DOI:
10.1039/D4FO00210E
(Paper)
Food Funct., 2024,
15, 8661-8673
A high-fat diet induced depression-like phenotype via hypocretin-HCRTR1 mediated inflammation activation†
Received
13th January 2024
, Accepted 8th July 2024
First published on 26th July 2024
Abstract
Background: A high-fat diet (HFD) is generally associated with an increased risk of mental disorders that constitute a sizeable worldwide health. A HFD results in the gut microbiota–brain axis being altered and linked to mental disorders. Hypocretin-1, which can promote appetite, has been previously confirmed to be associated with depression. However, no exact relationship has been found for hypocretin between depression and HFDs. Methods: Adult male SD rats were randomly assigned to either a HFD or a normal diet for eight weeks, followed by behavioral tests and plasma biochemical analyses. Then, we investigated the protein and mRNA levels of inflammation-related factors in the hippocampus. We also observed morphological changes in brain microglia and lipid accumulation. Additionally, metagenomic and metabolomic analyses of gut microbiomes were performed. 3T3-L1 cells were utilized in vitro to investigate the impact of hypocretin receptor 1 antagonists (SB334867) on lipid accumulation. To consider the connection between the brain and adipose tissue, we used a conditioned medium (CM) treated with 3T3-L1 cells to observe the activation and phagocytosis of BV2 cells. Following a 12-week period of feeding a HFD to C57BL/6 mice, a three-week intervention period was initiated during which the administration of SB334867 was observed. This was followed by a series of assessments, including monitoring of body weight changes and emotional problems, as well as attention to plasma biochemical levels and microglial cell phenotypes in the brain. Results: The HFD rats displayed anxiety and depressive-like behaviors. HFD rats exhibited increased plasma HDL, LDL, and TC levels. A HFD also causes an increase in hypocretin-1 and hypocretin-2 in the hypothalamus. Metagenomics and metabolomics revealed that the HFD caused an increase in the relative abundance of associated inflammatory bacteria and decreased the abundance of anti-inflammatory and bile acid metabolites. Compared with the CTR group, hippocampal microglia in the HFD group were significantly activated and accompanied by lipid deposition. At the same time, protein and mRNA expression levels of inflammation-related factors were increased. We found that SB334867 could significantly reduce lipid accumulation in 3T3-L1 cells after differentiation. The expression of inflammatory factors decreased in the SB334867 group. The administration of SB334867 was found to reverse the adverse effects of the HFD on body weight, depressive-like behaviour and anxiety-like mood. Furthermore, this treatment was associated with improvements in plasma biochemical levels and a reduction in the number of microglia in the brain. Conclusions: In summary, our results demonstrated that a HFD induced anxiety and depressive-like behaviors, which may be linked to the increased hypocretin-1 level and lipid accumulation. Supplementation with SB334867 improved the above. These observations highlight the possibility of hypocretin-1 inducing the risk of HFD-associated emotional dysfunctions.
1. Introduction
An excessive high-fat diet (HFD) often leads to several physical problems. A large amount of clinical data shows that a HFD can impair cognitive function, including memory and attention, and more importantly, a HFD may increase the likelihood of depression and anxiety.1 A HFD causes an increase in peripheral inflammatory factors and affects the central nervous system, leading to an inflammatory response. Notably, a HFD has been linked to depression associated with increased CORT, low-grade inflammation, neuronal atrophy, reduced hippocampal neurogenesis, and reduced neurotrophin expression in humans and rats.2,3
The gastrointestinal tract of humans or animals harbors a complex and diverse microbial community that significantly influences the host's metabolism, physiology, and immune function.4,5 Changes in the gut microbiome are closely related to changes in physical conditions (such as a HFD), which may lead to intestinal microecological disorders. Similarly, gut microbiome disorders will accelerate the process of a HFD.6 The brain and gut are interconnected and communicate bidirectionally through neural, immune, and endocrine pathways and the hypothalamic–pituitary–adrenal (HPA) stress axis.7–10 Therefore, gut microbiome disorders can have a significant impact on overall health. The HPA axis is pivotal in mediating stress responses and interactions between the brain and gut.11 Recent studies have shown that the gut microbiota of HFD mice transplanted to a healthy one can cause psychiatric disorders and increase neuroinflammation.12 However, the detailed mechanism of how a HFD affects the brain is still not precise, and its procedures are not yet fully understood.
The gut microbiota and its by-products have a direct neuroimmunomodulatory impact on the CNS. Microbiome-dependent signaling regulates the development, maturation, and function of microglia and astrocytes. Under homeostatic conditions, microglia retain a branching morphology and sustain a continuous brain environment of homeostasis. However, perturbations like a HFD cause microglia to undergo rapid shifts.13,14 Recent studies on macrophages have stimulated increased interest in lipid processing in microglia. Lipid storage in microglia is thought to be associated with changes in microglia phenotypes in neuroinflammatory environments.
The hypothalamus controls energy metabolism, homeostasis, food intake, and weight regulation across the body.15 Previous research shows that the polypeptide hypocretin in the hypothalamus regulates food consumption and body mass.16 Injecting hypocretin in the hypothalamus causes an increase in food intake.17 Studies have revealed that hypocretin and its receptors are also present in the pancreas and intestines,18 indicating that hypocretin contributes to regulating energy homeostasis and eating habits within these organs. Consequently, we hypothesize that a HFD influences the abundance of the gut microbiome and promotes hypocretin secretion, which promotes fat storage and ultimately activates microglia, increasing the inflammation of the brain and resulting in depression.
In this study, we investigated the impact of a HFD on mental disorders and brain inflammation, as well as the changes in the gut microbiome. Additionally, we used 3T3-L1 cells and BV2 cells as models to explore the effects of hypocretin-1 and hypocretin receptor 1 antagonists (SB334867) on lipid accumulation and changes in related inflammatory factors that may contribute to depression.
The current study describes the role of hypocretin-1 in HFD-mediated depressive and anxiety-like behaviours. A HFD diet led to elevated hypothalamus hypocretin levels and changes in gut flora, and notably, the mood disorders and intracerebral inflammation associated with the HFD were ameliorated after administration of SB334867. This may be achieved by modulating the inflammatory response, which was verified in vitro in cells. It is conceivable that SB334867 may be capable of improving depression and anxiety-like behaviour and systemic inflammatory responses caused by the HFD diet. It is possible that hypocretin-1 may be the trigger of affective disorders caused by the HFD.
2. Methods
2.1 Animals
Male Sprague–Dawley (SD) rats (220–240 g) were housed in a standard environment and maintained under a 12
:
12 h light/dark cycle. Food and water were provided ad libitum. After a week, rats were randomly assigned into two groups, one fed with a normal diet and another with HFD. The CTR group rats were fed a normal diet, while the HFD group was fed a diet of HFD pellets (TP23300, Trophic Animal Feed High-Tech Co., Ltd), and provided 5.0 kcal g−1 energy with 60% fat, 20.6% carbohydrates, and 19.4% protein for eight weeks.
Male C57BL/6 mice (20–22 g) were housed in a standard environment and maintained under a 12
:
12 h light/dark cycle. Food and water were provided ad libitum. After a week, mice were randomly assigned into two groups, one fed with a normal diet and another with HFD. The CTR group mice were fed a normal diet, while the HFD group was fed a diet of HFD pellets (TP23300, Trophic Animal Feed High-Tech Co., Ltd), and provided 5.0 kcal g−1 energy with 60% fat, 20.6% carbohydrates, and 19.4% protein for eight weeks. Following a 12-week period on a high-fat diet (HFD), mice were randomly assigned to two groups. One group received daily intraperitoneal injections of SB334867 (5 mg kg−1), while the other group was injected with the solvent, for three weeks. Subsequently, both groups underwent behavioral experiments and tissue collection. All animal works were performed according to the guidelines approved by the Animal Experimentation Ethics Committee of the First Affiliated Hospital, Zhejiang University School of Medicine (Reference Number: 2022-1221)
2.2 Behavior tests
Before every behavior test, animals were put in the test room for at least 2 h to adapt to the environment.
2.3 Open field test (OFT)
The OFT was conducted to observe the spontaneous exploration of motor activity and anxiety behavior in rats. The rat was put in the center of the open field (a box divided into 16 squares in the bottom) for 5 min in a quiet room. The time and distance the rat traveled in the center of four squares were recorded.
2.4 Elevated plus maze (EPM) test
The purpose of the elevated plus-maze is to study the anxiety state of animals by using the contradictory behavior of exploration of the new environment and fear of open arms. The rat was placed in the center of the maze with its head facing the open arm for 5 min in a quiet room. The time and distance traveled by the rat in the open arm were recorded.
