DOI:
10.1039/C9TA06534B
(Review Article)
J. Mater. Chem. A, 2019,
7, 23537-23562
2D molecular crystal lattices: advances in their synthesis, characterization, and application
Received
18th June 2019
, Accepted 22nd August 2019
First published on 22nd August 2019
Abstract
This review identifies how recent advances in controlling the structure and properties of MOF and COF thin films can be used to establish 2D molecular crystals as a new material platform with key applications in catalysis, sensing, separations, and electronics. In addition to summarizing research efforts surrounding the synthesis, characterization and utilization of 2D and thin film MOFs and COFs, this review identifies exigent challenges in their development, and motivates the search for new synthetic methods, advanced analytical techniques, and creative applications. Ultimately, 2D molecular lattices, be they 2D MOFs or COFs, are a compelling platform through which to discover new and emergent properties and to expand the library of 2D materials.
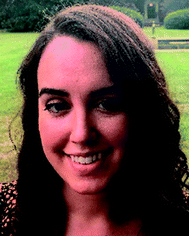 Marina A. Solomos | Marina Solomos earned her Ph.D. in 2018 from Georgetown University under the supervision of Prof. Jennifer A. Swift, where her research focused on controlling the crystallization behavior of polymorphic small molecules using functionalized templates and co-crystallization methods. In March 2018, she began her postdoctoral training at Johns Hopkins University with Prof. Thomas J. Kempa. Her current research explores vapor phase growth of coordination polymers for the development of responsive devices and sensors. |
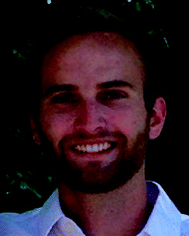 F. James Claire | Jamie Claire received his B.S. in Chemistry from the College of Charleston in 2016. He is a Ph.D. candidate at Johns Hopkins University under the supervision of Prof. Thomas J. Kempa. His research focuses on the synthesis of responsive one- and two-dimensional metal–organic frameworks through solution and gas-phase methods and the investigation of their physical properties. |
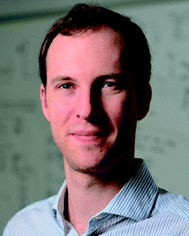 Thomas J. Kempa | Thomas (Tom) Kempa completed his Ph.D. studies in 2012 under the supervision of Prof. Charles M. Lieber at Harvard University. He then completed a post-doctoral fellowship with Prof. Daniel G. Nocera at MIT and Harvard. In July 2015, Tom joined the faculty of the Department of Chemistry at Johns Hopkins University as an Assistant Professor. Since 2017 he has held a joint appointment in the Department of Materials Science and Engineering. The Kempa group synthesizes and studies materials with novel structures, phases, and topologies and is currently focused on porous molecular solids, 2D materials, and multi-component nanostructures. These materials have unique properties rendering them useful for addressing outstanding challenges in fundamental science, energy sustainability, optoelectronics, and quantum information science. The Kempa group's expertise spans the areas of physical, inorganic, and materials chemistry. Tom has been the recipient of several grants and accolades including an NSF CAREER Award, a Toshiba Distinguished Young Investigator Award, and a Dreyfus Foundation Fellowship in Environmental Chemistry. |
1. Introduction
To appreciate the emerging opportunities in the study of two-dimensional (2D) molecular crystal lattices, one need only to look at the massive attention dedicated over the past 10 years to 2D atomic crystals. Graphene, graphene analogs of the group IV elements, and single-layer transition metal dichalcogenides are 2D atomic crystals that exhibit in-plane covalent bonding, out-of-plane van der Waals interactions with their surroundings, and electronic and optical properties that are distinct from those exhibited by their bulk counterparts.1–7 These materials are frequently classified as van der Waals solids and can be combined with one another to yield so-called van der Waals heterostructures encompassing expanded or hybrid properties.8
In this vein, 2D metal organic frameworks (MOFs) and covalent organic frameworks (COFs) are a compelling platform on which to discover new emergent properties and expand the library of 2D materials (Table 1).9–14 Two-dimensional molecular crystals can be synthetically designed and subsequently tuned to target a multitude of desirable material properties.15–18 Porous by nature, 2D MOFs and COFs offer higher surface areas than conventional dense solids, heightened guest capture19 and sensing20 abilities, new charge transport modalities,21 and improved catalytic performance.22,23 Moreover, the extensive internal surfaces within MOFs and COFs can be post-synthetically modified to introduce additional tailorable functionalities.15,24–27 Notably, the reduced dimensionality, the access to diverse bonding motifs, and the tunable symmetry and size of unit cells within 2D molecular lattices raises the tantalizing prospect for design and discovery of new properties that could be leveraged to create a new generation of optoelectronic devices.28–31
