemical indicators was performed using R Studio software.
3. Results
3.1. Improvement in glucose tolerance and IR by SFN in HFD-induced rats
SFN significantly reduced the body weight levels of serum TG and TC (ESI Fig. S2A–C†) in HFD rats. The blood glucose concentration in the SFN treatment group was significantly lower than that of the HFD group at different time points, and the AUC showed a similar trend (Fig. 1A & ESI Fig. S2D†). SFN improved the insulin tolerance of rats on HFD (Fig. 1B). SFN reduced high serum glucose and the insulin levels induced by HFD (Fig. 1C and D). HFD increased the HOMA-IRI (Fig. 1E), while it decreased the HOMA-ISI of rats (Fig. 1F). However, SFN reduced the HOMA-IRI while increasing the HOMA-ISI. In summary, SFN has the potential to reduce IR and increase insulin sensitivity in HFD rats.
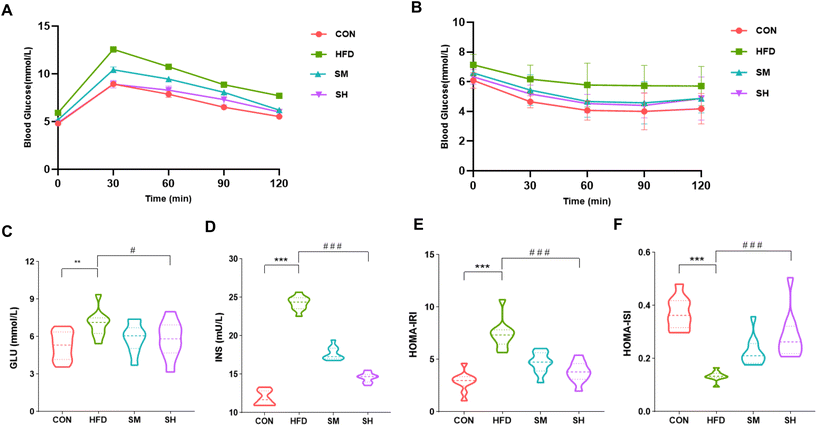 |
| Fig. 1 (A) Glucose tolerance measured by IPGTT at week 8. (B) Insulin tolerance measured by IPITT at week 9. (C) Fasting glucose and (D) insulin levels in blood at week 10. (E) HOMA-IRI of rats. (F) HOMA-ISI of rats. Data are presented as mean ± SD; significance was determined by one-way ANOVA corrected for multiple comparisons with Tukey's test. Compared to control group, ***P < 0.001; compared to HFD group, ###P < 0.001. | |
The insulin signaling pathway is mainly mediated via the insulin receptor substrate (IRS). IRS is a member of the tyrosine kinase receptor family. Insulin can change the conformation of the insulin receptor and activate its kinase activity, which phosphorylates the IRS. The phosphorylation sequence of IRS activates the phosphoinositide 3-kinase (PI3K) catalytic subunit, passes the insulin signal, and plays a role in hypoglycemic action. For example, PI3K inhibits glycogen synthase kinase 3 (GSK-3) to increase glycogen synthesis. PI3K also represses the transcription factor-fork box protein (FOX) activity, which regulates phosphoenolpyruvate carboxykinase (PEPCK), the key enzymes of gluconeogenesis, to reduce blood glucose25 (Fig. 2A). In our study, SFN significantly increased hexokinase (HK) activity, which catalyzes glucose to produce glucose 6-phosphate (G-6-P) (Fig. 2B). SFN reduced the high GSK-3 and PEPCK activity induced by HFD (Fig. 2C and D).
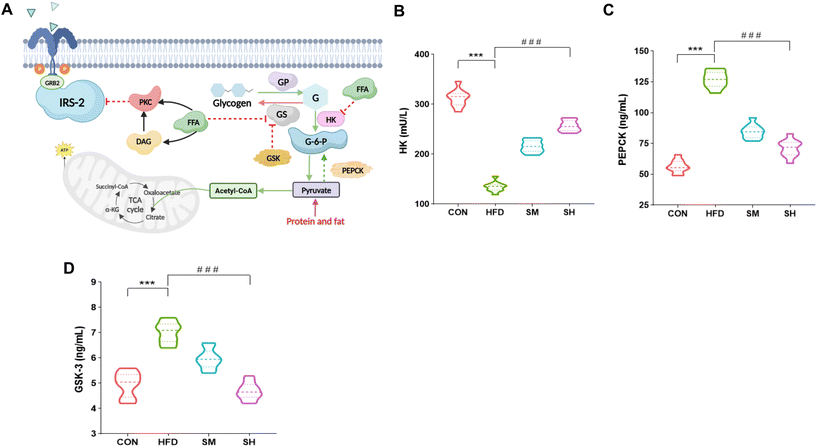 |
| Fig. 2 (A) Insulin signaling pathway. Insulin can change the conformation of the insulin receptor and activate its kinase activity, which activates the insulin receptor substrate (IRS). The phosphorylation sequence of IRS activates the phosphoinositide 3-kinase (PI3K) catalytic subunit and then inhibits glycogen synthase kinase 3 (GSK-3) to increase glycogen synthesis. On the other hand, PI3K represses the transcription factor-fork box protein (FOX) activity to regulate phosphoenolpyruvate carboxykinase (PEPCK), the key enzyme of gluconeogenesis, to reduce blood glucose. (B) HK, (C) PEPCK and (D) GSK-3 levels in rats’ liver. Data are presented as mean ± SD, and significance was determined by one-way ANOVA corrected for multiple comparisons with Tukey's test. Compared to control group, ***P < 0.001; compared to HFD group, ###P < 0.001. | |
3.2. SFN reshaped the gut microbiota composition of HFD-induced rats
Given the close relationship between gut microbiota and IR, we performed 16S rRNA gene sequencing on the stools of HFD rats. Venn diagrams of the operational taxonomic units (OTUs) showed that the overlap area of CON and HFD groups was large and the similarity was high. The number of specific OTUs of the SFN group significantly increased (ESI Fig. S3A and B†). Additionally, flora diversity was not significantly different, but increased bacterial richness (Chao index) was observed in rats administered with high-dose SFN intervention (Fig. 3A–C and Supplementary Fig. 3C†). Principal coordinate and non-metric multidimensional scaling analysis also showed that the microbiota composition clustered distinctly for rats in the SFN group and the HFD rats (Fig. 3D and E).