2.5 Y maze test
The Y maze is a three-equivalent radial maze consisting of three arms and a connecting area. The experiment is divided into an adaptation phase and an experimental phase. Adaptation phase: the new arm was blocked with a spacer and the rat was placed in the starting arm, allowing it to explore the starting arm and the old arm freely for 5 min; experimental phase: the spacer was removed and the rat was placed in the starting arm, and the time the rat took to pass the old arm and new arm in 5 min was observed.
2.6 Novel object recognition (NOR) test
The experiment is divided into an adaptation phase and an experimental phase. Adaptation phase: two identical objects were placed in the field, and the rats were placed into the field with their backs facing the two objects, training for 5 min. After the rats had rested for 1 hour, the experimental phase was conducted. Experimental phase: one object in the field was replaced with a new object, and the other remained unchanged; the rats were still placed into the field with their backs facing the two objects, and the number of times the rats touched the new object and the times they touched the two objects were recorded.
2.7 Tissue collection
The day after all behavior tests, animals were deeply anesthetized with pentobarbital. Normal saline was perfused through the heart, followed by an infusion of 4% PFA. The brain was dissected and fixed in 4% PFA for over 24 hours. Following dehydration and embedding of the brain, 4 μm paraffin sections and 40 μm frozen sections were prepared. Then, blood was collected by cardiac puncture; tissue samples were stored at −80 °C for further studies.
2.8 Enzyme-linked immunosorbent assay
Blood was centrifuged at 3000 rpm for 10 min at room temperature and stored at −80 °C until use. Serum levels of high-density lipoprotein (HDL), low-density lipoprotein (LDL), total cholesterol (TC), and triglyceride (TG) were assessed using mouse-specific kits purchased from Nanjing Jiancheng Bioengineering Institute (Nanjing, China). Hypocretin-1 and hypocretin-2 content in the hypothalamus were determined using the kits purchased from Novus Biologicals after homogenization.
2.9 Cell culture
3T3-L1 fibroblast cells and BV2 cells purchased from Procell Life Science & Technology Co., Ltd (Wuhan, China) were maintained in a standard growth medium (DMEM with 10% fetal bovine serum (BDBio, China), 1% penicillin, and 1% streptomycin) at 37 °C under a humidified atmosphere (5% CO2, 95% O2).
2.10 Differentiation procedure
3T3-L1 cells were planted in a Petri dish and fused for two days, and then the medium containing 0.5 mmol IBMX, 1 μmol DEX, and 10 μg mL−1 insulin was incubated for two days, and then the medium was changed with only 10 μg mL−1 insulin for another two days, and finally the complete medium set was changed in the last two days.
2.11 Oil red O staining
Lipid accumulation was observed through oil-red O staining. The differentiated 3T3-L1 cells were washed with PBS and fixed with 4% PFA for 20 min. After fixing the cells, the cells were washed with PBS 3 times and dried. The saturated oil red O dye solution was diluted with water at a ratio of 5
:
2 and then filtered for dyeing. After dyeing for 10 min, the excess dye was washed off with water. Images were obtained by using an Olympus IX73 (Tokyo, Japan) at ×20 magnification. For an intuitive quantification of lipid accumulation, the stain was eluted with isopropyl alcohol and observed at 500 nm by using a microcode.
2.12 3T3-L1 conditioned medium (CM)
3T3-L1 cells were planted in a 24-well plate and differentiated. The cells were pretreated with hypocretin-1 (200 nmol, MedChemExpression, USA) or HCRTR1 antagonist (SB334867, 10 μM, Selleck, USA) for 48 h in a serum-free culture medium. Considering the hypocretin-1 physiological level, the dose of hypocretin-1 was referred from a research study16 and a gradient cell viability test was also performed to ensure the reliability of the drug dose. After incubation, the supernatant was collected and filtered with a 0.45 μm sterile filter to remove cells and cell debris. For the control group, 3T3-L1 cells were incubated with a medium only for 24 h.
2.13 Western blots
The homogenized rat hippocampus tissue and cells were lysed by using a lysis buffer mixed with a protease inhibitor on ice for 20 min, and were then centrifuged at 12
000 rpm for 10 min to obtain a protein solution. The protein concentration was determined by using a BCA kit (Beyotime, China). All samples were mixed with a loading buffer and heated at 95 °C for 15 min. SDS-PAGE polyacrylamide gel electrophoresis was used to separate the proteins of different molecular weights. The proteins were transferred to a PVDF membrane, sealed with BSA for 1 hour, and washed three times with TBST for 5 min each time. Then, it was incubated with primary antibodies at 4 °C overnight. On the second day, they were washed with TBST for 5 min three times, and then incubated with goat anti-mouse/rabbit (Proteintech, 1
:
10
000) at room temperature for 1 hour, and finally washed with TBST three times for five min each time. Supersensitive ECL luminescence solution was used for development, and bands were uniformly analyzed and normalized using ImageJ. The following anti-bodies were used: SYP (Proteintech, 1
:
20
000) and β-actin (Proteintech, 1
:
2000).
2.14 Quantitative real-time PCR
According to the manufacturer's instructions, the total RNA was extracted from rat hippocampus tissue and cells using the FastPure Cell/Tissue Total RNA Isolation Kit (Nanjing, China). Following this, 1 μg of total RNA was transcribed reversely using the Vazyme PrimeScript™ RT Master Mix reverse transcription kit (Nanjing, China). The quantitative analysis of mRNA expression was carried out via RT-qPCR. It utilized the ABclonal 2X Universal SYBR Green Fast qPCR Mix (Wuhan, China) and the CFX384TM real-time system (Hercules, CA). Cycle threshold (Ct) values were recorded and normalized to β-actin, and the relative gene expression was calculated by the 2−ΔΔCt method. Primers were designed by NCBI Primer designing tool (https://www.ncbi.nlm.nih.gov/tools/primer-blast/).
2.15 Immunohistochemistry
Dewaxing paraffin sections to water, xylene for 10 min twice and gradient alcohol (anhydrous, 95%, 75%, 65%, 50%) 5 min per cylinder, PBST washing 3 times, for 3 min each time. Antigen repair: pH = 6.0 citric acid repair solution was added, and microwave 800w heating was applied for 30 min; after the repair solution was cooled to room temperature (25–30 °C) (about 1 h), the slices were removed from the repair solution, and washed in PBST (5 min × 3 times). 3% skim milk powder was incubated at room temperature for 1 h. The IBA1 antibody (1
:
2000 Abcam, ab178846) was diluted with 3% BSA and set with sections at 4 °C overnight. Slices were washed 3 × 5 min, and each section was incubated with goat anti-rabbit IgG-HRP at room temperature for 1 h, and washed with PBST 3 × 5 min. 50 μl DAB was added to each slice, and tap water was used to terminate the reaction. Hematoxylin was used for staining for 1 min and they were soaked, and hydrochloric acid and alcohol were used for differentiating the slices for 1–2 seconds, and they were washed, and a reverting blue solution was used for rinsing the slices for several seconds.
2.16 Immunofluorescence
For paraffin sections: The sections were dewaxed to water: using xylene for 10 min twice and then gradient alcohol (anhydrous, 95%, 75%, 65%, 50%) for 5 min. And the sections were washed with PBS 3 × 5 min. Antigen repair: pH = 6.0 citric acid repair solution was added, and subjected to microwaves at 800w for 30 min, and kept until the repair solution naturally cooled down to room temperature in about 1 h, and then washed with PBS 3 × 5 min. Sections were incubated with 3% skim milk powder for 1 h to block. Antibodies were diluted with 3% BSA and set at 4 °C overnight. Sections were rinsed three times with PBS for 3 × 5 min, and each section was incubated dropwise with goat anti-rabbit CY3 for 1 h at room temperature and washed three times with PBS for five mins.
For frozen sections, the sections were rinsed three times with PBS for five minutes each. Then, the sections were incubated with 3% skim milk powder for 1 h to block. After three washes, the sections were incubated with an antibody (IBA1, 1
:
300, Abcam) at 4 °C overnight, and the sections were rinsed three times with PBS for five minutes each, and each section was incubated dropwise with goat anti-rabbit IgG-HRP for one hour at room temperature. If necessary, the slices were incubated with a BODIPY493/503 (10 μmol, MCE, USA) lipid dye at room temperature for 30 min. Following this, the sections were washed three times with PBS for five minutes each. If multiple markers were required, the instructions for the tyramine signal amplification (TSA) method SuperBoost kit should be followed (Thermo Fisher Scientific, b40957). Sections were sealed with an anti-quenching sealer containing DAPI and visualized using a laser scanning confocal microscope (FV3000, Olympus, Japan).