Table 1 Summary of salient characteristics of 2D materials. Graphene scheme was adapted from ref. 32 and 2D transition metal dichalcogenide image is courtesy of Wang et al., MIT.33 2D MOF scheme was adapted from ref. 34. COF image adapted with permission.205 Copyright 2017 American Chemical Society
The appeal of 2D MOFs and COFs, molecular nanosheets, and crystalline thin films is well established throughout the solid-state community, but significant challenges remain.16,22,29,35–40 Past and current efforts consistently emphasize the need for improved thin film fabrication techniques. These methods must yield high-quality materials with minimal crystallographic defects and uniform surfaces, and must exert precise control over their dimensions.41,42 Ideally, these methods should be scalable, facile, and cost-efficient to facilitate large-scale production of 2D molecular crystals. High-quality films of MOFs and COFs are defined as those having strong substrate interactions, uniform pore orientation, and well-defined layers.43,44 In addition to these requirements, the preparation of singly- or highly-crystalline thin films over large areas is necessary for the integration of 2D molecular crystals into devices and other architectures.43,45 New molecular systems and synthetic methods, or adaptations of existing synthetic methods, as well as new characterization techniques, are required to accomplish these goals.23
This review identifies how recent advances in controlling the structure and properties of MOF and COF thin films can be used to establish 2D molecular crystals as a new material platform with key applications in catalysis, sensing, separations, and electronics. Though many methods exist for the growth of polycrystalline, micrometer-thick MOF or COF films, to-date very few methods can grow high-quality crystalline thin films approaching the single-layer limit. Standard preparative routes target anisotropic crystal dimensions by inhibiting MOF or COF layer assembly (e.g. through interfacial synthesis and surfactant assisted growth), while substrate-directed growth methods rely on surface functionalization or patterning to template MOF and COF thin films as they grow layer-by-layer.44,46,47 These “top-down” and “bottom-up” approaches have, in turn, led to a variety of promising techniques that improve thin film crystallinity and approach monolayer thicknesses by targeting the nucleation of molecular crystals. As the quality of 2D molecular crystals and thin films improves, their incorporation into multifunctional materials will be realized. The aim of this review is to summarize the state of research in 2D or near-2D MOFs and COFs, identify exigent challenges in their development, and motivate the search for new synthetic methods, characterization techniques, and creative applications.
2. Synthetic advances
The fabrication of molecular nanosheets and thin films can be accomplished using either solution- or vapor-phase techniques. While preparative methods for nanosheets and thin films share some common features, it is important to understand the differences between the two material forms and how these differences influence their synthesis and utilization. Thin films range in thickness from several nanometers to micrometers and can be phase pure, layered, or mixed materials comprised of oriented or non-oriented crystalline grains. Preparing thin films of MOFs or COFs frequently involves interfacial reactions of separate monomer solutions or vacuum-assisted filtration of reacting components to assemble a compacted disk. In contrast, nanosheets are typically 2D monolayer or few-layer nanoscale lattices that can function as stand-alone materials or as components of a larger thin film or membrane.22,48–50 The preparation of nanosheets may also be accomplished through interfacial, and other, reactions, but may also use techniques that limit the assembly of bulk MOF or COF crystals after nucleation. In this review, we include discussion of both nanosheet and thin film morphologies to emphasize the assorted applications of MOFs and COFs as they approach the scale of 2D materials.
