DOI:
10.1039/D3QI01213A
(Research Article)
Inorg. Chem. Front., 2023,
10, 5602-5610
The transformation of a zero-dimensional cluster into a one-dimensional chain structure achieving a dramatically enhanced birefringence in tin(II)-based oxalates†
Received
29th June 2023
, Accepted 18th August 2023
First published on 19th August 2023
Abstract
Developing new birefringent materials with large optical anisotropy is extremely urgent due to the fantastic progress of laser science and technology. Here, two tin(II)-based oxalates, K2Sn(C2O4)2·H2O and K2Sn2(C2O4)2F2·H2O, were successfully synthesized by simultaneously introducing π-conjugated [C2O4]2− groups and distorted Sn2+-polyhedra with stereochemically active lone pairs. The use of F− regulates the arrangement of birefringence-active groups, resulting in the transformation of zero-dimensional (0D) K2Sn(C2O4)2·H2O into 1D K2Sn2(C2O4)2F2·H2O, which successfully enhances birefringence from 0.103@546 nm to 0.301@546 nm. Meaningfully, detailed structural and property analysis demonstrates that the ideal spatial arrangement of all the birefringence-active functional modules can induce strong optical anisotropy, providing an idea for designing birefringent materials with excellent properties.
Introduction
Birefringent crystals, an important component of optoelectronic devices, exhibit strong light modulation ability.1,2 In particular, crystals with large birefringence play an extremely critical role in both military and civilian applications, such as medical diagnosis, fiber-optic communications, flat panel displays, polarimetry, etc.3,4 In the past decades, many birefringent crystals, including natural (e.g., quartz crystal, mica, calcite and so on) and artificial (e.g., α-BaB2O4(α-BBO),5 MgF2,6 YVO4
7 and so on) birefringent crystals, have been discovered and synthesized. Among these some have already gained commercial interest, but their inherent defects, such as unavoidable impurities of natural crystals and low transmittance in the UV region for YVO4
7 and CaCO3,8 prevent their application in more advanced miniature optical devices for future markets. Therefore, the pressing need for new optical crystals with excellent performance, a large birefringence, stable physicochemical properties and easy growth characteristics is driving research efforts.
The birefringence of crystals essentially arises from the anisotropic response of the electron distribution to the applied electric field, and the following groups have been confirmed to enhance the birefringence of compounds due to their strong anisotropic response: (1) π-conjugated planar units, such as HxBO3(x = 0–3), HxCO3(x = 0–2), HxB3O6(x = 0–3), HxC3N3O3(x = 0–3), and HxC3O4(x = 0–4).9–17 Wang's group reported that Rb3Na(H2C3N3O3)4·3H2O18 crystals exhibit a large birefringence of 0.368@1064 nm, which essentially derives from delocalized conjugated orbitals on the planar (H2C3N3O3)−. (2) Distorted tetrahedral moieties, such as fluorooxoborates [BOxF4−x](x+1)− and fluorophosphates PO3F, which have produced BaB8O12F2 (0.116@1064 nm)19 and (N2H6)[HPO3F]2 (0.077@1064 nm)20 with enhanced birefringence. (3) Jahn–Teller cations include the specifically octahedrally coordinated d0 transition metals (TMs: Mo6+, W6+, V5+, and Nb5+) and cations with stereochemically active lone pairs (SCALP, I5+, Te4+, Se4+, and Sn2+) such as d0 transition metal cations Nb5+ in K3Nb3Ge2O13 (0.196@546 nm)21 and (R)- and (S)-[C8H10NO3]2[NbOF5] (0.19–0.199@589.3 nm),22 Ti4+ in BaTi(BO3)2 (0.169@546 nm),23 stereochemically active lone pair (SCALP) cations Sn2+ in Sn2PO4Br (0.336@546 nm),24 Sb3+ in K2SbP2O7F (0.157@546 nm),25 and SbB3O6 (0.290@546 nm),26 I5+ in LiGaF2(IO3)2 (0.206@532 nm).27 The current strategy focuses on the simultaneous introduction of multiple birefringence-active functional modules (FMs) mentioned above into one structure to construct optical materials with large birefringence. Noteworthily, the large birefringence is determined not only by the screening of FMs, but also their spatial arrangement. In 2018, Jayakanth Ravichandran et al. found that the quasi-one-dimensional (quasi-1D) structural configuration in BaTiS3 could increase the polarizability anisotropy and favorably bring large birefringence up to 0.76 in the mid- to long-wave infrared region.28 Beyond that, the structural advantages of the 1D configuration are also reflected in Na2BP2 (0.68@2000 nm)29 and [C(NH2)3]Sb(C2O4)F2·H2O (0.323@546 nm).30 Hence, selecting suitable FMs to construct 1D structures is a promising strategy to gain crystals with large birefringence.
Obviously, a 1D configuration is an ideal structural framework for obtaining large birefringence as described above. Nevertheless, not all birefringence-active FMs can be constructed into chains, since the formation of chains has an indispensable condition, i.e., FMs with a unique coordination environment can prevent chain-to-chain connection and extension. So how do we effectively screen out the appropriate birefringence-active FMs? In molecular engineering, chemical scissors have been proved to be reliable structure-directing agents in the controlled design of crystal microstructures. To our knowledge, lone pair electrons act as chemical scissors to architecturally shape the target structure in many classical compounds of Pb2BO3Cl,31 Cs2Pb(NO3)2Br2,32 and KSb2C2O4F5,33 because cations occupied by lone pair electrons on one side can form highly distorted polyhedra that not only exhibit large anisotropic polarizabilities, but also prevent connection with other structure modules on the same side. The Sn2+ cation is a highly promising candidate that, in addition to possessing SCALP, has rich coordination modes (terdentate, quadridentate, quinquidentate, and sexadentate),34–36 allowing for increased structural diversity, e.g., Sn2B5O9Cl,37 α-SnF2,38 K3Sn2(SO4)3Cl,34 and Sn2[B7O12]F.39 In addition, the presence of planar anionic groups is essential for the development of low-dimensional structures because of their unique structural malleability. In recent years, experimental and theoretical evidence from Pan's and Zou's groups has demonstrated that planar C2O42− groups are promising birefringence-active FMs, as exemplified by (NH4)2C2O4·H2O,40 Na2Sb2(C2O4)F6,41 Cs2Sb2(C2O4)2F4·H2O
41 and so on. The four O atoms of the C2O42− groups all lie in the same plane, resulting in adjacent groups extending along the plane by sharing O atoms and ultimately forming a low-dimensional structure. In terms of properties, the C2O42− groups exhibit strong π-conjugated interactions due to shorter bond lengths, strong Pπ–Pπ interactions and additional electron-populated p orbitals provided by C and O atoms, resulting in the production of large anisotropic polarizability and even large birefringence.