2.17 Immunocytochemistry
3T3-L1 and BV2 cells were planted on cell slices in 24-well plates, washed with PBS for 5 min 3 times, and fixed with 4% PFA for 15 min. Then, the slices were rinsed with PBS and incubated with 0.1% Triton X-100 in PBS for 15 min, followed by 1% BSA in PBS at 37 °C for 30 min. Then, the sections were incubated with a primary antibody at 4 °C overnight. The next day, section incubation was performed with an Alexa-Fluor-conjugated secondary antibody (Proteintech, China) at room temperature for 1 h. If necessary, the slices were incubated with a BODIPY 493/503(10 μmol, MCE, USA) lipid dye at room temperature for 30 min, and then the sections were sealed with an antifading mounting medium (with DAPI) (Solarbio, China); the fluorescence images were captured with FV3000. ImageJ was used to analyze the fluorescence intensity. The following antibodies were used: α-tubulin (Proteintech, 1
:
1000) and IBA1 (Abcam, 1
:
300).
2.18 Hematoxylin–eosin staining
Paraffin sections were deparaffinized in xylene for 2 × 10 min and gradient alcohol (100%, 95%, 75%) for 5 min per vat, transferred to hematoxylin, dipped in hematoxylin for 10 min, and washed with water to remove hematoxylin, and then, subjected to 1% hydrochloric acid in alcohol for 30 s, and washed with running water for 30 min, dipped in eosin for 5 min, washed with water to remove eosin floats, and wiped with gauze to remove any excess dye from the slides. The water on the slides was absorbed and placed in graded alcohol (80%, 90% and 100%) for 5 min per vat, and made transparent in xylene 2 times, 5 min each time.
2.19 Luminex liquid suspension chip assay
Inflammatory factors were detected by a Luminex suspension chip assay supplied by Wayen Biotechnologies (Shanghai, China). Samples were obtained from the hippocampus, and samples were lysed and centrifuged at 10
000 rpm for 10 min. The standard and sample were added into plates embedded with microbeads and incubated for 30 minutes. After that, the detection antibody was added into wells and set for 30 minutes. Then, streptavidin-PE was added to each well. After 10 min incubation, the signal in each well was measured by using a Bio-Plex MAGPIX System (Bio-Rad; Canada).
2.20 Metagenomics
To evaluate the impact of an HFD on gut microbiota, metagenomic sequencing was carried out on rat fecal samples to extract the gut microbiota DNA. Quality-checked DNA samples were utilized to create a metagenomic shotgun sequencing library using the Enzymic Universal DNAseq Library Prep Kit. The resulting library is designed to be used in various applications in scientific research.
2.21 Non-targeted metabolomics
To assess the impact of HFD on intestinal flora metabolites, a non-targeted metabolomic analysis was carried out using LC-MS/MS on rats in the control and high-fat groups. Rat stool samples were weighed and mixed with 100 μl of pre-cooled ultra-pure water, followed by 400 μl of pre-cooled methanol acetonitrile solution (1
:
1, v/v) after 60 seconds of vortexing. The supernatant was centrifuged at 12
000 revolutions per minute for 20 min. Subsequently, it was redissolved in 200 microliters of 30% ACN through vacuum drying, followed by swirling and centrifugation at 14
000g at 4 °C for 15 min. The sample was injected into an LC-MS/MS system for analytical testing. To ensure accuracy and reliability in the results, quality control (QC) examples were included in the queue. The identification of metabolites relied on publicly available metabolomics and self-constructed databases, with mass and secondary spectral information employed. LC-MS/MS analyses were performed using an AB SciexQTRAP® 6500 + (SCIEX, USA) by Kaitaibio Co., Ltd (Hangzhou, China).
2.22 Statistical analysis
All the data were analyzed by using GraphPad Prism 9; ANOVA was used to analyz the data of more than two groups followed by Dunnett's test for post-hoc comparisons. And the data of two groups were analyzed by a T-test. Spearman correlation index was used to test the correlation between the two groups. All data were within the 95% confidence interval, and p < 0.05 was considered a significant difference.
3. Results
3.1 HFD induces alterations in serum biochemistry parameters
After 8 weeks of a HFD, HDL, LDL, TC, and TG levels in plasma were measured. The results showed that the contents of HDL, LDL, and TC in the plasma of the HFD group were significantly increased (Fig. 2b–d, n = 8); TG had no significant difference (Fig. 2e, n = 8), indicating that a HFD may damage lipid metabolism.
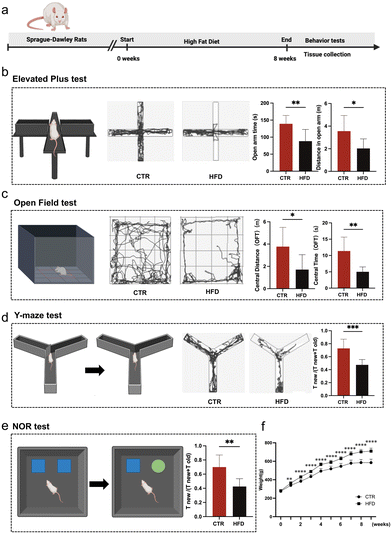 |
| Fig. 1 (a) This is a schematic diagram illustrating the construction of the HFD rat model. The representative road map of the CTR and HFD groups follows the open field test model figure. (b) The pattern diagram showcases the elevated plus maze test and the path taken by the HFD and CTR groups. The results showed that the movement distance and residence time of HFD rats in the open arm were significantly smaller than that of the CTR group (n = 8). (c) The open field model diagram and the representative road map of the CTR and HFD groups. The results indicate that the centre time and centre distance of the HFD group were significantly smaller than those of the CTR group (n = 8). (d) In the Y-maze test, the Y-maze setup is shown in the training and experimental phases, respectively, with the training phase blocking an arm. The proportion of time the HFD group spent in the new arm was lower than that of the CTR group (n = 8). (e) During the novel object test, it was observed that the HFD group spent less time around the new object than the control group (n = 8). (f) Additionally, the line chart depicting the body weight record during model establishment demonstrated a faster increase in the HFD group compared to the CTR group. A significant difference in body weight between the two groups was also observed (n = 8). Statistical analysis using Student's two-tailed t-test showed no significant difference between groups. *p < 0.05, **p < 0.01, ***p < 0.001, ****p < 0.0001. | |
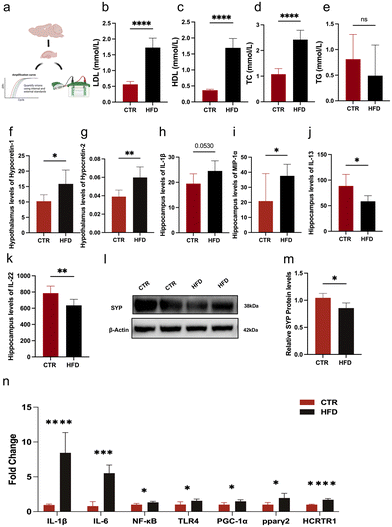 |
| Fig. 2 Effects of high fat diet on plasma biochemical levels and hippocampus. (a) Schematic diagram of the experiments related to the hippocampus. (b) Determination of plasma low-density lipoprotein (LDL) level: high fat diet (HFD) significantly increased plasma LDL levels in rats (n = 8). (c) Determination of plasma high-density lipoprotein (HDL) level: HFD significantly increased plasma HDL levels in rats (n = 8). (d) Determination of plasma total cholesterol (TC) level: HFD significantly increased plasma TC levels in rats (n = 8). (e) Determination of plasma triglyceride (TG) level. No significant difference was found between the HFD and CTR groups (n = 8). (f) The hypocretin-1 content in the hypothalamus was determined, and it was found that the HFD diet significantly increased the level of hypocretin-1 in the hypothalamus of rats (n = 6). (g) Additionally, the content of hypocretin-2 in the hypothalamus was significantly higher in the HFD group (n = 6). (h and i) The protein levels of IL-1β and MIP-1α in the hippocampus of rats were also determined. It was found that the HFD diet increased the levels of IL-1β and MIP-1α proteins (n = 6). (j and k) When examining the protein levels of IL-13 and IL-22 in the hippocampus of rats, it was found that HFD reduced their contents (n = 6). (l and m) Western blotting was utilised to demonstrate the effect of HFD on the protein levels of SYP. SYP indicated that HFD was detrimental to synaptic plasticity (n = 5). (n) The fold change of mRNA levels of related inflammatory factors and hypocretin receptor 1 (HCRTR1) in the hippocampus of rats underwent fold changes. Data are presented as mean ± SD. Significance levels were denoted as follows: statistical analysis using Student's two-tailed t-test showed no significant difference between groups (ns). *p < 0.05, **p < 0.01, ***p < 0.001, ****p < 0.0001. | |
3.2 HFD rats exhibit anxiety and depressive-like behaviors
To investigate the influence of HFD intake on emotional functions, we performed OFT, Y-maze, elevated plus-maze, and NOR tests. In the OFT, the number and time of entering the center squares were significantly reduced compared with those in the CTR group (Fig. 1c, n = 8). Similarly, in elevated plus-maze tests, the distance and time of entering the open arm of the HFD group were significantly shortened compared with the CTR group (Fig. 1b, n = 8). These tests proved that HFD caused anxiety and depressive-like behavior in rats. The Y-maze and NOR tests were used to evaluate animals’ learning and memory abilities. The Y-maze test showed that the time to enter new arms in Y-maze decreased significantly compared with the CTR group (Fig. 1d, n = 8). The NOR test showed that the HFD group touched new objects at a considerably lower time than old things, indicating that the HFD group had lower learning and memory ability than the CTR group (Fig. 1e, n = 8). Behavior tests showed that HFD rats showed significant anxiety and depressive-like behaviors and a decline in learning and memory ability. Western blotting also showed that HFD significantly decreased synaptic plasticity-related proteins and SYP protein levels19 (Fig. 2m, n = 5).