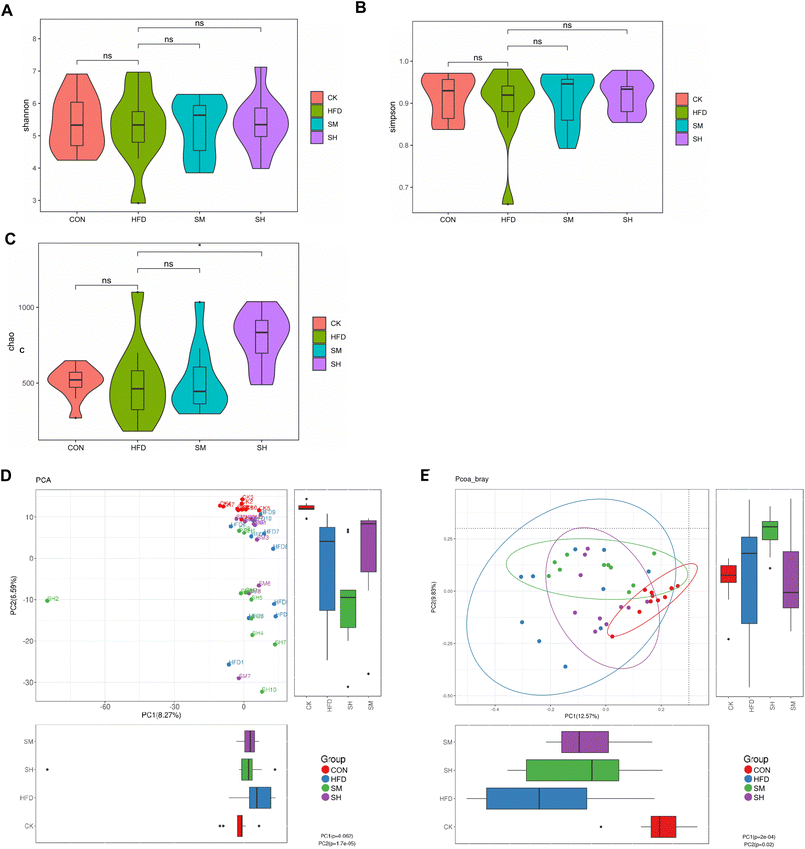 |
| Fig. 3 Significant reshaping of the structure of gut microbiota by SFN. The intestinal flora data were mainly analyzed using OUT, then the Ribosomal Database Program (RDP) classifier. A random sampling method of the sample sequence was adopted to calculate the α diversity indexes: (A) Shannon index, (B) Simpson index, (C) Chao 1 index. (D) Principal coordinate analysis (PCA) and (E) PCoA diagram. Data are presented as mean ± SD, and significance was determined by one-way ANOVA corrected for multiple comparisons with Tukey's test. Compared to control group, ***P < 0.001; compared to HFD group, ###P < 0.001. | |
Several bacterial OTUs were differentially abundant in rats fed with HFD compared with the SFN group (Fig. 4A). Also, among others, we observed the enrichment of Ruminococcaceae (most strains could produce SCFAs), Christensenellaceae, Laevis, and Lactobacillus, as well as the depleted abundance of harmful bacteria such as Clostridiales vadin BB60 group, Pseudomonas, Bacteroides, Enterobacteriaceae, Clostridiaceae, and Desulfovibrioceae in the SFN group. Moreover, at the genus level, SFN significantly increased the abundance of Rumenococcus-UCG-014, Rumenococcus-UCG-005, Lacetospiraceae NK4A136, Bifidobacterium, Vibrio butyricum, Pseudomonas, Klebsiella, Facklamia, and Alistipes (Fig. 4B–F and ESI Fig. S4†). The ratio of Bacteroides/Firmicutes (B/F value) in the SFN group significantly decreased, which indicates a reduced degree of intestinal flora disorder induced by HFD (Fig. 4G). These results suggested that SFN could reshape the intestinal flora disorder caused by HFD.
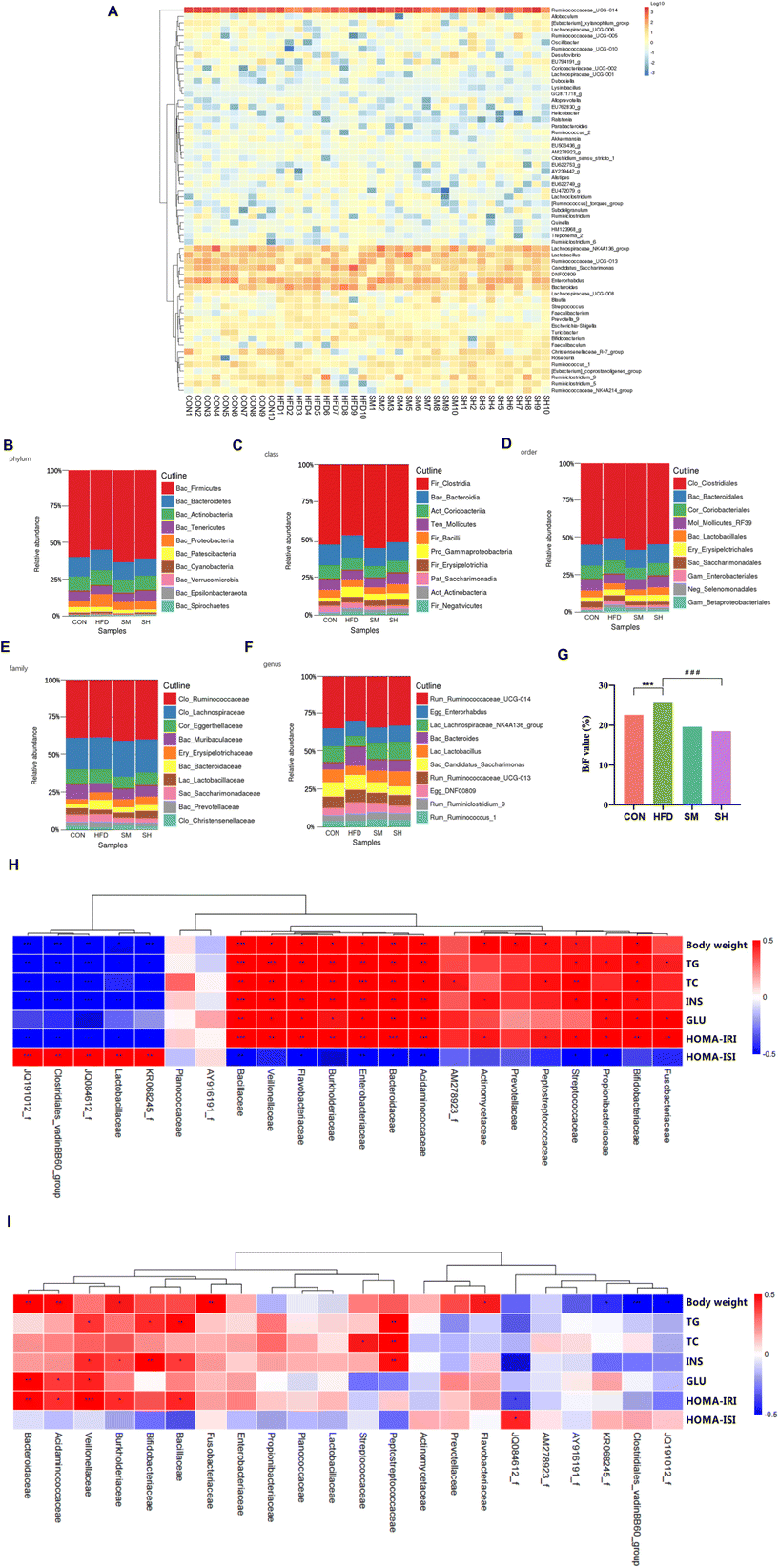 |
| Fig. 4 Characterization of intestinal microbiome. (A) Heatmap plot of the bacteria. Characterization of intestinal flora at the (B) phylum level, (C) class level, (D) order level, (E) family level and (F) genus level. (G) The ratio of Bacteroides/Firmicutes (B/F value). (H) Correlation of bacteria in HFD mice and (I) SFN intervention group abundance with phenomes. Data are presented as mean ± SD, and significance was determined by one-way ANOVA corrected for multiple comparisons with Tukey's test. Compared to control group, ***P < 0.001; compared to HFD group, ###P < 0.001. | |
Correlation analysis was performed to determine the potential association of bacterial abundance with phenomes. We observed that Bacillaceae, Flavobacteriaceae, Burkholderiaceae, Enterobacteriaceae, Bacteroidaceae, Acidaminococcaceae, and Bifidobacteriaceae, which were enriched in fecal samples of HFD rats, were positively correlated with body weight, TG, TC, INS, GLU and HOMA-IRI levels in serum, and negatively correlated with HOMA-ISI (except Bifidobacteriaceae). On the contrary, JQ191012-f, Clostridiales vadin BB60 group, JQ084612-f, Lactobacillaceae, and KR068245-f were negatively correlated with body weight, TG, TC, INS and HOMA-IRI, while positively correlated with HOMA-ISI (Fig. 4H). After SFN intervention, Bacteroidaceae and Acidaminococcaceae were no longer positively related to TG, TC and INS. Bifidobacteriaceae was only positively correlated with TG and INS. Burkholderiaceae, Enterobacteriaceae, Bacillaceae were all uncorrelated with certain biochemical markers (Fig. 4I). The positive correlation between HOMA-ISI and several bacteria represented by Lactobacillaceae also disappeared (Fig. 4I). These results suggest that gut microbiota dysbiosis correlates with HFD. However, the improvement of SFN on GLU and HOMA-IRI may be related to some bacteria such as Bacteroidaceae, Bifidobacteriaceae, Enterobacteriaceae, Bacillaceae and Lactobacillaceae.