Based on the above ideas, the Sn2+–C2O42− system has been studied systematically. K2Sn(C2O4)2·H2O was successfully synthesized by the hydrothermal reaction method. Regrettably, however, although K2Sn(C2O4)2·H2O consists of Sn2+-polyhedra and C2O42− groups, it features a 0D anionic structure. We noticed that F− ions are also widely considered to be excellent chemical scissors. When the F− ions are introduced into the cation-centered polyhedra(M-polyhedra) of oxygenates, the other oxygen ligands are squeezed and moved toward the equator, which not only decrease the symmetry of the polyhedra, but also facilitate the extension of the polyhedra by sharing oxygen atoms. The C–F bond, which is generally shorter than the M–O bond, could cut the connecting bridge of chain to chain. As a result, F− was considered for introduction into the Sn2+–C2O42− system, and K2Sn2(C2O4)2F2·H2O featuring a unique 1D anionic structure was successfully synthesized by the solution evaporation method. Fascinatingly, as the crystal structure transforms from 0D to 1D, the birefringence increases dramatically from 0.103@546 nm in K2Sn(C2O4)2·H2O to 0.301@546 nm as expected.
Experimental section
Synthesis of K2Sn(C2O4)2·H2O and K2Sn2(C2O4)2F2·H2O
Reaction reagents: SnO (AR, 99%), K2C2O4·H2O (AR, 99.8%), SnF2 (AR, 99%), and HF (AR, ≥40.0%). All materials were used as received and without further operation. There are some important things to follow when using HF: pay attention to ventilation, do a good job of safety protection, wear rubber acid-alkali resistant clothing and gloves, wash your hands thoroughly after use, and keep the place clean.
The compound K2Sn(C2O4)2·H2O was synthesized by the hydrothermal reaction method. SnO (0.135 g) and K2C2O4·H2O (0.737 g) were added into H2O (3 mL) and HF (0.1 mL). After 20 minutes of stirring, the mixture was sealed into an autoclave with a 23 mL Teflon liner and heated at 100 °C for 5 days, and then slowly cooled down to room temperature at 6 °C h−1. In order to obtain a pure compound, the reaction mixture has to be rinsed with ethanol, and dried in air.
The compound K2Sn2(C2O4)2F2·H2O was gained by the solution evaporation method. A mixture of SnF2 (0.317 g) and K2C2O4·H2O (0.252 g) with a molar ratio of 2
:
3 was dissolved in H2O (5 mL), and the solution was reddish brown after stirring for 20 minutes. Then the solution was transferred into a refrigerator at 4 °C. After 5 days, the reaction product was washed with ethanol and dried in air. Finally, block-like crystals were obtained.
Single crystal structure determination
By using a Rigaku XtaLAB Synergy R diffractometer with graphite monochromatic Mo-Kα radiation, the single crystal data of K2Sn(C2O4)2·H2O and K2Sn2(C2O4)2F2·H2O can be obtained at 150(2) K. The structures of the two compounds were refined with SHELXL-2014.42 We used the program PLATON43 to inspect the structure, and no higher symmetries could be found. The related crystal data and structure refinement of the two compounds are listed in Tables S1–S5.†
Powder X-ray Diffraction
A SmartLab powder X-ray diffractometer with Cu-Kα radiation was used to gather the powder X-ray diffraction patterns for K2Sn(C2O4)2·H2O, and K2Sn2(C2O4)2F2·H2O at room temperature. The decay test conditions are as follows: the 2θ angular range starts from 5° and stops at 70°, the scan step width is 0.02°, and the fixed time is 0.2 s. The test results show that the experimental pattern is consistent with the calculated pattern (Fig. S1†).
Thermal analysis
Thermogravimetric analysis of the two compounds was performed using NETZSCH STA-449C with a constant flow of N2 gas and the test temperature was from room temperature to 800 °C, with a heating rate of 10 °C per min (Fig. S2†).
Infrared spectroscopy
The data of infrared (IR) spectroscopy for K2Sn(C2O4)2·H2O and K2Sn2(C2O4)2F2·H2O were recorded with a Fourier transform infrared (FTIR) spectrometer with the model number Vertex 70. The range of measurement is 4000–400 cm−1. The proportion of the sample and dried KBr is about 1
:
100 (Fig. S4†).
UV–Vis diffuse reflectance spectroscopy
A Shimadzu UV-2600 spectrophotometer was used to record the data of UV-vis diffuse reflectance spectroscopy for the two compounds with the wavelength range of 200–800 nm at room temperature (Fig. S5†).
Birefringence measurements
Birefringence tests of K2Sn(C2O4)2·H2O and K2Sn2(C2O4)2F2·H2O were conducted on a ZEISS Axio Scope A5 polarizing microscope with a Berek compensator. The formula used to calculate the birefringence is listed as follows:
ΔR indicates the optical path difference, Δn refers to the birefringence, and d is the thickness of the crystal. The results of the tests are shown in Fig. S6.†
Theoretical calculations
The data of the theoretical calculations for both the compounds were calculated using a Cambridge Serial Total Energy Package (CASTEP)44 program, which is based on density functional theory (DFT).45 In this way, we could calculate the band structure, density of states, optical properties and so on. The Perdew–Burke–Ernzerhof (PBE)46 functional with Generalized Gradient Approximation (GGA) was employed for all calculations. To simplify the potential energy of all the atoms, Norm-conserving Pseudopotential (NCP)47 was adopted. The kinetic energy cutoffs for K2Sn(C2O4)2·H2O and K2Sn2(C2O4)2F2·H2O were 750 eV, and the k-points in the first Brillouin zone were 4 × 4 × 2 (K2Sn(C2O4)2·H2O) and 2 × 3 × 2 (K2Sn2(C2O4)2F2·H2O). The valences of all atoms are listed below: H 1s1, C 2s22p2, O 2s22p4, F 2s22p5, K 3s23p64s1, Sn 4d105s25p2.
Results and discussion
Crystal structures
The two centrosymmetric Sn2+-based oxalates K2Sn(C2O4)2·H2O and K2Sn2(C2O4)2F2·H2O crystallize in the triclinic space group P
(No. 2) and P21/c (No. 14), respectively, and the crystal structure of K2Sn(C2O4)2·H2O48 was previously reported. Their basic functional anionic groups are planar π-conjugated [C2O4]2− with rational bond lengths (C–O: 1.228–1.286 Å and C–C: 1.550–1.558 Å) and bond angles (O–C–O: 123.7–126.7°, O–C–C: 115.4–120.3°), which are interconnected with the cationic building modules Sn2+-based polyhedra and K+ cations to assemble crystal frameworks respectively (Fig. 1). It is interesting that the diverse coordination environments and spatial arrangements in these building blocks lead to the unique crystal structures of these two compounds.