3.3 HFD affected the expression of inflammatory-related targets in the hippocampus
HFD can cause chronic inflammation in the whole body. Inflammation in the hippocampus can lead to mental disorders, including depression and anxiety.20 Luminex suspension chip detection found that IL-1β and MIP1 protein levels increased (Fig. 2h and i, n = 6), and HFD caused a significant decrease in IL-13 and IL-22 levels (Fig. 2j and k, n = 6).21 The change of other relative factors is shown in Table 1.
Table 1 The content of hippocampal inflammatory factors
Protein name |
Mean ± standard deviation (pg mL−1) |
F value |
p value |
CTR |
HFD |
Abbreviations: CTR, control; HFD, high fat diet; IL-1α, interleukin 1 alpha; IL-4, interleukin 4; IL-5, interleukin 5; IL-6, interleukin 6; IL-7, interleukin 7; IL-10, interleukin 10; IL-12, interleukin 12; IL-17A, interleukin 17A; G-CSF, granulocyte colony-stimulating factor; GM-CSF, granulocyte-macrophage colony stimulating factor; GRO-α, growth-regulated α protein; IFN-γ, interferon gamma; M-CSF, colony stimulating factor 1; MCP-1, monocyte chemoattractant protein-1; MIP-3α, macrophage inflammatory protein-3 alpha; RANTES, regulated upon activation, normal T cell expressed, and secreted; TNF-α, tumour necrosis factor alpha; VEGF, vascular endothelial growth factor. |
IL-1α |
19.16 ± 5.06 |
18.59 ± 5.44 |
2.009 |
0.18 |
IL-4 |
17.6 ± 5.01 |
17.7 ± 5.59 |
4.501 |
0.47 |
IL-5 |
71.24 ± 12.32 |
70.2 ± 13.48 |
1.041 |
0.10 |
IL-6 |
243.6 ± 75.21 |
232.89 ± 78.81 |
3.606 |
0.17 |
IL-7 |
21.06 ± 5.32 |
19.18 ± 2.95 |
5.564 |
0.56 |
IL-10 |
25.16 ± 7.02 |
23.27 ± 5.91 |
2.985 |
0.97 |
IL-12 |
21.04 ± 4.01 |
20.23 ± 3.89 |
1.071 |
0.09 |
IL-17A |
24.89 ± 2.63 |
24.57 ± 2.8 |
1.243 |
0.31 |
G-CSF |
2.81 ± 0.28 |
2.73 ± 0.2 |
1.197 |
0.05 |
GM-CSF |
28.96 ± 5.99 |
26.89 ± 3.56 |
5.568 |
0.21 |
GRO-α |
17.19 ± 11.52 |
18.73 ± 12.17 |
12.56 |
0.55 |
IFN-γ |
141.25 ± 39.94 |
134.71 ± 40.89 |
2.518 |
0.13 |
M-CSF |
10.97 ± 1.45 |
10.73 ± 1.48 |
2.19 |
0.21 |
MCP-1 |
57.94 ± 24.21 |
58.19 ± 27.06 |
16.96 |
0.32 |
MIP-3α |
1.95 ± 0.5 |
1.89 ± 0.53 |
1.475 |
0.43 |
RANTES |
14.17 ± 2.58 |
14.24 ± 2.88 |
1.207 |
0.21 |
TNF-α |
197.53 ± 35.77 |
190.66 ± 35.3 |
4.826 |
0.15 |
VEGF |
8.88 ± 1.7 |
8.37 ± 1.3 |
2.698 |
0.64 |
Therefore, RT-qPCR was used to measure the mRNA expression levels of related inflammatory factors in the hippocampus of rats, including IL-1β, IL-6, NF-κB, TLR4, and other associated genes. As shown in Fig. 2n (n = 5), the expression of inflammation-related factors in the hippocampus is significantly increased.
3.4 HFD changed the levels of hypocretin-1 and hypocretin-2 in the hypothalamus and hippocampus
Previous studies have shown that hypocretin is a central system that regulates energy metabolism and food intake.22 We examined changes in hypothalamic hypocretin-1 and hypocretin-2 levels and significant increases in hypocretin-1 and hypocretin-2 levels in the HFD group (Fig. 2f and g, n = 6). HFD increased the mRNA levels of hypocretin receptor 1 in the hippocampus (Fig. 2n, n = 5).
3.5 Changes in the gut microbiome are correlated with a HFD
Alpha diversity, a prevalent measure of species diversity in community ecology, was used. The Shannon index was selected to observe bacterial diversity. We noted a decrease in bacterial diversity in HFD rats compared to those in CTR rats. Analyzing β diversity, we employed principal coordinate analysis (PCoA; see Fig. 3a, n = 8) to assess the similarity between species communities. We observed significant differences in bacterial communities between the CTR and HFD groups. At the phylum level, we found that23 Firmicutes had increased in abundance and Bacteroides had decreased, like the previous effects of HFD on gut microbiota (Fig. 3d, n = 8). At the genus level, inflammation-associated bacteria, such as Candidatus_Woykebacteria_noname, increased in response to the HFD.
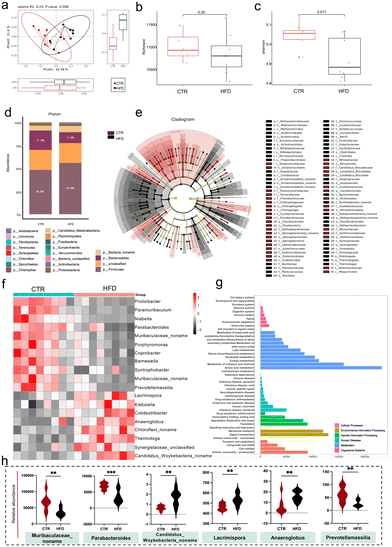 |
| Fig. 3 Alterations in the gut microbiota induced by a HFD were observed. (a) PCoA analysis showed significant differences in the gut microbiota of rats in the HFD and CTR groups (n = 8). (b) The gut microbiome enrichment demonstrated no significant difference between the HFD and CTR groups (n = 8). (c) Nevertheless, Shannon's analysis revealed that gut microbial diversity was significantly lower in rats fed with an HFD (n = 8). (d) The relative abundance of microbes was evaluated at the phylum level. (e) Based on the LEfSe analysis, the characteristic flora of the gut microbiota was found to differ between the HFD and CTR groups. (f) After a HFD diet, changes were observed in the HFD-related microbiota at the genus level. (g) Changes in the gut microbiome resulted in alterations in KEGG pathways. (h) Lastly, a violin plot was used to display the relative abundance of the associated differential gut microbiome. Data are presented as mean ± SD. Student's two-tailed t-test, *p < 0.05, **p < 0.01, ***p < 0.001, ****p < 0.0001. | |
In contrast, those related to diabetes metabolism, such as Lacrimispora, were also enriched in HFD rats. These findings were consistent with previous research.24–27 The consumption of HFD resulted in the downregulation of beneficial flora, such as Muribaculaceae_noname, Prevotellamassilia, and Parabacteroides (Fig. 3d, f and h, n = 8). Linear discriminant analysis (LDA) effect size (LEfSe) was utilized to analyze the various bacterial populations in each group (Fig. 3e, n = 8). Pearson's correlation analysis showed that the abundance of the relative gut microbiome was significantly associated with hippocampal pro-inflammatory cytokine levels (Fig. 4f, n = 6). The outcomes imply that the HFD results in an imbalance in the gut microbiome.