3.3. The protection of intestinal permeability by SFN may be related to the increase of Bacteroides and Lactobacillus
Disturbance of the intestinal flora could damage the intestinal mucosa and enhance its permeability. Therefore, the levels of LPS and D-Lac, the marker macromolecules for evaluating intestinal permeability, were detected to clarify the effect of SFN on intestinal mucosal permeability. SFN significantly reduced the high levels of LPS, but not D-Lac, in the serum of HFD rats (Fig. 5A and ESI Fig. S5A†). Compared with the control group, the mRNA expression of tight junction proteins ZO-1 and occludin were significantly decreased in the HFD group, while SFN increased their expression (Fig. 5B and C). The structure of mucous membrane in the control group is intact. However, HFD led to damage of the villi and crypt structure; the intestinal villi extensively fell off, the crypt openings widened, and the lamina propria were infiltrated with a large number of inflammatory cells. SFN significantly improved the structure of the cecum and crypts. Furthermore, the inflammatory response was also alleviated (Fig. 5D).
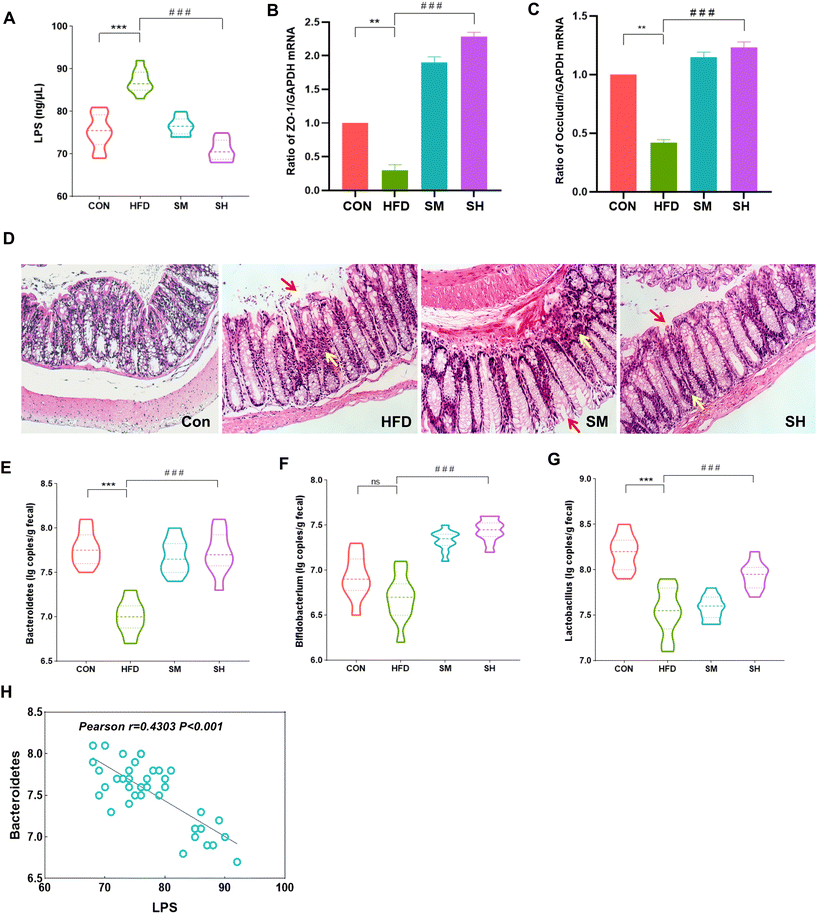 |
| Fig. 5 (A) LPS level in serum. (B) ZO-1 mRNA and (C) occludin mRNA expression of intestine. (D) H&E staining of cecum for representative gross morphology. The yellow arrows represent inflammatory cells, and red arrows represent crypt openings. DNA expression of (E) Bacteroides, (F) Bifidobacteria and (G) Lactobacillus. (H) The correlation analysis between Bacteroides and LPS. Data are presented as mean ± SD, and significance was determined by one-way ANOVA corrected for multiple comparisons with Tukey's test. Compared to control group, **P < 0.01; compared to HFD group, ###P < 0.001. | |
Bacteroides, Bifidobacteria and Lactobacillus were capable of consolidating the structure of tight junction proteins, maintaining the integrity of the intestinal mucosa. Moreover, SFN intervention changed the correlation between these three bacteria and TG, TC, INS, GLU, HOMA-IRI and HOMA-ISI. Therefore, the numbers of these three bacteria were measured. Compared with the control group, the numbers of Bacteroides and Lactobacillus in the HFD group were significantly lowered. High-dose SFN significantly increased the numbers of Bacteroides, Bifidobacteria and Lactobacillus (P < 0.01) (Fig. 5E–G). The correlation analysis between Bacteroides, Bifidobacteria, Lactobacillus and LPS was carried out to further explore whether the decreased endotoxin from SFN could be related with the intestinal flora. The number of Bacteroides is significantly and negatively correlated with the level of endotoxin (Fig. 5H). The number of Bifidobacteria and Lactobacillus were, respectively, positively and negatively correlated with LPS, but the correlation is weak (ESI Fig. S5B and C†). The above results suggested that the decrease in LPS level in rats by SFN treatment may be related to the increase in the numbers of Bacteroides and Lactobacillus in the intestinal flora. SFN increased the numbers of Bacteroides and Lactobacillus, thereby protecting the intestinal mucosal barrier, decreasing LPS content to reduce inflammation, and ultimately alleviating IR.