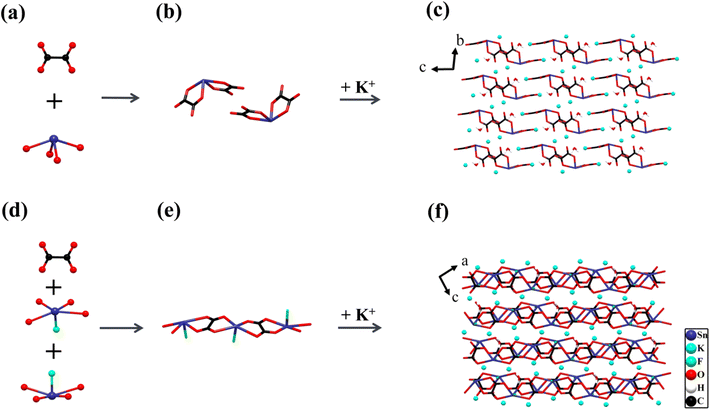 |
| Fig. 1 (a and d) The coordination modes of Sn2+ and planar [C2O4]2− groups; (b and e) the different connection modes between Sn2+-polyhedra and [C2O4]2− groups; (c and f) the structure of K2Sn(C2O4)2·H2O and K2Sn2(C2O4)2F2·H2O along the a-axis and b-axis, respectively. | |
K2Sn(C2O4)2·H2O contains one independent Sn atom, two K atoms, four C atoms, and nine O atoms in a unit cell. The Sn atom is four coordinated with four O atoms to form a SnO4 seesaw polyhedron with Sn–O bond distances of 2.148–2.375 Å (Fig. 1a). Each SnO4 seesaw polyhedron is linked to two nearly perpendicular [C2O4]2− planar groups by sharing four O atoms to form a 0D [Sn(C2O4)2]2− anionic structure (Fig. 1b). In addition, K+ cations with charge compensation and H2O molecules are distributed in the voids of these [Sn(C2O4)2]2− clusters (Fig. 1c).
For the compound K2Sn2(C2O4)2F2·H2O, the two independent Sn1 and Sn2 atoms are all connected with four O atoms and one F atom in a SnO4F tetragonal pyramid with the bond length of Sn–O of 2.281–2.729 Å and that of Sn–F of 2.020–2.045 Å, respectively (Fig. 1d). The [C2O4]2− planar groups act as bridges to connect [Sn(1)O4F]7− and [Sn(2)O4F]7− in opposite directions by sharing O atoms, constructing a 1D [Sn2(C2O4)2F2]∞2− anionic structure, and these chains are parallel to each other. The K atoms and H2O molecules are arranged between the chains, with the K atoms playing a major role in charge-balancing (Fig. 1e and f).
Thermal analysis
The results of thermogravimetric analysis of the two title compounds are shown in Fig. S2.† The decomposition temperatures of K2Sn(C2O4)2·H2O and K2Sn2(C2O4)2F2·H2O are 40 °C and 42 °C, and the corresponding total weight loss rates are about 40% and 27%, respectively. The final decomposition products of these two compounds were tested by X-ray powder diffraction, demonstrating that the main products of the two compounds are SnO2 and K2SnO3 in K2Sn(C2O4)2·H2O and SnO2 in K2Sn2(C2O4)2F2·H2O, respectively (Fig. S3†).
Infrared spectroscopy
As shown in Fig. S4,† the IR spectra of K2Sn(C2O4)2·H2O and K2Sn2(C2O4)2F2·H2O were recorded from 4000 to 400 cm−1. The peaks from 3416 cm−1 to 3709 cm−1 are the stretching vibrations of H2O. The peaks at 1637 cm−1 and 1642 cm−1 are identified as the stretching vibrations of C
O bonds. The bands at 1485 cm−1, 1421 cm−1, 1273 cm−1, and 1047 cm−1 can be ascribed to C–O stretching vibrations. The peaks at about 887 cm−1, 783 cm−1 and 786 cm−1 are considered to be the stretching vibrations of C–C and bending vibrations of O–C
O bonds. The characteristic absorption bands at around 493 cm−1 and 487 cm−1 belong to the vibrations of Sn–F bonds for K2Sn2(C2O4)2F2·H2O. All of the vibrations are in agreement with previously reported spectra in the literature.18,41,49–51
UV–Vis diffuse reflectance spectroscopy
The UV-vis diffuse reflectance spectra of K2Sn(C2O4)2·H2O and K2Sn2(C2O4)2F2·H2O are shown in Fig. S5.† It reveals that the band gaps of K2Sn(C2O4)2·H2O and K2Sn2(C2O4)2F2·H2O are 3.74 eV and 3.21 eV, and the corresponding UV absorption cutoff edges are 286 nm and 310 nm, respectively, illustrating that the two compounds can be used in the ultraviolet region.
Birefringence measurements
A ZEISS Axio A5 polarizing microscope was used to measure the birefringence of K2Sn(C2O4)2·H2O and K2Sn2(C2O4)2F2·H2O at 546 nm. The retardation and thickness of these two are 762 nm, 1803 nm and 7.433 μm, 5.993 μm, respectively. After substituting these values into eqn (1), the birefringence values of K2Sn(C2O4)2·H2O and K2Sn2(C2O4)2F2·H2O were obtained as 0.103 and 0.301 at 546 nm, respectively (Fig. 2a and b). In addition, the refractive indices of the compounds are calculated and presented in Fig. 2c and d. The results show that K2Sn(C2O4)2·H2O is positive biaxial crystals (nz − ny > ny − nx) and K2Sn2(C2O4)2F2·H2O is negative biaxial crystals (nz − ny < ny − nx). The calculated birefringence of these compounds is 0.113 and 0.300 at 546 nm, respectively, which closely match the experimental values. It is obvious that K2Sn2(C2O4)2F2·H2O has a significantly enhanced birefringence compared to K2Sn(C2O4)2·H2O, which surpasses that of traditional birefringent crystals such as LiNbO3 (0.074@546 nm),52 MgF2 (0.012@532 nm),6 α-BBO (0.122@546 nm),5 CaCO3 (0.172@532 nm),8 YVO4 (0.204@532 nm),7 and TiO2 (0.256@546 nm),53 and some reported oxalate crystals, e.g., (CN4H7)SbC2O4F2(H2O)0.5 (0.126@546 nm),54 KSb2C2O4F5 (0.170@546 nm),33 Rb2C2O4 (0.201@1064 nm),55 and (NH4)2SbC2O4Cl3 (0.270@546 nm).56 Besides, the title compounds are preferred in terms of stable physicochemical properties, suggesting that Sn2+-oxalates can be used as potential birefringent materials.