When considering nanosheets and thin films of MOFs and COFs, optimized growth conditions specific to each unique framework are required. Though it is exceptionally challenging to prepare defect-free materials, the synthetic methods discussed herein offer diverse approaches for the formation of ordered MOFs and COFs as they approach the 2D scale and in many cases provide improved ordering compared to bulk growth methods. MOFs, assembled from metal nodes and organic linkers, can adopt 2D or 3D lattices and may require more carefully controlled coordination than COFs. As such, techniques that facilitate careful assembly of metals and linkers are better suited for MOF nanosheet and thin film growth, whereas the inherent 2D layering of COFs more easily allows for the manipulation of bulk phases towards 2D materials. MOF growth exploits slow diffusion of reactants, longer nucleation and growth times, higher boiling point solvents, and templating substrates.51–53 COFs form 2D lattices from planar organic linkers motivating the use of supramolecular design to introduce stronger layer interactions that lead to growth of high quality material.54 COF growth can also be improved by simply altering modulator and reactant concentrations during assembly.55
2.1 Solution-phase methods
Solution-based synthetic methods for the preparation of crystalline thin films of molecular systems are well established. Bulk MOFs and COFs can be readily synthesized through straightforward mixing of monomer components in solution. MOF and COF crystal quality is closely dependent on the solvent used, the amount of heat introduced if using a solvothermal process, and the concentration of added monomers or modulators. Two-dimensional MOF or COF nanosheets are frequently synthesized from solution by adapting or expanding upon traditional solvothermal strategies employed for preparation of the corresponding bulk phases. As is the case during preparation of 2D atomic crystals, exfoliation of MOF or COF crystals is able to disrupt van der Waals contact forces and yield a layered lattice. However, gentler, solution-based exfoliation methods are often required to prevent substantial damage to the framework's crystalline structure. In many cases, the layered structure of MOFs lends itself well to simple sonication delamination.56,57
Banerjee et al.36 synthesized two new bulk samples of imide-based COFs through standard solvothermal methods and subsequently sonicated these in isopropyl alcohol for 45–60 minutes. Centrifugation and drying of the collected material yielded covalent organic nanosheets with heights ranging between 1.5–5.1 nm, which corresponded to 5–15 COF layers. Initial wet ball-milling of bulk MOF crystals in methanol and propanol at slow speeds was shown by Yang et al.23 to facilitate methanol penetration between constituent Zn2(bim)4 layers and to stabilize the resultant nanosheets prior to further exfoliation by sonication (Fig. 1a). The nanosheets have uniform thicknesses and are reported to be as thin as 1.12 nm, which is consistent with a monolayer. Sun and co-workers58 recently reported a facile method for sonication exfoliation of a cobalt and pyrazine based MOF, Co(CNS)2(pyz)2, in ethanol for 30 minutes. The product was collected by rotary evaporation and was found to consist of monolayer nanosheets, which exhibited solvatochromic behavior and were subsequently implemented as solvent polarity sensors.