3.4.
Lactobacillus and Bacteroides mediates the SCFAs–GPR–GLP signaling axis to regulate glucose by SFN
Intestinal flora produce SCFAs, which not only regulate the composition of the intestinal flora, but also act as signal molecules to reduce blood glucose through GPR. Compared with the control group, the quantities of acetic acid, propionic acid and butyric acid in the intestine of the HFD group were significantly decreased, while they were significantly increased by SFN (Fig. 6A–C). We observed that the abovementioned SCFAs were positively correlated with HOMA-ISI and negatively correlated with glucose, INS, TG, TC and HOMA-IRI levels in serum (Fig. 6D). The correlation between bacteria and SCFAs was analyzed to determine the influence of SFN on the relationship between SCFAs and target bacteria. The numbers of Lactobacillus and Bacteroides were positively correlated with the levels of acetic acid, propionic acid and butyric acid, respectively. In contrast, the correlation between the number of Bifidobacteria and SCFAs was very weak. The results suggested that the increase in the levels of acetic acid, propionic acid and butyric acid caused by SFN may be related to the increase in the numbers of Lactobacillus and Bacteroides in the intestinal tract of rats, and had nothing to do with the number of Bifidobacteria (Fig. 6E–G and ESI Fig. S5D†).
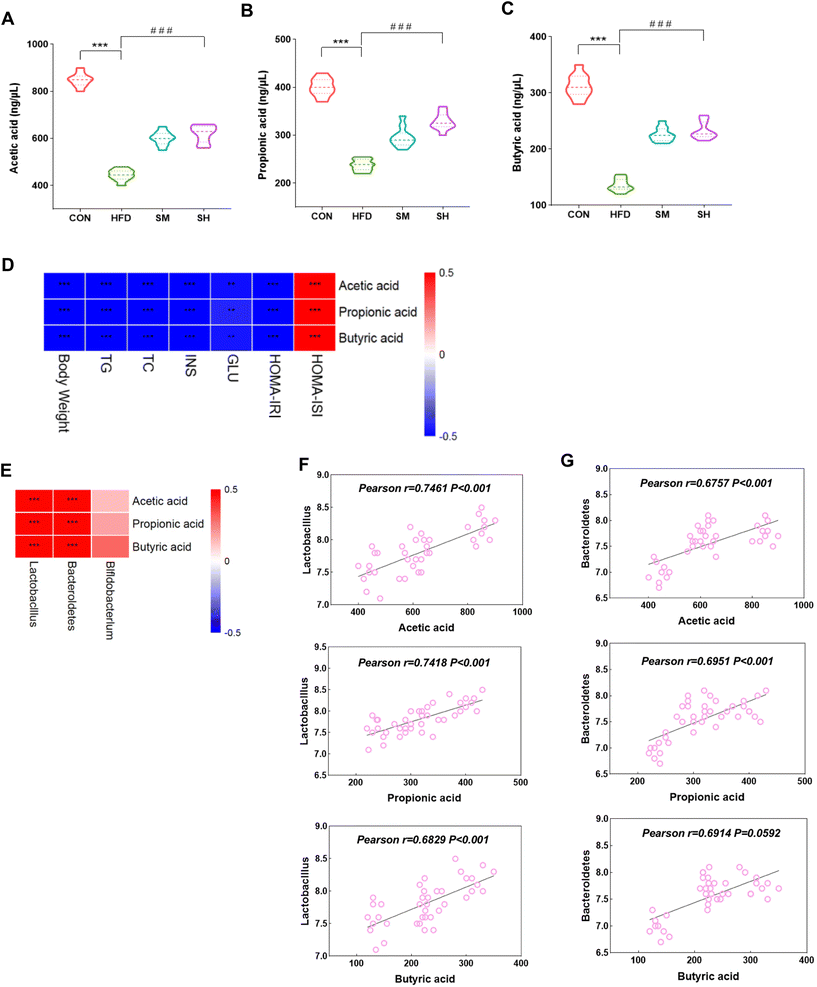 |
| Fig. 6 (A) Acetic acid, (B) propionic acid and (C) butyric acid content in the intestine measured by GC. (D) The association of serological indicators and SCFAs. (E) The association of bacteria and SCFAs. (F) The correlation between Lactobacillus and acetic acid, propionic acid and butyric acid. (G) The correlation between Bacteroides and acetic acid, propionic acid and butyric acid. Data are presented as mean ± SD, and significance was determined by one-way ANOVA corrected for multiple comparisons with Tukey's test. Compared to control group, **P < 0.01; compared to HFD group, ###P < 0.001. | |
SCFAs activates intestinal mucosal L cells to make use of two G-protein-coupled receptors (GPR), GPR43 and GPR41, on the L cell surface. GPR43 and GPR41 increased peptide YY (PYY) and GLP1 secretion. GLP1 has hypoglycemic and anti-inflammation function, therefore protecting islet cells and alleviating IR. In our study, SFN up-regulated the expression of GPR41 and GPR43 (Fig. 7A). HFD significantly reduced the level of the hormone GLP1 secreted by SCFA receptors in the intestine of rats, while high-dose SFN increased the GLP1 level. SFN had no regulatory effect on the secretion of PYY in the downstream receptors of SCFAs (Fig. 7B and C).