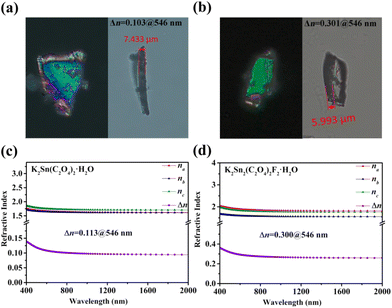 |
| Fig. 2 (a and b) Experimental birefringence at 546 nm; (c and d) calculated refractive index for K2Sn(C2O4)2·H2O and K2Sn2(C2O4)2F2·H2O. | |
Molecular engineering regulates birefringence
As described above, these two compounds employ the same birefringence-active FMs, i.e., π-conjugated [C2O4]2− planar groups and Sn2+ cations with SCALP, but their birefringence changes tremendously, which may indicate that the spatial arrangement of the FMs has a significant effect on the birefringence. By comparing the structural features of the two compounds, the only difference lies in the construction of the FMs, namely a 0D [Sn(C2O4)2]2− anionic structure in K2Sn(C2O4)2·H2O and a 1D [Sn2(C2O4)2F2]∞2− anionic structure in K2Sn2(C2O4)2F2·H2O. Then, the spatial arrangement characteristics of these two are analyzed in detail. The lone pair electrons of the Sn2+ cation act as chemical scissors to shear the tendency to connect to its ipsilateral side, so that all O ligands of the Sn are evenly distributed on the other side of the lone pair electrons in K2Sn(C2O4)2·H2O. As a result, the [C2O4]2− groups connected to the SnO4 seesaw polyhedron by shared O atoms are arranged non-coplanarly, and inconsistent orientations between [C2O4]2− groups and lone pair elections lead to a moderate birefringence of 0.103@546 nm (Fig. 3a and b). K2Sn2(C2O4)2F2·H2O has not only the chemical scissors of the lone pair electrons, but also other chemical scissors of the fluorine atoms. The shorter Sn–F bonds keep the chain from being linked to another chain by sharing F atoms in one direction, successfully forming the [Sn2(C2O4)2F2]∞2− chains in K2Sn2(C2O4)2F2·H2O. The 1D anionic structure is widely recognized as a structural mode with great potential to produce large birefringence, such as Na2Sb2(C2O4)F6 and Cs2Sb2(C2O4)2F4·H2O. In addition, the introduced F ligand occupies the space around Sn2+, forcing the four O ligands to shift toward the equatorial position of the Sn atom. Naturally, the [C2O4]2− groups attached to the Sn2+-polyhedra also tend to be arranged in parallel, further enabling effective superposition of π-conjugated orbitals. In K2Sn2(C2O4)2F2·H2O, there is only one wave-like [Sn2(C2O4)2F2]∞2− chain consisting of alternating linkages of a SnO4F tetragonal pyramid and [C2O4]2− groups named chain A. As expected, all the [C2O4]2− groups in one chain are arranged with near coplanarity, and all of the SnO4F tetragonal pyramids stand neatly. Subsequently, all chains A are arranged strictly parallel in the ac plane (Fig. 3c and d), with the lone pair electrons of Sn2+ and the π-conjugated electrons of the [C2O4]2− group lying in the same plane. Such a regular planar arrangement and uniform orientation result in K2Sn2(C2O4)2F2·H2O exhibiting a large birefringence of 0.301@546 nm, which is approximately 2.9 times larger than that of K2Sn(C2O4)2·H2O. Thus, achieving a parallel and consistent alignment of birefringence-active FMs may be an effective approach to obtain significantly enhanced birefringence.
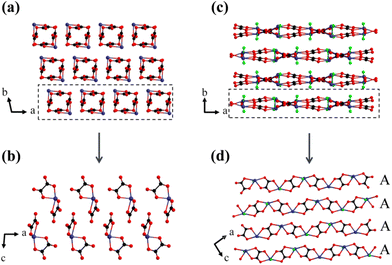 |
| Fig. 3 The different arrangements of Sn2+-[C2O4]2− groups in K2Sn(C2O4)2·H2O (a and b) and K2Sn2(C2O4)2F2·H2O (c and d). | |
In this work, synergetic interactions of Sn2+ cations with SCALP and π-conjugated [C2O4]2− anion groups makes the birefringence vary dramatically from 0.103@546 nm of K2Sn(C2O4)2·H2O to 0.301@546 nm of K2Sn2(C2O4)2F2·H2O based on the structure–property relationship. To investigate in depth the contribution of birefringence-active FMs to the birefringence of the compounds, a detailed analysis of the microscopic mechanism is further conducted. First, the optical properties of all FMs were calculated using the Gaussian 09 package. As shown in Fig. 4a, the results indicate that the polarization anisotropy of the SnO4F tetragonal pyramid is significantly greater than that of the SnO4 seesaw polyhedron as expected. For [C2O4]2− groups with slight flexibility, the dihedral angles between the two [CO2] in one [C2O4]2− group range from 1.512° to 6.736° in the title compounds. Therefore, the small degree of distortion of [C2O4]2− groups has a negligible effect on their optical anisotropy (Table S6†). Obviously, the individual birefringence-active FM with superior polarization anisotropy is not the only essential factor to obtain a large birefringence, since birefringence originates from the effective superposition of microscopic polarization anisotropies of all birefringence-active FMs, which is reflected in their spatial orientation and density. In this work, the calculated refractive index shows that the two compounds are biaxial crystals, and the birefringence can be obtained by Δn = nz − nx. It can be speculated that the larger the birefringence-active FMs acting on the optical principal axis Z or the smaller on the X-axis, the more favorable it is to obtain a large birefringence. Therefore, the relationship between the YZ plane (ac plane for K2Sn(C2O4)2·H2O, ac plane for K2Sn2(C2O4)2F2·H2O) and the orientation of the lone pair electrons in the Sn2+ cations and the C2O42− planes, respectively, is essential for exploring their contribution to birefringence. In Sn2+ cations with a SCALP, the lone pair electron is located on the opposite sides of the vector sum of the all Sn–O and/or Sn–F bonds of Sn2+-based polyhedra, and the angle it forms with the YZ plane is defined as α. Ideally, the lone pair electron would make the largest contribution to the birefringence when α is equal to 0°, i.e., the lone pair electron is completely parallel to the YZ plane. Here, the cos
α values reveal that the contribution of the SnO4 seesaw polyhedron in K2Sn(C2O4)2·H2O to birefringence is significantly smaller than that of the SnO4F tetragonal pyramid in K2Sn2(C2O4)2F2·H2O (Fig. 4b) (Table S7†). In C2O42− groups, the π-conjugated electrons that delocalized over the group plane are substantially responsible for the optical properties, so the anisotropic polarizability is very weak in the out-of-plane direction, especially perpendicular to the C2O42− plane, and strong in the plane direction. Subsequently, the β is adopted to describe the dihedral angle between the C2O42− plane and the YZ plane, which varies abundantly in the two compounds. According to the cos
β values of the two compounds in Fig. 4c, Table S7,† the order of contribution of C2O42− groups to birefringence is completely the same as that of Sn2+-polyhedra. Further considering the density of birefringence-active FMs in a unit cell, the contributions of π-conjugated C2O42− anion groups and Sn2+-based polyhedra with SCALP to birefringence exhibit corresponding increasing trends of 0.0047 < 0.0066 and 0.0030 < 0.0067 in K2Sn(C2O4)2·H2O and K2Sn2(C2O4)2F2·H2O, respectively. Unsurprisingly, the result confirms the previous conclusion that the contribution from the C2O42− anion groups and Sn2+-polyhedra is almost equally responsible for the birefringence of the compounds. Furthermore, the result is consistent with K2Sn2(C2O4)2F2·H2O having a large birefringence, followed by K2Sn(C2O4)2·H2O. Thus, the relationship between birefringence and the spatial arrangement of FMs in this work provides an idea to predict the birefringence of compounds containing lone pair electrons and/or π-conjugated planar groups.