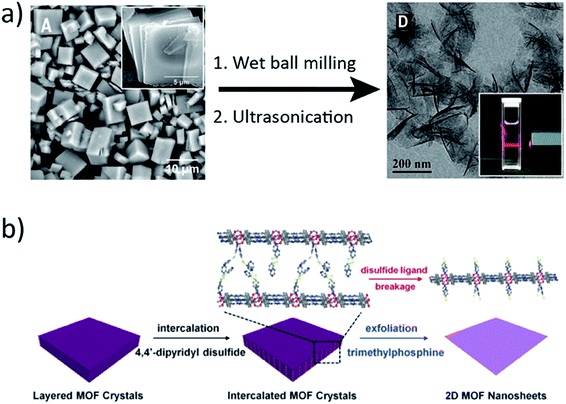 |
| Fig. 1 (a) SEM image of as-synthesized Zn2(bim)4 crystals (left) which undergo wet ball-milling and ultrasonication to exfoliate single layers. TEM image of exfoliated nanosheets (right). The inset shows Tyndall scattering of the colloidal solution of nanosheets. (b) Schematic representation of the chemical exfoliation process using 4,4′-dipyridyl disulfide to produce single MOF nanosheets. Reprinted with permission from AAAS.23 Adapted with permission.59 Copyright 2017 American Chemical Society. | |
Chemical exfoliation methods have also been used to disrupt layered frameworks. PPF-1 MOF crystals, comprised of porphyrin sheets assembled from zinc paddlewheels, were exposed to 4,4′-dipyridyl disulfide to form a new, intercalated 3D MOF. Selective reduction of the disulfide bond by trimethylphosphine yielded ∼1 nm thick MOF nanosheets. Zhou et al.59 compared their method to surfactant-assisted exfoliation and found chemical exfoliation to be better suited for extracting single layer nanosheets (Fig. 1b). Similarly, Zhang et al.60 designed a layered MOF incorporating 2,3-dihydroxy-1,4-benzenedicarboxylic acid as a redox-active pillar ligand. Selective oxidation of the pillar ligands realized conversion of the 3D MOF to ultrathin 2D nanosheets (∼2 nm thick) in O2 saturated electrolyte solutions. Delamination of Cu2(bdc)2 MOF crystals in oleylamine was demonstrated by Kaskel and coworkers61 to yield ∼70 nm thick sheets. The authors found that addition of polyvinylpyrrolidone, PVP, during crystallization of the parent Cu2(bdc)2 bulk phase yielded thinner (4–14 nm) 2D MOF sheets after exfoliation in oleylamine. Foster et al.62 use a strategy of chemical design and ultrasonic exfoliation to prepare copper-based MOF nanosheets. They installed hydrophobic or hydrophilic pendant ligands in the parent bulk MOF phase and attempted exfoliation in solvents of ranging polarities. Sonication in acetonitrile for 12 hours yielded nanosheets as thin as 2–10 nm, and the authors found that nanosheet dimensions could be tuned through judicious choice of exfoliation solvent, sonication duration, and centrifugation time. COF nanosheets have also been produced by chemical exfoliation.63–65 Banerjee and coworkers66 combined post-synthetic modifications with a Diels Alder reaction to convert their anthracene-based COF to N-hexylmaleimide functionalized nanosheets, which were subsequently used to prepare thin films at an air–water interface.
Interfacial syntheses using solution phases of metal and/or linkers are another route towards the preparation of nanosheets and include strategies such as direct mixing, liquid–liquid layering, or three liquid layering processes. Inspiration for these strategies comes from success in preparing large area MOF and COF films of controlled thickness at vapor–liquid interfaces.67,68 Tsapatsis et al.69 showed that Cu(BDC) nanosheet formation is possible by mixing of copper(II) nitrate trihydrate in DMF and acetonitrile with a 1,4-benzenedicarboxylic acid solution. Either no mixing or gentle agitation formed ∼25 nm thick Cu(BDC) nanosheets, which were readily collected by centrifugation. These nanosheets were then incorporated into mixed-matrix membranes, which exhibited an increased matrix selectivity for CO2/CH4. Thicker Cu(BHT) films were prepared by Zhu et al.70 using a liquid–liquid interface reaction between aqueous copper(II) nitrate and benzenehexathiol in dichloromethane. The films, which can be clearly identified as forming at the interface, range in thickness from 20–140 nm, with thicker films exhibiting increased surface roughness. Films of 15–500 nm thickness were transferred to substrates and exhibited record conductivities ranging from 750–1580 S cm−1. Comparable layering of monomer solutions was shown to prepare COF nanosheets as thin as 0.35 nm by Ma and coworkers.71 Feng et al.72 demonstrated the versatility of liquid–liquid interfacial synthesis by showing that the method could be used to prepare large-area thin films of a supramolecular organic framework prepared from truxene, 6-methoxynaphthalene, N-methyl-4,4′-bipyridin-1-ium, naphthalene diimide, and cucurbit[8]uril. After a two hour assembly period in a custom-made glass trough, which facilitated layering of precursors, 1.8 nm thick monolayer sheets were isolated and transferred to Si/SiO2 substrates. In recent work, interfacial synthesis has been used to prepare polyCOFs, covalent organic frameworks that incorporate linear polymers for heightened flexibility and lessened internal defects.73 Zhang et al. prepared a water-soluble polymer that was mixed and layered with organic linkers and acetic acid catalyst, forming a free-standing membrane as thin as 4.5 nm.