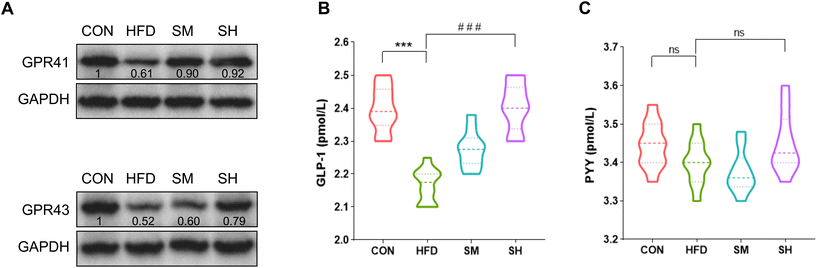 |
| Fig. 7 (A) Western blot analysis of GPR41 and GPR43 of intestine. (B) GLP1 and (C) PYY level in intestine. Data are presented as mean ± SD; significance was determined by one-way ANOVA corrected for multiple comparisons with Tukey's test. Compared to control group, **P < 0.01; compared to HFD group, ###P < 0.001. | |
3.5. SFN improved IR in NAFLD adults with IR
To investigate the effects of SFN on multiple glucose metabolism parameters and whether they are associated with GLP1, 154 subjects were recruited. The participation of subjects is shown in the Fig. 8 flowchart. After signing the informed consent, baseline blood samples were collected and the questionnaires were completed. According to the Guidelines for the Prevention and Treatment of Non-alcoholic Fatty Liver Disease (2018 Updated edition) revised by the Hepatology Branch of the Chinese Medical College, B-ultrasound detection was performed on 154 participants. Combined with serological indicators, 143 participants met the requirements of this study. However, three participants discontinued participating in the trial. Finally, 140 subjects with NAFLD agreed to participate in the trial and were randomly divided into two groups (N1 = N2 = 70). In the control group, six participants withdrew from the trial, and four participants lost contact. In the intervention group, seven participants dropped out of the trial. The follow-up loss rate was 12.1%, which was within the reasonable range. The intervention group took six broccoli seed tablets (rich in SFN 42 mg d−1) three times a day, while the control group took placebos. Both groups of patients were instructed to take the drug before the formal start of the study, and the entire intervention process lasted for 12 weeks.
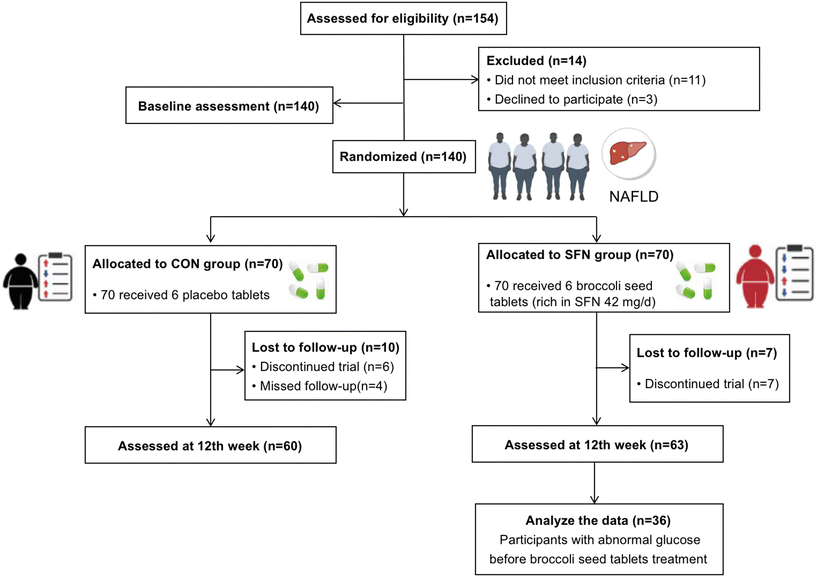 |
| Fig. 8 Flowchart of participants through the trial. | |
Thirty-six volunteers with abnormal glucose before broccoli seed tablet treatment were selected from the intervention group to analyze the data. We thoroughly analyzed the variations in terms of five glucose metabolism parameters, including blood glucose (GLU), INS, HOMA-IRI, HOMA-ISI and GLP1. Compared to baseline values, GLU, INS and HOMA-IR were significantly reduced at the end of the broccoli seed tablet intervention (P < 0.001, P = 0.0409, and P = 0.0175 respectively), while HOMA-ISI and GLP1 increased (P = 0.0121 and P = 0.0027 respectively). However, there was still a small portion of the subjects in which these parameters were stable after broccoli seed tablet intervention (Fig. 9A–E). It is worth mentioning that GLP1 was negatively related to GLU, INS and HOMA-IR (Fig. 9F–I). Overall, the 12 week-long broccoli seed tablet intervention produced clinical effects, but the effect was person-specific with inter-individual variability. In summary, the decreasing effect of the SFN on GLU, INS and HOMA-IR was related to the increase of GLP1.
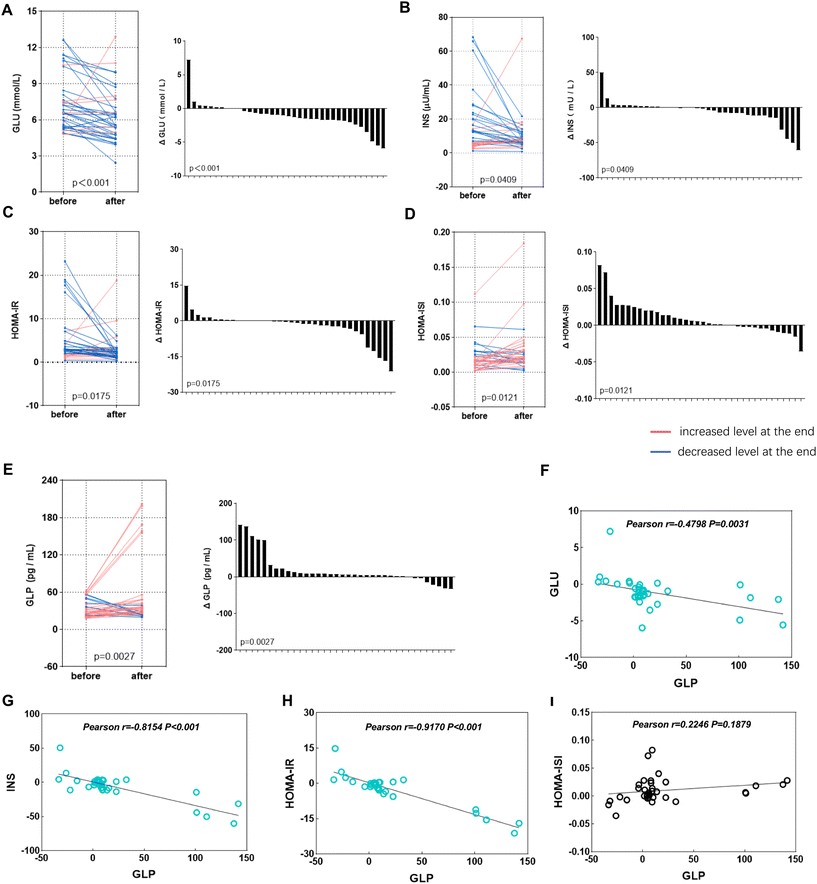 |
| Fig. 9 The effects of SFN intervention on (A) blood glucose, (B) insulin, (C) HOMA-IR, (D) HOMA-ISI and (E) GLP at the individual level and the change after intervention in NAFLD patients. Red lines indicate the individuals with increased level at the end compared with before intervention. Blue lines indicate individuals with decreased level at the end compared with before intervention. The p values represent the results of significance tests of the average changes after the intervention (n = 36). The correlation between GLP and (F) blood glucose, (G) INS, (H) HOMA-IR, and (I) HOMA-ISI. Pearson correlation test was performed for indicators that conformed to normal distribution. | |
4. Discussion
Disorders of glucose and lipid metabolism could lead to IR, which is a key cause of obesity, T2DM and NAFLD.26 This study found that SFN increased the ratio and abundance of Lactobacillus and Bacteroides in the intestine of HFD rats. Lactobacillus and Bacteroides produced SCFAs, which activated the GPR-gastrointestinal hormone pathway to secrete GLP1. In addition, SFN reduced circulating LPS levels and increased the expression of tight junction proteins ZO-1 and occludin to maintain the stability of the intestinal mucosal barrier, thereby reducing inflammatory response and improving IR (Fig. 10). In NAFLD patients, it was further verified that intervention with broccoli seed tablets could improve IR, along with reducing blood glucose and increasing GLP1 levels. GLP1 levels were negatively correlated with HOMA-IR and blood glucose levels, which suggested that SFN may reduce IR by increasing GLP secretion.