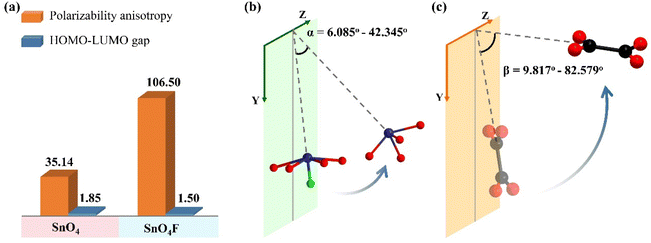 |
| Fig. 4 (a) The polarizability anisotropy and HOMO–LUMO gap of Sn2+-polyhedra (SnO4 for K2Sn(C2O4)2·H2O and SnO4F for K2Sn2(C2O4)2F2·H2O); (b) the angles between the lone pair electron of Sn2+ and the YZ plane; and (c) the dihedral angles between the [C2O4]2− plane and YZ plane in K2Sn(C2O4)2·H2O and K2Sn2(C2O4)2F2·H2O. | |
Theoretical calculations
In order to further understand the structure–property relationship of K2Sn(C2O4)2·H2O and K2Sn2(C2O4)2F2·H2O, the electronic band structure, total and partial density of states (DOS) and electronic density differences were calculated based on the DFT method. The calculated band gaps for K2Sn(C2O4)2·H2O and K2Sn2(C2O4)2F2·H2O are 3.00 eV and 2.29 eV, which are 0.74 eV, and 0.92 eV smaller than the experimental results, respectively (Fig. 5a and d). The reason for the underestimation of the calculated value is usually attributed to the utilization of the DFT-GGA method.57
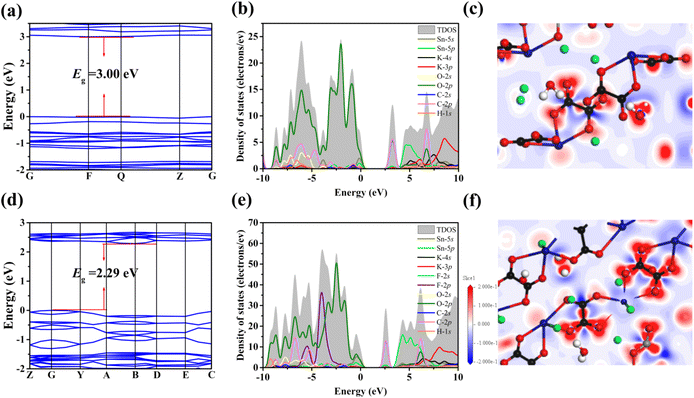 |
| Fig. 5 (a and d) Calculated band structures; (b and e) total and partial density of states; and (c and f) electron density difference maps for K2Sn(C2O4)2·H2O and K2Sn2(C2O4)2F2·H2O. | |
The data of Total Density of States (TDOS) and Partial Density of States (PDOS) for the two title compounds in Fig. 5b and e can prove the contributions of atomic orbitals to the bands. For the valence band (VB) from −10 eV to the Fermi level, the contributions are attributed to the H-1s, C-2s, C-2p, O-2s, O-2p, K-4p, Sn-5s, and Sn-5p states in K2Sn(C2O4)2·H2O and the H-1s, C-2s, C-2p, O-2s, O-2p, F-2p, K-4p, Sn-5s, and Sn-5p states are the main contributors in K2Sn2(C2O4)2F2·H2O. For the conduction band (CB), the H-1s, C-2s, C-2p, O-2p, K-4s, K-4p, Sn-5s, and Sn-5p states are the main contributors in K2Sn(C2O4)2·H2O and K2Sn2(C2O4)2F2·H2O. In partial DOS, for K2Sn(C2O4)2·H2O, it is clearly known that there are overlaps between C-2s, C-2p, Sn-5s, and Sn-5p states and O-2s and O-2p states, indicating the presence of C–O and Sn–O covalent bonds in this compound. For K2Sn2(C2O4)2F2·H2O, the C-2s, C-2p, Sn-5s, and Sn-5p states overlap with the O-2s, O-2p, and F-2p states, illustrating the presence of C–O, Sn–O, and Sn–F covalent bonds in these compounds. For the two compounds, it is quite clear that the electron orbitals near the Fermi level are composed of C-2s, C-2p, Sn-5s, Sn-5p, O-2p (K2Sn(C2O4)2·H2O), C-2s, C-2p, Sn-5s, Sn-5p, O-2p, and F-2p (K2Sn2(C2O4)2F2·H2O), respectively. This shows that the linear optical properties of the two compounds are mainly derived from the synergy of the C2O42− group and Sn2+-based polyhedra. Furthermore, in comparison with K2Sn(C2O4)2·H2O, the birefringence can be further enhanced due to the neat arrangement of the 1D [Sn2(C2O4)2F2]∞2− anionic structure caused by the scissor effect of the F atom with greater electronegativity in K2Sn2(C2O4)2F2·H2O. In the electron density difference maps of the two compounds (Fig. 5c and f), red electronic clouds are observed around the Sn2+ cations and C2O42− planar groups, indicating that the lone pair electrons are stereoactive in Sn2+-polyhedra and the strong interaction between C and O atoms is charge transfer in the C2O42− planar groups. The result further verified that the large birefringence of the two compounds originated from synergetic interactions of the C2O42− groups and the Sn2+ polyhedra.