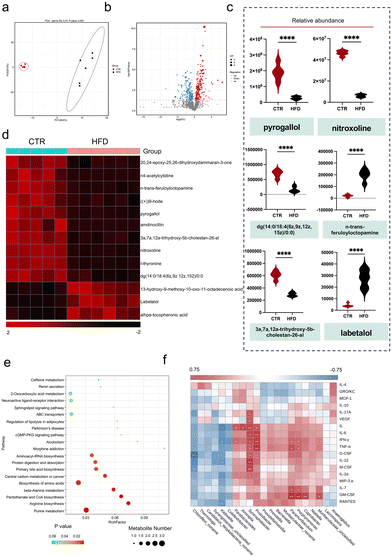 |
| Fig. 4 Changes in the gut microbial characteristics revealed by metabolomics. (a) PCA plot showed a large diversity of metabolites in the HFD and CTR groups (CTR = 5, HFD = 6). (b) The volcano plot indicates differential metabolites between rats in the HFD and CTR groups (CTR = 5, HFD = 6). (c) Violin plot depicting the relative abundance of related metabolites (CTR = 5, HFD = 6). (d) HFD and CTR differential metabolites shown by using a heat map (CTR = 5, HFD = 6). (e) HFD-induced metabolite changes affecting signalling pathways (CTR = 5, HFD = 6). (f) The Pearson correlation inflammatory cytokines and differential microbiota (n = 6). Student's two-tailed t-test, *p < 0.05, **p < 0.01, ***p < 0.001, ****p < 0.0001. | |
3.6 Effects of a HFD on gut microbiome metabolites
To better understand the triggering factors for effects induced by gut microbiota from a HFD, we conducted non-targeted metabolomic analyses of cecal contents from CTR and HFD group rats. Principal component analysis (PCA) was employed to observe the overall distribution of each group of samples and the stability of the entire analysis process (Fig. 4a, CTR = 5, HFD = 6). As demonstrated in Fig. 4b (CTR = 5, HFD = 6), CTR and HFD samples exhibited relatively dispersed and clustered groupings, respectively. The volcanic map illustrates the distinctive metabolites between CTR and HFD (Fig. 4c, CTR = 5, HFD = 6), with 103 differential metabolites identified. Nitroxoline and pyrogallol,28 anti-inflammatory products, significantly reduced the HFD group. Amongst these, HFD showed a significant reduction in 3a,7a,12a-trihydroxy-5b-cholestan-26-al,29 secondary bile acid metabolites, and n-trans-feruloyl octopamine, which promotes apoptosis (as indicated in Fig. 4c (CTR = 5, HFD = 6)).30 The KEGG database was employed to conduct an enrichment analysis on metabolic pathways to examine the mechanism behind how high-fat diets affect metabolite changes. Among other ways, purine metabolism and amino acid synthesis were observed to have significant metabolite differences, as illustrated in Fig. 4e (CTR = 5, HFD = 6).
3.7 HFD consumption alters the hippocampal microglial morphology in the hippocampus
It is recognized that microglia are activated after stimulation and their morphology changes. As shown in Fig. 5b and d (n = 3), the number of microglia in the HFD group significantly increased. Moreover, the staining indicated that microglia in the dentate gyrus (DG) area showed apparent antenna retraction and cell size, suggesting that the HFD led to microglial activation.
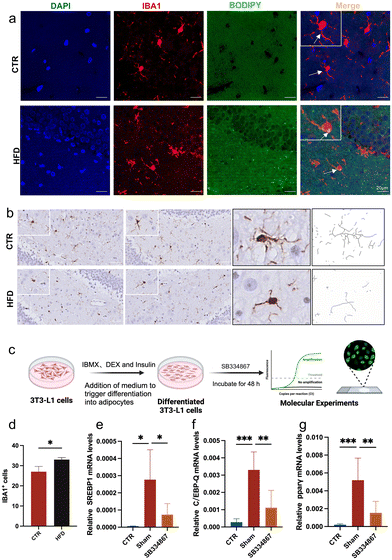 |
| Fig. 5 (a) Representative morphological changes were observed in microglia and lipid accumulation in the DG region of HFD and CTR groups (bar = 20 μm). (b) Hippocampal histochemical staining (IBA1) of the HFD group revealed altered cell morphology and activated microglia. (c) Schematic diagram of induced differentiation and drug incubation of 3T3-L1 cells. (d) Immunohistochemical quantitative results for IBA1 were collected (n = 3). (e–g) Adipogenic factor mRNA levels (SREBP1, C/EBP-Q, and PPARγ) were measured following differentiation of 3T3-L1 cells. After treatment with SB334867, mRNA levels of adipogenic factors were significantly reduced (n = 4). Statistical analysis using one-way ANOVA followed by Dunnett's test for post-hoc comparisons. Significant results were observed with *p < 0.05, **p < 0.01, ***p < 0.001, and ****p < 0.0001. | |
3.8 Hypocretin-1 and SB334867 affect lipid accumulation in 3T3-L1 cells
Early studies have demonstrated that hypocretin-1 enhances the proliferation of 3T3-L1 preadipocytes.31 Our experimental results indicate that hypocretin-1 can promote the accumulation of lipids (Fig. S4a and b,†n = 10; Fig. S4c–e,†n = 4). Oil red O staining and the results of absorption light intensity of isopropyl alcohol elution showed that SB334867 substantially reduced lipid accumulation (Fig. 6b and d, n = 10). Post-induced differentiation affected the expression of the adipogenic transcription factors involving C/EBPα, PPARγ, and SREBP-1, which were significantly increased. The manifestation of lipogenic genes was significantly decreased in the SB334867 group (Fig. 5e–g, n = 4). The lipid dye BODIPY was used to stain the cells with immunofluorescence, and the average fluorescence intensity also indicated that SB334867 reduced lipid accumulation (Fig. 6a and c, n = 4).
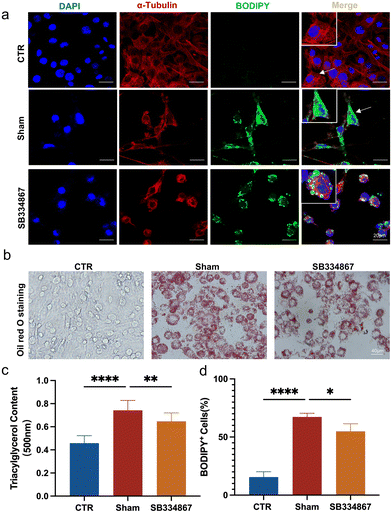 |
| Fig. 6 Effects of SB334867 on lipid accumulation in 3T3-L1 cells. (a) Immunofluorescence staining of 3T3-L1 cells showed that the SB334867 reversed lipid accumulation in 3T3-L1 cells. (b) Oil red O staining of 3T3-L1 cells. (c) Quantification of the relative fluorescence intensity for BODIPY (n = 4). (d) After oil red O staining, the cells were eluted with isopropanol, and the absorbed light intensity was measured at 500 nm using a microplate reader (n = 10). One-way ANOVA analysis followed by Dunnett's test for post-hoc comparisons, *p < 0.05, **p < 0.01, ***p < 0.001, ****p < 0.0001. | |
3.9 3T3-L1 CM leads to lipid accumulation and activation in BV2 cells
Lipid droplets containing neutral lipids acting as storage organelles are becoming widely accepted as markers of inflammation.32 Microglia regulate lipid metabolism.33 Our study revealed that BV2 cells were activated and able to phagocytose lipid droplets following treatment with a CM of 3T3-L1. The average fluorescence intensity of BODIPY showed no significant differences among the Sham and SB334867 treated groups, indicating that microglia were stimulated to phagocytose lipids for preserving environmental homeostasis. The CM to the model group increased the average fluorescence intensity of IBA1, which was then reversed in the SB334867 group (Fig. 7b, f and g and Fig. S4e, i and j,†n = 6). As illustrated in Fig. 7c–e and Fig. S4 (k–m),† (n = 5), the mRNA expression of inflammatory-related factors of BV2 cells exhibiting lipid droplet accumulation increased, and the SB334867 CM group markedly decreased.