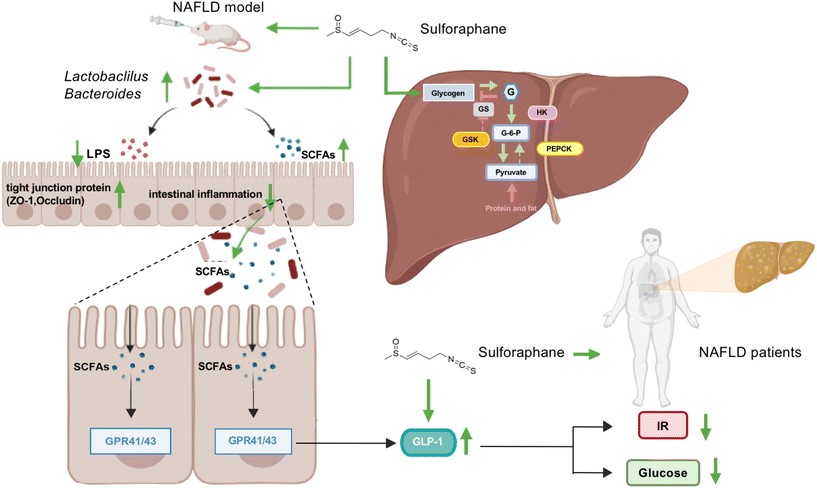 |
| Fig. 10 SFN altered the composition of gut microbes, especially increasing the content of Bacteroides and Lactobacillus, which activated the SCFAs–GPR–GLP1-related pathways to play a hypoglycemic role. On the other hand, the increasing number of Bacteroides and Lactobacillus by SFN contributed to restoring the intestinal mucosal barrier, which reduced the LPS level, thus decreasing inflammation and alleviating IR. In addition, SFN not only inhibited GSK-3 and promoted the oxygenation of glucose, but also increased glycogen synthesis by increasing HK and inhibiting PEPCK via regulating the IRS-2 signaling pathway. | |
GLP1 is an endogenous intestinal hormone with hypoglycemic effects, and there is strong evidence indicating that GLP1 increases insulin sensitivity.27 Further trials on healthy humans carried out with GLP1 antagonists confirmed their actions on glucagon secretion, and as predicted, their role in post-glucose insulin secretion.28 The end products of intestinal flora metabolism are SCFAs. SCFAs promote the secretion of hormones by intestinal mucosal L cells, which make use of GPR43 and GPR41 on their surface to secrete GLP 1, playing a hypoglycemic role and alleviating IR. Thus, GPR41 and GPR43 play a connecting role in the signal transmission of SCFAs.29 In our study, SFN increased the content of SCFAs in the intestine of HFD rats, thereby stimulating the expression of its receptor GPR41/43 and, furthermore, increased GLP1 content both in NAFLD patients and rats to regulate insulin sensitivity. The regulatory effect of SFN on SCFAs–GPR–GLP1 signaling was achieved by increasing the number of Lactobacillus and Bacteroides (SCFA-producing bacteria). In addition, there are also studies that show GLP-1 improved inflammatory macrophage-derived IR by inhibiting the NF-κB pathway and secretion of inflammatory cytokines in macrophages.30 Interestingly, SFN could also play an anti-inflammatory role by suppressing the NF-κB pathway.31 Therefore, aside from Lactobacillus and Bacteroides SCFAs–GPR–GLP1 signaling, whether the regulation of SFN on GLP is related to the NF-κB pathway requires further study.
Imbalance of the intestinal flora induced by HFD destroyed the tight junctions of intestinal epithelial cells, increasing the permeability of the intestinal mucosa, so that the Gram-negative cell wall component LPS entered the blood, causing IR.32 In a prospective cohort study of patients with NAFLD and obesity, LPS levels were high in the patients’ plasma, and as the disease progressed, LPS induced an inflammatory response that destroyed islet cells.33 SFN reduced LPS levels in serum. On the one hand, SFN increased the abundance of probiotics represented by Bacteroides and Lactobacillus in the intestinal tract, thus inhibiting the growth of harmful bacteria that produce LPS. On the other hand, SFN increased the expression of tight-linking proteins to block LPS release to the blood. Due to the increase in intestinal permeability, D-Lac enters the blood, but it is not absorbed, so it could be used as an index to evaluate the intestinal permeability.34 SFN reduced the LPS level, while the content of D-Lac did not change much. This may be due to an increase of probiotics in the gut, which leads to a decrease in the number of various D-lac-producing bacteria in the gut.
The enhanced HK level via SFN in our study indicated that SFN may promote the oxygenolysis of glucose. HK catalyzes glucose to produce glucose 6-phosphate (G-6-P), which is the important intermediate product in glucose metabolism. G-6-P generates pyruvate to enter the Krebs cycle and increase the oxidation of glucose.35 This may be the reason that SFN reduced the fasting blood glucose level of rats. Insulin receptor substrate-2 (IRS-2) is the major isoform expressed in liver, mainly responsible for glycogen synthesis and inhibiting hepatic glucose output.36 Once hepatic IR happens, the IRS-2 serine residues are phosphorylated, thus inhibiting the phosphorylation of tyrosine kinase.37 In our study, depression of GSK-3 and PEPCK by SFN may be due to increasing glycogen synthesis via regulating the IRS-2 signaling pathway.