Conclusions
In brief, K2Sn(C2O4)2·H2O and K2Sn2(C2O4)2F2·H2O were successfully synthesized, in which Sn2+ cations with SCALP and π-conjugated C2O42− anion groups were employed simultaneously to construct a 0D [Sn(C2O4)2]2− anionic structure, and a 1D [Sn2(C2O4)2F2]∞2− anionic structure, respectively. Significantly, with the increase in dimensionality, the birefringence successfully increases from 0.103@546 nm in K2Sn(C2O4)2·H2O to 0.301@546 nm in K2Sn2(C2O4)2F2·H2O. Detailed structural and property analysis confirmed that the rational molecular engineering of the birefringence-active FMs can induce strong optical anisotropy. The significant experimental results provide the prominent UV birefringent crystals and a meaningful strategy to design novel functional materials with large birefringence.
Author contributions
L. Y. Ren: conceptualization, methodology, investigation, writing – original draft, and writing – review & editing. Y. Q. Zhou and D. J. Gao: data curation. L. L. Cao: formal analysis, project administration, resources, and writing – review & editing. L. H. Cheng: characterization analysis. X. Y. Zhou: investigation. J. X. Ren: theoretical calculation. G. H. Zou and L. Huang: resources and supervision. X. H. Dong: conceptualization and investigation.
Conflicts of interest
There are no conflicts to declare.
Acknowledgements
The authors thank Dr Daichuan Ma at Analytical and Testing Center, Sichuan University for technical help in the Material Studio calculations. This work was supported by the National Natural Science Foundation of China (Grant No. 22201195, 22122106, 22071158, and 21971171), Natural Science Foundation of Sichuan Province (2023NSFSC1066) and the Fundamental Research Funds from Sichuan University (2021SCUNL101).
References
- X. L. Chen, B. B. Zhang, F. F. Zhang, Y. Wang, M. Zhang, Z. H. Yang, K. R. Poeppelmeier and S. L. Pan, Designing an excellent deep-ultraviolet birefringent material for light polarization, J. Am. Chem. Soc., 2018, 140, 16311–16319 CrossRef CAS PubMed.
- Z. Y. Xie, L. G. Sun, G. Z. Han and Z. Z. Gu, Optical switching of a birefringent photonic crystal, Adv. Mater., 2008, 20, 3601–3604 CrossRef CAS.
- S. J. Han, C. M. Huang, A. Tudi, S. S. Hu, Z. H. Yang and S. L. Pan,
β-CsB9O14: a triple-layered borate with edge-sharing BO4 tetrahedra exhibiting a short cutoff edge and a large birefringence, Chem. – Eur. J., 2019, 25, 11614–11619 CrossRef CAS PubMed.
- Z. L. Gao, Q. Wu, X. T. Liu, Y. X. Sun and X. T. Tao, Biaxial crystal α-BaTeMo2O9: theory study of large birefringence and wide-band polarized prisms design, Opt. Express, 2015, 23, 3851–3860 CrossRef CAS PubMed.
- G. Q. Zhou, J. Xu, X. D. Chen, H. Y. Zhong, S. T. Wang, K. Xu, P. Z. Deng and F. X. Gan, Growth and spectrum of a novel birefringent α-BaB2O4 crystal, J. Cryst. Growth, 1998, 191, 517–519 CrossRef CAS.
- F. Sedlmeir, R. Zeltner, G. Leuchs and H. G. L. Schwefel, High-Q MgF2 whispering gallery mode resonators for refractometric sensing in aqueous environment, Opt. Express, 2014, 22, 30934–30942 CrossRef CAS PubMed.
- H. T. Luo, T. Tkaczyk, E. L. Dereniak, K. Oka and R. Sampson, High birefringence of the yttrium vanadate crystal in the middle wavelength infrared, Opt. Lett., 2006, 31, 616–618 CrossRef CAS PubMed.
- G. Ghosh, Dispersion-equation coefficients for the refractive index and birefringence of calcite and quartz crystals, Opt. Commun., 1999, 163, 95–102 CrossRef CAS.
- G. Peng, C. S. Lin, H. X. Fan, K. C. Chen, B. X. Li, G. Zhang and N. Ye, Be2(BO3)(IO3): the first anion-mixed van der Waals member in the KBe2BO3F2 family with a very strong second harmonic generation response, Angew. Chem., Int. Ed., 2021, 60, 17415–17418 CrossRef CAS PubMed.
- G. H. Zou, Z. E. Lin, H. M. Zeng, H. Jo, S. J. Lim, T. S. You and K. M. Ok, Cs3VO(O2)2CO3: an exceptionally thermostable carbonatoperoxovanadate with an extremely large second-harmonic generation response, Chem. Sci., 2018, 9, 8957 RSC.
- G. H. Zou, H. Jo, S. J. Lim, T. S. You and K. M. Ok, Rb3VO(O2)2CO3: a four-in-one carbonatoperoxovanadate exhibiting an extremely strong second-harmonic generation response, Angew. Chem., Int. Ed., 2018, 57, 8619–8622 CrossRef CAS PubMed.
- R. K. Li and Y. Y. Ma, Chemical engineering of a birefringent crystal transparent in the deep UV range, CrystEngComm, 2012, 14, 5421–5424 RSC.
- W. J. Xie, Z. Fang and J. G. Mao, Ba6Zn6(B3O6)6(B6O12): barium zinc borate contains π-conjugated [B3O6]3− anions and [B6O12]6− anion with edge-sharing BO4 tetrahedra, Inorg. Chem., 2022, 61, 18260–18266 CrossRef CAS PubMed.
- Z. Li, F. Liang, Y. W. Guo, Z. S. Lin, J. Y. Yao, G. C. Zhang, W. L. Yin, Y. C. Wu and C. T. Chen, Ba2M(C3N3O3)2 (M=Mg, Ca): potential UV birefringent materials with strengthened optical anisotropy originating from the (C3N3O3)3−group, J. Mater. Chem. C, 2018, 6, 12879 RSC.
- L. L. Cao, H. T. Tian, D. H. Lin, C. S. Lin, F. Xu, Y. L. Han, T. Yan, J. D. Chen, B. X. Li, N. Ye and M. Luo, A flexible
functional module to regulate ultraviolet optical nonlinearity for achieving a balance between a second-harmonic generation response and birefringence, Chem. Sci., 2022, 13, 6990 RSC.