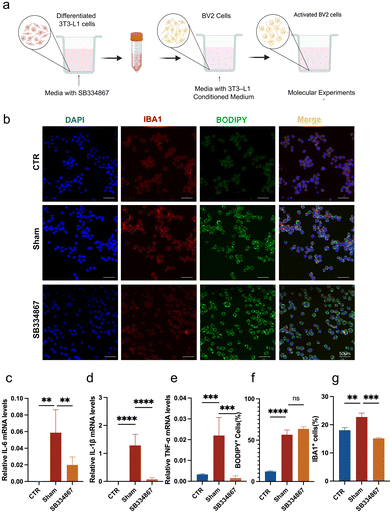 |
| Fig. 7 Effect of the 3T3-L1 cell supernatant on BV2 cells. (a) Flowchart illustrating the process of acquiring conditioned medium from 3T3-L1 cells before introducing BV2 cells. (b) Immunofluorescence (IBA1 and BODIPY) showed that the conditioned medium increased lipid accumulation in BV2 cells; however, the IBA1 intensity was altered. (c–e) The mRNA levels of inflammatory factors (IL-6, IL-1β, and TNF-α) were analyzed. BV2 cells were altered following the introduction of the conditioned medium. Implementing an antagonist treatment notably decreased the expression of inflammatory substances (n = 5). (f and g) While the BODIPY average fluorescence intensity of BV2 immunofluorescence staining remained unchanged between the treatment and model groups, the mean fluorescence intensity of IBA1 observed through BV2 immunofluorescence staining of the antagonist group was significantly lower than that of the model group (n = 6). One-way ANOVA analysis followed by Dunnett's test for post-hoc comparisons, no significant difference (n.s.) (*p < 0.05, **p < 0.01, ***p < 0.001, ****p < 0.0001). | |
3.10 SB334867 reversed the phenotype and plasma biochemical levels conferred by a HFD
The SB334867 injection significantly improved the weight gain caused by the HFD diet (as shown in the Fig. 8g, n = 5), and SB334867 improved the increase in TC and HDL plasma biochemical indices caused by HFD (Fig. 8h, j and k, n = 5). The supplementation of the HFD significantly elevated plasma LDL levels. However, the use of the SB334867 only reduced plasma LDL levels, and the difference was not statistically significant. Meanwhile, consistent with the previous results, SB334867 did not improve TG indexes, possibly due to the short injection time (Fig. 8i, n = 5).
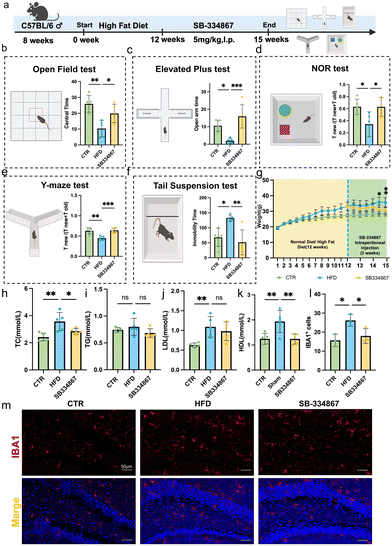 |
| Fig. 8 SB334867 reversed the HFD-induced depressive and anxiety-behaviors and microglial activation. (a) The timeline of the HFD model, drug administration and behavioral tests. (b) Compared with the HFD group, SB334867 significantly reversed HFD-induced decreased central time in open field test (n = 5). (c) SB334867 significantly reversed HFD-induced decreased open arm time in the elevated plus maze test (n = 5). (d) SB334867 significantly increased the exploration time in new object compared with the HFD group in the NOR test (n = 5). (e) SB334867 significantly increased the exploration time in new arm compared with the HFD group in the Y-maze test (n = 5). (f) SB334867 significantly decreased the immobility time in the tail suspension test (n = 5). (g) Body weight change during the HFD. SB334867 significantly reduced HFD-induced increase (n = 5). (h–k) SB334867 reversed the HFD-induced changes in plasma biochemical indicators, including TC, TG, LDL and HDL (n = 5). (l) Compared with the HFD group, the SB334867 group showed a reduced number of IBA1+ cells (n = 3). (m) Typical microscopic images of immunofluorescence staining of IBA1 in different groups in the hippocampus. One-way ANOVA analysis followed by Dunnett's test for post-hoc comparisons, no significant difference (n.s.) (*p < 0.05, **p < 0.01, ***p < 0.001, ****p < 0.0001). | |
3.11 SB334867 reversed the depression and anxiety-like behaviours induced by a HFD
In the open field test, the SB334867 group exhibited a longer centre stay time compared to the HFD group (Fig. 8b, n = 5); whereas in the elevated cross test, the HFD group exhibited a shorter open arm exploration time, which was significantly improved by treatment with SB334867 (Fig. 8c, n = 5); similarly, in the hanging tail test, the immobility time of the animals in the SB334867 group was significantly reduced, suggesting that treatment with SB334867 significantly improved the depression and anxiety-like behaviour caused by the HFD diet (Fig. 8f, n = 5).
In the novel object recognition test and the Y-maze test, SB334867 significantly improved HFD-induced cognitive impairment: in the NOR test, SB334867 treatment increased the exploration time for novel objects (Fig. 8d, n = 5); in the Y-maze test, SB334867 treatment increased the exploration time for novel arms (Fig. 8e, n = 5).
3.12 SB334867 rescues HFD-induced microglial activation in the brain
Following SB334867 treatment in mice fed an HFD for 12 weeks, as shown in Fig. 8m (n = 3), the activation of microglia induced by the HFD was alleviated after SB334867 supplementation, evidenced by the decreased microglial number in the hippocampus.
4. Discussion
The objective of this study was to investigate the relationship between hypocretin-1 and HFD induced depressive and anxiety behaviors. Our findings suggest that HFD resulted in increased hypothalamic hypocretin levels and impaired gut microbiota and metabolite abundance in rats and promoted lipid accumulation, persistent microglial activation, and central inflammation, which may lead to psychiatric disorders (Fig. 9).
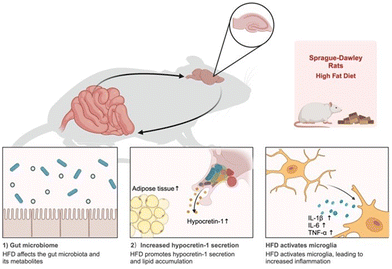 |
| Fig. 9 HFD may affect the abundance of gut microbiota, increasing the abundance of harmful bacteria and decreasing the abundance of beneficial bacteria. Changes in the gut microbiota affect the brain through the gut–brain axis. We found that along with fat accumulation, hypocretin secretion also increased. At the same time, microglia, which maintain brain homeostasis, are disturbed, and are activated by a variety of factors (including hypocretin and HFD), increasing the release of pro-inflammatory factors, and ultimately leading to depression, anxiety, and other mental disorders. | |
High-fat diet results in elevated hypothalamic secretin levels, altered gut microbiota and metabolite abundance in rats. Hypocretin-1 modulates appetite stimulation, energy expenditure and body weight. It has also been observed to promote lipid accumulation in human adipocytes,34 expressing HCRTR1 and HCRTR2.16 Hypocretin-1 can directly contribute to peripheral tissues through its receptors.35 Hypocretin-1 may regulate food intake and depression and anxiety via peripheral circulation. Clinical studies have shown that hypocretin play an important role in eating disorders.36,37 A HFD increased hypocretin-1 mRNA levels in rats; interestingly, binge eating behavior was inhibited only by HCRTR1 antagonist but not HCRTR2 antagonist.38 Our previous study demonstrated that the level of hypocretin-1 in the cerebrospinal fluid of healthy individuals fluctuates between 300 and 450 pg mL−1.39 Similarly, the level of hypocretin-1 in the peripheral plasma of healthy individuals fluctuates between 20 and 50 pg mL−1.40 Comparable to our study, the level of hypocretin-1 in the peripheral blood of healthy rodents fluctuates between 200 and 400 pg mL−1.41 Previous studies have also revealed that the normal cerebrospinal fluid level of rodents fluctuates between 500 and 700 pg mL−1.42 Given the disparate in vitro and in vivo environments and considering the findings of peer experiment,16 we selected a concentration of 200 nmol hypocretin-1 in combination with the pre-experiment.