5. Conclusion
In summary, SFN remediated the intestinal flora disorder in HFD-induced rats, especially increasing the content of Bacteroides and Lactobacillus, which protected the intestinal mucosal barrier to decrease inflammation and ultimately alleviate IR. More importantly, Bacteroides and Lactobacillus produced SCFAs, signaling molecules that activated GPR41/43 on the intestinal mucosal L cell surface to secrete GLP1 to improve IR. Moreover, it was also confirmed in RCT that SFN intervention increased the level of GLP1 in NAFLD patients, which was positively correlated with the reduction of blood glucose and HOMA-IR. In a word, the Bacteroides and Lactobacillus SCFAs–GPR–GLP1 axis was the new target of SFN to alleviate IR in NAFLD.
Abbreviations
SFN | Sulforaphane |
T2DM | Type 2 diabetes mellitus |
TG | Triglycerides |
IRS1 | Insulin receptor substrate 1 |
IR | Insulin resistance |
NAFLD | Non-alcoholic fatty liver disease |
LPS | Lipopolysaccharide |
HFD | High-fat diet |
ZO-1 | Zonulaoccludens-1 |
SCFAs | Short chain fatty acids |
GPR43 | G protein coupled receptor 43 |
GPR41 | G protein coupled receptor 41 |
PYY | Peptide YY |
GLP-1 | Gastrointestinal hormone glucagon-like peptide |
TC | Total cholesterol |
D-Lac |
D-Lactic acid |
IPGTT | Intraperitoneal injections of glucose tolerance test |
AUC | Area under curve |
IPITT | Intraperitoneal injections of insulin tolerance test |
Author contributions
Sicong Tian (first author): funding acquisition; experimental design, methodology, RCT, investigation, analysis, writing – original draft; Yiting Lei: RCT, writing; Fangling Zhao: animal experiment; Jiawen Che: RCT; Yanhong Wu: data analysis; Yea Eun Kang (corresponding author): review and editing; Yujuan Shan (corresponding author): funding acquisition, resources, supervision, writing – review and editing.
Conflicts of interest
The authors declare no conflict of interest.
Acknowledgements
This work was funded by the National Natural Science Foundation of China (No. 82073540, 82204034).
References
- Z. M. Younossi, P. Golabi, J. M. Paik, A. Henry, C. Van Dongen and L. Henry, The global epidemiology of nonalcoholic fatty liver disease (NAFLD) and nonalcoholic steatohepatitis (NASH): a systematic review, Hepatology, 2023, 77, 1335–1347 CrossRef PubMed.
- J. Zhou, F. Zhou, W. Wang, X. J. Zhang, Y. X. Ji and P. Zhang,
et al., Epidemiological Features of NAFLD From 1999 to 2018 in China, Hepatology, 2020, 71, 1851–1864 CrossRef PubMed.
- J. Weiß, M. Rau and A. Geier, Non-alcoholic fatty liver disease: epidemiology, clinical course, investigation, and treatment, Dtsch. Arztebl. Int., 2014, 111, 447–452 Search PubMed.
- Z. M. Younossi, A. B. Koenig, D. Abdelatif, Y. Fazel, L. Henry and M. Wymer, Global epidemiology of nonalcoholic fatty liver disease-Meta-analytic assessment of prevalence, incidence, and outcomes, Hepatology, 2016, 64, 73–84 CrossRef PubMed.
- G. Targher, K. E. Corey, C. D. Byrne and M. Roden, The complex link between NAFLD and type 2 diabetes mellitus - mechanisms and treatments, Nat. Rev. Gastroenterol. Hepatol., 2021, 18, 599–612 CrossRef PubMed.
- H. Fujii, M. Enomoto, W. Fukushima, A. Tamori, H. Sakaguchi and N. Kawada, Applicability of BARD score to Japanese patients with NAFLD, Gut, 2009, 58, 1566–1567 CrossRef CAS PubMed ; author reply 1567.
- T. Nakahara, H. Hyogo, M. Yoneda, Y. Sumida, Y. Eguchi and H. Fujii,
et al., Type 2 diabetes mellitus is associated with the fibrosis severity in patients with nonalcoholic fatty liver disease in a large retrospective cohort of Japanese patients, J. Gastroenterol., 2014, 49, 1477–1484 CrossRef CAS PubMed.
- R. Kwok, K. C. Choi, G. L. Wong, Y. Zhang, H. L. Chan and A. O. Luk,
et al., Screening diabetic patients for non-alcoholic fatty liver disease with controlled attenuation parameter and liver stiffness measurements: a prospective cohort study, Gut, 2016, 65, 1359–1368 CrossRef CAS PubMed.
- M. C. Petersen and G. I. Shulman, Mechanisms of Insulin Action and Insulin Resistance, Physiol. Rev., 2018, 98, 2133–2223 CrossRef CAS PubMed.
- G. I. Smith, M. Shankaran, M. Yoshino, G. G. Schweitzer, M. Chondronikola and J. W. Beals,
et al., Insulin resistance drives hepatic de novo lipogenesis in nonalcoholic fatty liver disease, J. Clin. Invest., 2020, 130, 1453–1460 CrossRef CAS PubMed.
- J. E. Lambert, M. A. Ramos-Roman, J. D. Browning and E. J. Parks, Increased de novo lipogenesis is a distinct characteristic of individuals with nonalcoholic fatty liver disease, Gastroenterology, 2014, 146, 726–735 CrossRef CAS PubMed.
- J. Chen and L. Vitetta, Gut Microbiota Metabolites in NAFLD Pathogenesis and Therapeutic Implications, Int. J. Mol. Sci., 2020, 21, 5214 CrossRef CAS PubMed.
- J. Tan, R. Hu, J. Gong, C. Fang, Y. Li and M. Liu,
et al., Protection against Metabolic Associated Fatty Liver Disease by Protocatechuic Acid, Gut Microbes, 2023, 15, 2238959 CrossRef PubMed.
- Y. Choi, S. I. Choi, N. Kim, R. H. Nam, J. Y. Jang and H. Y. Na,
et al., Effect of Clostridium butyricum on High-Fat Diet-Induced Intestinal Inflammation and Production of Short-Chain Fatty Acids, Dig. Dis Sci., 2023, 68, 2427–2440 CrossRef CAS PubMed.
- M. A. Neag, A. E. Craciun, A. I. Inceu, D. E. Burlacu, C. I. Craciun and A. D. Buzoianu, Short-Chain Fatty Acids as Bacterial Enterocytes and Therapeutic Target in Diabetes Mellitus Type 2, Biomedicines, 2022, 11, 72 CrossRef PubMed.
- N. Mohamadi, V. Baradaran Rahimi, M. R. Fadaei, F. Sharifi and V. R. Askari, A mechanistic overview of sulforaphane and its derivatives application in diabetes and its complications, Inflammopharmacology, 2023, 31, 2885–2899 CrossRef PubMed.