- X. P. Shi, W. B. Zhang, W. B. Cai, S. J. Han, Z. H. Yang and S. Pan, Li3La2(BO3)3 and Li1.75Na1.25La2(BO3)3: a great enhancement in birefringence induced by optimal arrangement of π-Conjugated [BO3] Units, Inorg. Chem., 2021, 60, 12565–12572 CrossRef CAS PubMed.
- W. B. Cai, J. Q. Chen, S. L. Pan and Z. H. Yang, Enhancement of band gap and birefringence induced via π-conjugated chromophore with “tail effect”, Inorg. Chem. Front., 2022, 9, 1224–1232 RSC.
- M. Aibibula, L. Wang and S. Z. Huang, Rb3Na(H2C3N3O3)4·3H2O with Large Birefringence, ACS Omega, 2019, 4, 22197–22202 CrossRef CAS PubMed.
- Z. Z. Zhang, Y. Wang, H. Li, Z. H. Yang and S. L. Pan, BaB8O12F2: a promising deep-UV birefringent material, Inorg. Chem. Front., 2019, 6, 546 RSC.
- H. T. Qiu, F. M. Li, C. C. Jin, J. J. Lu, Z. H. Yang, S. L. Pan and M. Mutailipu, (N2H6)[HPO3F]2: maximizing the optical anisotropy of deep-ultraviolet fluorophosphates, Chem. Commun., 2022, 58, 5594 RSC.
- K. C. Chen, C. S. Lin, J. D. Chen, G. S. Yang, H. T. Tian, M. Luo, T. Yan, Z. G. Hu, J. Y. Wang, Y. C. Wu, N. Ye and G. Peng, Intense d-p hybridization in Nb3O15 tripolymer induced the largest second harmonic generation response and birefringence in germanates, Angew. Chem., Int. Ed., 2023, 62, e202217039 CrossRef CAS PubMed.
- J. Moon and K. M. Ok, (R)- and (S)-[C8H10NO3]2[NbOF5]: noncentrosymmetric niobium oxyfluorides with large optical anisotropy, Inorg. Chem. Front., 2022, 9, 2498–2507 RSC.
- M. Aibibula, J. Y. Guo, J. Han, A. Tudi, Z. H. Yang and S. L. Pan, BaTi(BO3)2: an excellent birefringent material with highly coplanar isolated [BO3] groups, New J. Chem., 2021, 45, 7065 RSC.
- J. Y. Guo, A. Tudi, S. J. Han, Z. H. Yang and S. L. Pan, Sn2PO4I: an excellent birefringent material with giant optical anisotropy in non π-conjugated phosphate, Angew. Chem., Int. Ed., 2021, 60, 24901–24904 CrossRef CAS PubMed.
- Y. L. Deng, L. Huang, X. H. Dong, L. Wang, K. M. Ok, H. M. Zeng, Z. E. Lin and G. H. Zou, K2Sb(P2O7)F: cairo pentagonal layer with bifunctional genes reveal optical performance, Angew. Chem., Int. Ed., 2020, 59, 21151–21156 CrossRef CAS PubMed.
- Y. C. Liu, X. M. Liu, S. Liu, Q. R. Ding, Y. Q. Li, L. N. Li, S. G. Zhao, Z. S. Lin, J. H. Luo and M. C. Hong, An unprecedented antimony(III) borate with strong linear and nonlinear optical responses, Angew. Chem., Int. Ed., 2020, 59, 7793–7796 CrossRef CAS PubMed.
- J. Chen, C. L. Hu and J. G. Mao, LiGaF2(IO3)2: a mixed-metal gallium iodate-fluoride with large birefringence and wide band gap, Sci. China Mater., 2021, 64, 400–407 CrossRef CAS.
- S. Y. Niu, G. Joe, H. Zhao, Y. C. Zhou, T. Orvis, H. Huyan, J. Salman, K. Mahalingam, B. Urwin, J. B. Wu, Y. Liu, T. E. Tiwald, S. B. Cronin, B. M. Howe, M. Mecklenburg, R. Haiges, D. J. Singh, H. Wang, M. A. Kats and J. Ravichandran, Giant optical anisotropy in a quasi-one dimensional crysta, Nat. Photonics, 2018, 12, 392–396 CrossRef CAS.
- P. F. Gong, S. Z. Zhang, G. M. Song, X. M. Liu and Z. S. Lin, An unprecedented planar π-conjugated [B2P5]5−group with ultra-large birefringence and nonlinearity: an ab initio study, Chem. Commun., 2020, 56, 643 RSC.
- D. Zhang, Q. Wang, L. Y. Ren, L. L. Cao, L. Huang, D. J. Gao, J. Bi and G. H. Zou, Sharp enhancement of birefringence in antimony oxalates achieved by the cation−anion synergetic interaction strategy, Inorg. Chem., 2022, 61, 12481–12488 CrossRef CAS PubMed.
- G. H. Zou, C. S. Lin, H. Jo, G. Nam, T. S. You and K. M. Ok, Pb2BO3Cl: a tailor-made polar lead borate chloride with very strong second harmonic generation, Angew. Chem., Int. Ed., 2016, 55, 12078–12082 CrossRef CAS PubMed.
- Y. Long, X. H. Dong, H. M. Zeng, Z. E. Lin and G. H. Zou, Layered perovskite-like nitrate Cs2Pb(NO3)2Br2 as a multifunctional optical material, Inorg. Chem., 2022, 61, 4184–4192 CrossRef CAS PubMed.
- Y. X. Chen, Z. X. Chen, Y. Zhou, Y. Q. Li, Y. C. Liu, Q. R. Ding, X. Chen, S. G. Zhao and J. H. Luo, An antimony(III) fluoride oxalate with large birefringence, Chem. – Eur. J., 2021, 27, 4557–4560 CrossRef CAS PubMed.
- Y. W. Ge, Q. Wang, F. Yang, L. Huang, D. J. Gao, J. Bi and G. H. Zou, Tin chloride sulfates A3Sn2(SO4)3−xCl1+2x (A=K, Rb, Cs; x=0, 1) as multifunctional optical materials, Inorg. Chem., 2021, 60, 8322–8330 CrossRef CAS PubMed.
- H. X. Qi, H. Jo, X. L. Chen, J. Hong and K. M. Ok, Second-harmonic generation and photoluminescence properties of Sn(II)- and Bi(III)-based lone pair cation-pyridine dicarboxylate coordination compounds, Inorg. Chem., 2020, 59, 11554–11561 CrossRef CAS PubMed.