In addition, HCRTR1 antagonist significantly reduced the food intake in rats.43 To further validate the role of the hypocretin system in HFD, the process of cell differentiation was found to increase lipid accumulation in 3T3-L1 cells. While the supernatant of differentiated can activate BV2 cells and increase the expression of inflammatory factors. And it was ameliorated by pre-treatment with SB334867. It was further validated in a 12-week HFD mouse model. Specifically, SB334867 significantly ameliorated HFD-induced increased body weight and depressive and anxiety-like behaviors. In addition, the imbalance of plasma biochemical levels and microglial activation in the brain were reserved. In conclusion, this suggests that HFD-induced elevated hypocretin-1 levels may play an important role in its associated depressive and anxiety-like behaviors.
Microglia have been found to activate in response to weight gain and increased appetite induced by the HFD.15 Microglia maintain homeostasis in the brain as an important immune role of the central nervous system (CNS),44 activation of which significantly increases CNS neuroinflammation, leading to neurodegenerative pathologies.45 Activated by immune factors secreted by adipose tissue, there is an increase in the release of inflammatory factors, leading to neurological damage and mood disorders.46 Previous studies have found that hypocretin promote adipocyte value addition,34 like our findings, hypocretin-1 promote triacylglycerol production in adipocytes. It was found that hypocretin-1-induced increased lipid accumulation and secretion of associated factors in adipocytes significantly activated microglia and promoted the expression of inflammatory factors, which can be significantly reversed by the SB334867. Therefore, hypocretin-1 may promote lipid accumulation and thus induce microglia activation, leading to central inflammation, contributing to mood disorders.
HFD significantly altered the composition of gut flora,47 which plays an important role in the bidirectional gut–brain axis.48 Dysregulated gut ecology can lead to cognitive decline through direct inflammatory stimulation, production of pro-inflammatory metabolites, and loss of immunomodulatory activity.49 The role of gut microbiota in the association between HFD and psychiatric disorders may be related to neuroinflammation induced by pro-inflammatory environmental stimuli, changes in neurotransmitters and short-chain fatty acids, and overproduction of HPA axis corticosteroids.50 Our study found that in response to HFD, there were significant changes in gut flora species, including the increase of the inflammation-associated bacterium (Candidatus_Woykebacteria_nonname) and the downregulation of the beneficial flora (Prevotellamassilia), which is usually considered to have an anti-inflammatory effect.51
However, there was a lack of validation of peripheral levels of hypocretin-1. Additionally, hypocretin is thought to have significant sex differences; the verification in terms of gender is deficient. Only a HFD was explored, without considering other diets including high-carbohydrate and high-protein diets, which is a direction we urgently need to explore in the future. In terms of serum biochemical levels, the HFD did not result in a significant difference in TG in this experiment. It is possible that a partially unknown mechanism may be involved. Paraffin sections may have some controversies for multiple finally, regarding the immunofluorescence. Finally, we only observed the changes in the hippocampus, and did not consider the changes in the other brain regions in this study, while psychiatric disorders can have a wide range of effects on multiple brain regions, which is what we are going to consider in the future as well.
5. Conclusions
In summary, we explored the association between HFD-induced depressive and anxiety-like behaviors and the hypocretin system. A HFD resulted in an increased hypothalamic hypocretin level, leading to an inflammatory response in the central system, which in turn leads to depressive and anxiety-like behaviors.
Author contributions
JYD: writing – original draft and formal analysis; JHZ: validation and writing – original draft; SPC: visualization; BQ: resources; KYJ and BC: conceptualization; JL and YYZ: funding acquisition and writing – review and editing.
Data availability
The data supporting this article have been included as part of the ESI.†
Conflicts of interest
The authors declare that they have no known competing financial interests or personal relationships that could have appeared to influence the work reported in this paper.
Acknowledgements
We sincerely acknowledge the support of funds from the National Natural Science Foundation of China (82271561), the Medical Science and Technology Project of Zhejiang Province (2022RC024) and the Natural Science Foundation of Zhejiang Province (LQ20H090010). The figure of the article was drawn using BioRender (https://biorender.com/). The correlation index was calculated by a correlation method using the OmicShare tools (https://www.omicshare.com/tools).
References
- F. N. Jacka, N. Cherbuin, K. J. Anstey, P. Sachdev and P. Butterworth, BMC Med., 2015, 13, 215 CrossRef PubMed
.
- R. S. Duman, N. Li, R. J. Liu, V. Duric and G. Aghajanian, Neuropharmacology, 2012, 62, 35–41 CrossRef CAS PubMed
.
- R. Ghasemi, A. Haeri, L. Dargahi, Z. Mohamed and A. Ahmadiani, Mol. Neurobiol., 2013, 47, 145–171 CrossRef CAS PubMed
.
- Y. Duan, C. Llorente, S. Lang, K. Brandl, H. Chu, L. Jiang, R. C. White, T. H. Clarke, K. Nguyen, M. Torralba, Y. Shao, J. Liu, A. Hernandez-Morales, L. Lessor, I. R. Rahman, Y. Miyamoto, M. Ly, B. Gao, W. Sun, R. Kiesel, F. Hutmacher, S. Lee, M. Ventura-Cots, F. Bosques-Padilla, E. C. Verna, J. G. Abraldes, R. S. Brown Jr, V. Vargas, J. Altamirano, J. Caballería, D. L. Shawcross, S. B. Ho, A. Louvet, M. R. Lucey, P. Mathurin, G. Garcia-Tsao, R. Bataller, X. M. Tu, L. Eckmann, W. A. van der Donk, R. Young, T. D. Lawley, P. Stärkel, D. Pride, D. E. Fouts and B. Schnabl, Nature, 2019, 575, 505–511 CrossRef CAS PubMed
.
- D. H. Dapito, A. Mencin, G. Y. Gwak, J. P. Pradere, M. K. Jang, I. Mederacke, J. M. Caviglia, H. Khiabanian, A. Adeyemi, R. Bataller, J. H. Lefkowitch, M. Bower, R. Friedman, R. B. Sartor, R. Rabadan and R. F. Schwabe, Cancer Cell, 2012, 21, 504–516 CrossRef CAS PubMed
.
- L. Abenavoli, E. Scarpellini, C. Colica, L. Boccuto, B. Salehi, J. Sharifi-Rad, V. Aiello, B. Romano, A. De Lorenzo, A. A. Izzo and R. Capasso, Nutrients, 2019, 11, 2690 CrossRef CAS PubMed
.
- F. Shanahan, Semin. Gastrointest. Dis., 1999, 10, 8–13 CAS
.
- D. A. Drossman, J. Clin. Gastroenterol., 2005, 39, S251–S256 CrossRef PubMed
.
- E. A. Mayer, Nat. Rev. Neurosci., 2011, 12, 453–466 CrossRef CAS PubMed
.
- J. Zhang, D. Feng, H. K. Law, Y. Wu, G. H. Zhu, W. Y. Huang and Y. Kang, Microbiol. Spectrum, 2021, 9, e0065021 CrossRef PubMed
.
- T. G. Dinan and J. F. Cryan, J. Physiol., 2017, 595, 489–503 CrossRef CAS PubMed
.
- D. Erny, A. L. Hrabě de Angelis, D. Jaitin, P. Wieghofer, O. Staszewski, E. David, H. Keren-Shaul, T. Mahlakoiv, K. Jakobshagen, T. Buch, V. Schwierzeck, O. Utermöhlen, E. Chun, W. S. Garrett, K. D. McCoy, A. Diefenbach, P. Staeheli, B. Stecher, I. Amit and M. Prinz, Nat. Neurosci., 2015, 18, 965–977 CrossRef CAS PubMed
.
- H. Keren-Shaul, A. Spinrad, A. Weiner, O. Matcovitch-Natan, R. Dvir-Szternfeld, T. K. Ulland, E. David, K. Baruch, D. Lara-Astaiso, B. Toth, S. Itzkovitz, M. Colonna, M. Schwartz and I. Amit, Cell, 2017, 169, 1276–1290 CrossRef CAS PubMed
.
- D. M. Norden, P. J. Trojanowski, E. Villanueva, E. Navarro and J. P. Godbout, Glia, 2016, 64, 300–316 CrossRef PubMed
.
- A. Niraula, R. D. Fasnacht, K. M. Ness, J. M. Frey, S. A. Cuschieri, M. D. Dorfman and J. P. Thaler, Diabetes, 2023, 72, 233–244 CrossRef CAS PubMed
.
- M. Skrzypski, T. L. T. P. Kaczmarek, E. Pruszynska-Oszmalek, P. Pietrzak, D. Szczepankiewicz, P. A. Kolodziejski, M. Sassek, A. Arafat, B. Wiedenmann, K. W. Nowak and M. Z. Strowski, Diabetologia, 2011, 54, 1841–1852 CrossRef CAS PubMed
.