- Y. Xu, J. F. Fu, J. H. Chen, Z. W. Zhang, Z. Q. Zou and L. Y. Han,
et al., Sulforaphane ameliorates glucose intolerance in obese mice via the upregulation of the insulin signaling pathway, Food Funct., 2018, 9, 4695–4701 RSC.
- Y. Zhang, Q. Wu, J. Liu, Z. Zhang, X. Ma and Y. Zhang,
et al., Sulforaphane alleviates high fat diet-induced insulin resistance via AMPK/Nrf2/GPx4 axis, Biomed. Pharmacother., 2022, 152, 113273 CrossRef CAS PubMed.
- S. Tian, P. Lei, C. Teng, Y. Sun, X. Song and B. Li,
et al., Targeting PLIN2/PLIN5-PPARγ: Sulforaphane Disturbs the Maturation of Lipid Droplets, Mol. Nutr. Food Res., 2019, 63, e1900183 CrossRef PubMed.
- S. Tian, B. Li, P. Lei, X. Yang, X. Zhang and Y. Bao,
et al., Sulforaphane Improves Abnormal Lipid Metabolism via Both ERS-Dependent XBP1/ACC &SCD1 and ERS-Independent SREBP/FAS Pathways, Mol. Nutr. Food Res., 2018, 62, e1700737 CrossRef PubMed.
- S. Tian, P. Lei, J. Zhang, Y. Sun, B. Li and Y. Shan, Sulforaphane Balances Ca(2+) Homeostasis Injured by Excessive Fat via Mitochondria-Associated Membrane (MAM), Mol. Nutr. Food Res., 2021, 65, e2001076 CrossRef PubMed.
- M. Kikuchi, Y. Ushida, H. Shiozawa, R. Umeda, K. Tsuruya and Y. Aoki,
et al., Sulforaphane-rich broccoli sprout extract improves hepatic abnormalities in male subjects, World J. Gastroenterol., 2015, 21, 12457–12467 CrossRef CAS PubMed.
- C. He, B. Li, L. Huang, C. Teng, Y. Bao and M. Ren,
et al., Gut microbial composition changes in bladder cancer patients: A case-control study in Harbin, China, Asia Pac. J. Clin. Nutr., 2020, 29, 395–403 Search PubMed.
- D. R. Matthews, J. P. Hosker, A. S. Rudenski, B. A. Naylor, D. F. Treacher and R. C. Turner, Homeostasis model assessment: insulin resistance and beta-cell function from fasting plasma glucose and insulin concentrations in man, Diabetologia, 1985, 28, 412–419 CrossRef CAS PubMed.
- R. Alaaeldin, I. A. M. Abdel-Rahman, H. A. Hassan, N. Youssef, A. E. Allam and S. F. Abdelwahab,
et al., Carpachromene Ameliorates Insulin Resistance in HepG2 Cells via Modulating IR/IRS1/PI3k/Akt/GSK3/FoxO1 Pathway, Molecules, 2021, 26, 7629 CrossRef CAS PubMed.
- E. E. Canfora, R. C. R. Meex, K. Venema and E. E. Blaak, Gut microbial metabolites in obesity, NAFLD and T2DM, Nat. Rev. Endocrinol., 2019, 15, 261–273 CrossRef CAS PubMed.
- M. K. Thomas, A. Nikooienejad, R. Bray, X. Cui, J. Wilson and K. Duffin,
et al., Dual GIP and GLP-1 Receptor Agonist Tirzepatide Improves Beta-cell Function and Insulin Sensitivity in Type 2 Diabetes, J. Clin. Endocrinol. Metab., 2021, 106, 388–396 CrossRef PubMed.
- M. Salehi, B. Aulinger, R. L. Prigeon and D. A. D'Alessio, Effect of endogenous GLP-1 on insulin secretion in type 2 diabetes, Diabetes, 2010, 59, 1330–1337 CrossRef CAS PubMed.
- Y. Yao, L. Yan, H. Chen, N. Wu, W. Wang and D. Wang, Cyclocarya paliurus polysaccharides alleviate type 2 diabetic symptoms by modulating gut microbiota and short-chain fatty acids, Phytomedicine, 2020, 77, 153268 CrossRef CAS PubMed.
- C. Guo, T. Huang, A. Chen, X. Chen, L. Wang and F. Shen,
et al., Glucagon-like peptide 1 improves insulin resistance in vitro through anti-inflammation of macrophages, Braz. J. Med. Biol. Res., 2016, 49, e5826 CrossRef CAS PubMed.
- L. Subedi, J. H. Lee, S. Yumnam, E. Ji and S. Y. Kim, Anti-Inflammatory Effect of Sulforaphane on LPS-Activated Microglia Potentially through JNK/AP-1/NF-κB Inhibition and Nrf2/HO-1 Activation, Cells, 2019, 8, 194 CrossRef CAS PubMed.
- W. Tan, L. Mao, S. Yu, J. Huang, Q. Xie and M. Hu,
et al., DHA and EPA improve liver IR in HFD-induced IR mice through modulating the gut microbiotas-LPS-liver axis, J. Funct. Foods, 2024, 112, 105917 CrossRef CAS.
- J. du Plessis, H. Korf, J. van Pelt, P. Windmolders, I. Vander Elst and A. Verrijken,
et al., Pro-Inflammatory Cytokines but Not Endotoxin-Related Parameters Associate with Disease Severity in Patients with NAFLD, PLoS One, 2016, 11, e0166048 CrossRef PubMed.
- P. Tian, B. Li, C. He, W. Song, A. Hou and S. Tian,
et al., Antidiabetic (type 2) effects of Lactobacillus, G15 and Q14 in rats through regulation of intestinal permeability and microbiota, Food Funct., 2016, 7, 3789–3797 RSC.
- E. V. Prochownik and H. Wang, The Metabolic Fates of Pyruvate in Normal and Neoplastic Cells, Cells, 2021, 10, 762 CrossRef CAS PubMed.
- J. R. Roy, C. S. Janaki, S. Jayaraman, V. P. Veeraraghavan, V. Periyasamy and T. Balaji,
et al., Hypoglycemic Potential of Carica papaya in Liver Is Mediated through IRS-2/PI3K/SREBP-1c/GLUT2 Signaling in High-Fat-Diet-Induced Type-2 Diabetic Male Rats, Toxics, 2023, 11, 240 CrossRef CAS PubMed.
- A. Y. Sim, S. Barua, J. Y. Kim, Y. H. Lee and J. E. Lee, Role of DPP-4 and SGLT2 Inhibitors Connected to Alzheimer Disease in Type 2 Diabetes Mellitus, Front. Neurosci., 2021, 15, 708547 CrossRef PubMed.
|
This journal is © The Royal Society of Chemistry 2024 |