- R. A. Li, Z. Y. Zhou, Y. K. Lian, F. Jia, X. X. Jiang, M. C. Tang, L. M. Wu, J. L. Sun and L. Chen, A2SnS5: a structural incommensurate modulation exhibiting strong second-harmonic generation and a high laser-induced damage threshold (A=Ba, Sr), Angew. Chem., Int. Ed., 2020, 59, 11861–11865 CrossRef CAS PubMed.
- J. Y. Guo, A. Tudi, S. J. Han, Z. H. Yang and S. L. Pan, Sn2B5O9Cl: a material with large birefringence enhancement activated prepared via alkaline-earth-metal substitution by Tin, Angew. Chem., Int. Ed., 2019, 58, 17675–17678 CrossRef CAS PubMed.
- J. Y. Guo, A. Tudi, S. J. Han, Z. H. Yang and S. L. Pan,
α-SnF2: a UV birefringent material with large birefringence and easy crystal growth, Angew. Chem., Int. Ed., 2021, 60, 3540–3544 CrossRef CAS PubMed.
- M. J. Schäfer, S. G. Jantz, F. Pielnhofer and H. A. Höppe, The very first normal-pressure tin borate Sn3B4O9, and the intermediate Sn2[B7O12]F, Dalton Trans., 2019, 48, 10398 RSC.
- T. H. Tong, W. Y. Zhang, Z. H. Yang and S. L. Pan, Series of crystals with giant optical anisotropy: a targeted strategic research, Angew. Chem., Int. Ed., 2021, 60, 1332–1338 CrossRef CAS PubMed.
- D. Zhang, Q. Wang, T. Zheng, L. L. Cao, K. M. Ok, D. J. Gao, J. Bi, L. Huang and G. H. Zou, Cation-anion synergetic interactions achieving tunable birefringence in quasi-one-dimensional antimony(III) fluoride oxalates, Sci. China Mater., 2022, 65, 3115–3124 CrossRef CAS.
- G. M. Sheldrick, A short history of SHELX, Acta Crystallogr., Sect. A: Found. Crystallogr., 2008, 64, 112–122 CrossRef CAS PubMed.
- A. L. Spek, Single-crystal structure validation with the program PLATON, J. Appl. Crystallogr., 2003, 36, 7–13 CrossRef CAS.
- S. J. Clark, M. D. Segall, C. J. Pickard, P. J. Hasnip, M. I. J. Probert, K. Refson and M. C. Payne, First principles methods using CASTEP, Z. Kristallogr. - Cryst. Mater., 2005, 220, 567–570 CrossRef CAS.
- M. D. Segall, P. J. D. Lindan, M. J. Probert, C. J. Pickard, P. J. Hasnip, S. J. Clark and M. C. Payne, First-principles simulation: ideas, illustrations and the CASTEP code, J. Phys.: Condens. Matter, 2002, 14, 2717–2744 CrossRef CAS.
- D. Vanderbilt, Soft self-consistent pseudopotentials in a generalized eigenvalue formalism, Phys. Rev. B: Condens. Matter Mater. Phys., 1990, 41, 7892 CrossRef PubMed.
- K. Kobayashi, Norm-conserving pseudopotential database (NCPS97), Comput. Mater. Sci., 1999, 14, 72–76 CrossRef.
- A. D. Christie, R. A. Howie and W. Moser, Tin(II) oxalate structures, Inorg. Chim. Acta, 1979, 36, L447–L448 CrossRef CAS.
- L. D. Luan, J. Li, C. Chen, Z. E. Lin and H. Huang, Solvent-free synthesis of crystalline metal phosphate oxalates with a (4,6)-connected fsh topology, Inorg. Chem., 2015, 54, 9387–9389 CrossRef CAS PubMed.
- Y. V. Kokunov, D. G. Detkov, Y. E. Gorbunova, M. M. Ershova, Y. N. Mikhailov and Y. A. Buslaev, Crystal structure of (NH4)2Sn2F4(NO3)2: the first example of dimeric [Sn2F4E2] complexes in Tin(II) fluorides, Dokl. Chem., 2001, 378, 135–138 CrossRef.
- Y. V. Kokunov, D. G. Detkov, Y. E. Gorbunova, M. M. Ershova and Y. N. Mikhailov, Synthesis and crystal structure of calcium trifluorostannate(II), Dokl. Chem., 2001, 376, 52–54 CrossRef.
- D. E. Zelmon, D. L. Small and D. Jundt, Infrared corrected Sellmeier coefficients for congruently grown lithium niobate and 5 mol% magnesium oxide–doped lithium niobate, J. Opt. Soc. Am., 1997, 14, 3319–3322 CrossRef CAS.
- J. R. Devore, Refractive indices of rutile and sphalerite, J. Opt. Soc. Am., 1951, 41, 416–419 CrossRef CAS.
- Y. X. Chen, T. T. Zhu, Z. Y. Xiong, Y. Zhou, Y. Q. Li, Q. R. Ding, Y. C. Liu, X. Chen, S. G. Zhao and J. H. Luo, An organic-inorganic hybrid birefringent material with diverse functional groups, Chem. Commun., 2021, 57, 6668 RSC.
- R. E. Dinnebier, S. Vensky, M. Jansen and J. C. Hanson, Crystal structures and topological aspects of the high-temperature phases and decomposition products of the alkali-metal oxalates M2[C2O4] (M=K, Rb, Cs), Chem. – Eur. J., 2005, 11, 1119–1129 CrossRef CAS PubMed.
- D. Zhang, Q. Wang, H. Luo, L. L. Cao, X. H. Dong, L. Huang, D. J. Gao and G. H. Zou, Deep eutectic solvents synthesis of A2Sb(C2O4)Cl3 (A=NH4, K, Rb) with superior optical performance, Adv. Opt. Mater., 2023, 11, 2202874 CrossRef CAS.
- J. L. Song, C. L. Hu, X. Xu, F. Kong and J. G. Mao, A facile synthetic route to a new SHG material with two types of parallel π-conjugated planar triangular units, Angew. Chem., Int. Ed., 2015, 54, 3679–3682 CrossRef CAS PubMed.
Footnote |
† Electronic supplementary information (ESI) available: Additional crystallographic data, XRD patterns, IR spectra, TGA, the residue of TGA and UV-vis-NIR diffuse reflectance spectra of K2Sn(C2O4)2·H2O and K2Sn2(C2O4)2F2·H2O. CCDC 2267040 for K2Sn(C2O4)2·H2O and 2267041 for K2Sn2(C2O4)2F2·H2O. For ESI and crystallographic data in CIF or other electronic format see DOI: https://doi.org/10.1039/d3qi01213a |
|
This journal is © the Partner Organisations 2023 |