- T. Sakurai, A. Amemiya, M. Ishii, I. Matsuzaki, R. M. Chemelli, H. Tanaka, S. C. Williams, J. A. Richardson, G. P. Kozlowski, S. Wilson, J. R. Arch, R. E. Buckingham, A. C. Haynes, S. A. Carr, R. S. Annan, D. E. McNulty, W. S. Liu, J. A. Terrett, N. A. Elshourbagy, D. J. Bergsma and M. Yanagisawa, Cell, 1998, 92, 573–585 CrossRef CAS PubMed
.
- M. Nakabayashi, T. Suzuki, K. Takahashi, K. Totsune, Y. Muramatsu, C. Kaneko, F. Date, J. Takeyama, A. D. Darnel, T. Moriya and H. Sasano, Mol. Cell. Endocrinol., 2003, 205, 43–50 CrossRef CAS PubMed
.
- U. Suryavanshi, K. K. Angadi, V. S. Reddy and G. B. Reddyx, Chem.-Biol. Interact., 2023, 110823, DOI:10.1016/j.cbi.2023.110823
.
- A. Wu and J. Zhang, J. Neuroinflammation, 2023, 20, 283 CrossRef CAS PubMed
.
- M. Saraiva and A. O'Garra, Nat. Rev. Immunol., 2010, 10, 170–181 CrossRef CAS PubMed
.
- J. Hara, C. T. Beuckmann, T. Nambu, J. T. Willie, R. M. Chemelli, C. M. Sinton, F. Sugiyama, K. Yagami, K. Goto, M. Yanagisawa and T. Sakurai, Neuron, 2001, 30, 345–354 CrossRef CAS PubMed
.
- A. Everard, V. Lazarevic, M. Derrien, M. Girard, G. G. Muccioli, A. M. Neyrinck, S. Possemiers, A. Van Holle, P. François, W. M. de Vos, N. M. Delzenne, J. Schrenzel and P. D. Cani, Diabetes, 2011, 60, 2775–2786 CrossRef CAS PubMed
.
- R. Zhu, J. Tang, C. Xing, Q. Nan, G. Liang, J. Luo, J. Zhou, Y. Miao, Y. Cao, S. Dai and D. Lan, Front. Microbiol., 2022, 13, 883495 CrossRef PubMed
.
- T. C. Kwong, E. C. T. Chau, M. C. H. Mak, C. T. Choy, L. T. Chan, C. K. Pang, J. Zhou, P. H. C. Poon, Y. Guan, S. K. W. Tsui, S. W. Chan, G. P. H. Leung, W. C. S. Tai and Y. W. Kwan, Animals, 2023, 13, 2479 CrossRef PubMed
.
- W. Song, Y. Wang, G. Li, S. Xue, G. Zhang, Y. Dang and H. Wang, Gut Microbes, 2023, 15, 2276814 CrossRef PubMed
.
- X. Li, Y. Ling, X. Huang, T. Zhou, S. Wu, S. Zhang, H. Zhou, Y. Kang, L. Wang, X. Wang and W. Yin, Nutrients, 2023, 15, 4560 CrossRef CAS PubMed
.
- Y. Zhang, S. Yang, Z. Qiu, L. Huang, L. Huang, Y. Liang, X. Liu, M. Wang and B. Zhou, Free Radicals Biol. Med., 2022, 191, 66–81 CrossRef CAS PubMed
.
- G. A. Altê and A. L. S. Rodrigues, Pharmaceuticals, 2023, 16, 1013 CrossRef PubMed
.
- B. Ma, J. Li, W. K. Yang, M. G. Zhang, X. D. Xie and Z. T. Bai, Anal. Cell. Pathol., 2021, 2021, 1560307 Search PubMed
.
- K. Zwirska-Korczala, M. Adamczyk-Sowa, P. Sowa, K. Pilc, R. Suchanek, K. Pierzchala, G. Namyslowski, M. Misiolek, K. Sodowski, I. Kato, A. Kuwahara and R. Zabielski, J. Physiol. Pharmacol., 2007, 58 Suppl 1, 53–64 CAS
.
- P. T. Bozza and J. P. Viola, Prostaglandins, Leukotrienes Essent. Fatty Acids, 2010, 82, 243–250 CrossRef CAS PubMed
.
- M. S. Ioannou, J. Jackson, S. H. Sheu, C. L. Chang, A. V. Weigel, H. Liu, H. A. Pasolli, C. S. Xu, S. Pang, D. Matthies, H. F. Hess, J. Lippincott-Schwartz and Z. Liu, Cell, 2019, 177, 1522–1535 CrossRef CAS PubMed
.
- J. E. Digby, J. Chen, J. Y. Tang, H. Lehnert, R. N. Matthews and H. S. Randeva, J. Endocrinol., 2006, 191, 129–136 CAS
.
- C. E. Perez-Leighton, C. J. Billington and C. M. Kotz, Biochim. Biophys. Acta, 2014, 1842, 440–445 CrossRef CAS PubMed
.
- J. Bronsky, J. Nedvidkova, H. Krasnicanova, M. Vesela, J. Schmidtova, J. Koutek, R. Kellermayer, M. Chada, Z. Kabelka, M. Hrdlicka, J. Nevoral and R. Prusa, Int. J. Eat. Disord., 2011, 44, 547–552 CrossRef PubMed
.
- M. Janas-Kozik, M. Stachowicz, I. Krupka-Matuszczyk, J. Szymszal, K. Krysta, A. Janas and J. K. Rybakowski, Regul. Pept., 2011, 168, 5–9 CrossRef CAS PubMed
.
- L. Piccoli, M. V. Micioni Di Bonaventura, C. Cifani, V. J. Costantini, M. Massagrande, D. Montanari, P. Martinelli, M. Antolini, R. Ciccocioppo, M. Massi, E. Merlo-Pich, R. Di Fabio and M. Corsi, Neuropsychopharmacology, 2012, 37, 1999–2011 CrossRef CAS PubMed
.
- J. Lu, M. L. Huang, J. H. Li, K. Y. Jin, H. M. Li, T. T. Mou, R. Fronczek, J. F. Duan, W. J. Xu, D. Swaab and A. M. Bao, Schizophr. Bull., 2021, 47, 1310–1319 CrossRef PubMed
.
- H. Li, X. Chen, J. Dong, R. Liu, J. Duan, M. Huang, S. Hu and J. Lu, Life Sci., 2024, 344, 122581 CrossRef CAS PubMed
.
- B. Chen, J. Xu, S. Chen, T. Mou, Y. Wang, H. Wang, Z. Zhang, F. Ren, Z. Wang, K. Jin and J. Lu, J. Affective Disord., 2023, 325, 256–263 CrossRef CAS PubMed
.
- P. J. Martins, V. D'Almeida, M. Pedrazzoli, L. Lin, E. Mignot and S. Tufik, Regul. Pept., 2004, 117, 155–158 CrossRef CAS PubMed
.
- L. Buczek, J. Migliaccio and G. D. Petrovich, Neuroscience, 2020, 436, 34–45 CrossRef CAS PubMed
.
- A. Aguzzi, B. A. Barres and M. L. Bennett, Science, 2013, 339, 156–161 CrossRef CAS PubMed
.
- H. D. E. Booth, W. D. Hirst and R. Wade-Martins, Trends Neurosci., 2017, 40, 358–370 CrossRef CAS PubMed
.
- M. Jian, J. S. Kwan, M. Bunting, R. C. Ng and K. H. Chan, J. Neuroinflammation, 2019, 16, 110 CrossRef PubMed
.
- P. J. Turnbaugh, F. Bäckhed, L. Fulton and J. I. Gordon, Cell Host Microbe, 2008, 3, 213–223 CrossRef CAS PubMed
.
- M. Solas, F. I. Milagro, M. J. Ramírez and J. A. Martínez, Curr. Opin. Pharmacol., 2017, 37, 87–92 CrossRef CAS PubMed
.
- K. M. Neufeld, N. Kang, J. Bienenstock and J. A. Foster, Neurogastroenterol. Motil., 2011, 23, 255–264 CrossRef CAS PubMed
.
- A. Agustí, M. P. García-Pardo, I. López-Almela, I. Campillo, M. Maes, M. Romaní-Pérez and Y. Sanz, Front. Neurosci., 2018, 12, 155 CrossRef PubMed
.
- J. Sang, D. Zhuang, T. Zhang, Q. Wu, J. Yu and Z. Zhang, mSystems, 2022, 7, e0151221 CrossRef PubMed
.
|
This journal is © The Royal Society of Chemistry 2024 |