Additional COF thin films were prepared by Dichtel et al.74 through a process that involved first mixing all required monomers in a single organic phase and then layering with Sc(OTf)3 catalyst in an aqueous phase. The resulting COF films covered a large area and had thickness ranging from 2.5 nm to 100 μm (Fig. 2). The authors found that film thickness could be controlled by changing monomer concentration, with low concentrations yielding thinner, less crystalline films. The COF films were used as the active layer in nanofiltration membranes, which were tested for their efficacy with micropollutant model compounds. Using a three-layer approach, Gascon et al.75 demonstrated the formation of 5–25 nm thick Cu(BDC) nanosheets by separating layered solutions of the copper(II) nitrate and 1,4-benzenedicarboxylic acid precursors with an intermediate solvent layer. Slow diffusion of metal ions and BDC linker molecules forms MOF films within the intermediate layer. In yet another adaptation of liquid–liquid interfacial reactions, Su et al.76 formed large area thin films of two new MOFs, Au3BHT2 and Ag3BHT2, by forming an organic solvent–water interface in a large dish with a SiO2-coated silicon wafer resting at the bottom. Slow injection of a metal ion solution into the organic layer facilitated the formation of thin films of Ag3BHT2 and Au3BHT2 in 30 min and 6.5 hours, respectively. The MOF thin films were deposited onto the SiO2 substrate through careful removal of the solvent. Though the films are several hundreds of nanometers thick and contain defects, the Ag-containing MOF exhibited a significant conductivity of 363 S cm−1.
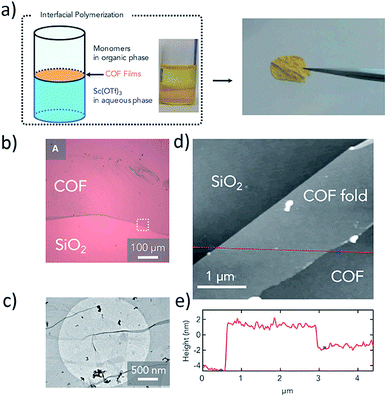 |
| Fig. 2 (a) Schematic and photograph of liquid–liquid interfacial synthesis process (left) used to prepare TAPB-PDA COF and the resulting free-standing film (right). (b) Optical and (c) TEM micrographs of TAPB-PDA COF transferred onto Si/SiO2 substrate and TEM carbon grids, respectively. (d) AFM image (white box from (b)) of isolated monolayer sheets and (e) height profile along the red line in (d). Adapted with permission from Elsevier.74 | |
As seen in the examples above, interfacial synthesis is effective at limiting growth of molecular materials perpendicular to the interface and is therefore commonly used to prepare 2D MOF materials. The incorporation of a surfactant during nucleation and growth of MOFs has also been shown to be effective in encouraging the formation of nanosheets. Zhang et al.77 developed a surfactant-assisted method for the formation of 2D Zn-TCPP MOF nanosheets. When introduced as a reagent during conventional MOF synthesis, polyvinylpyrrolidone (PVP) selectively attaches to zinc cations on the MOF surface and leads to anisotropic growth. Zn-TCPP nanosheets 7.6 ± 2.6 nm thick were achieved and correspond to 8 ± 3 layers of Zn-TCPP per nanosheet. Tsapatsis et al.78 also demonstrated the utility of surfactant incorporation during MOF synthesis by mixing a metal precursor and ligand in a suspension of cetyltrimethylammonium bromide, CTAB, in heptane and hexanol. The selective binding of CTAB to the major crystal planes of Zn(Bim)OAc in conjunction with optimization of temperature conditions facilitates the formation of surfactant-free MOF nanosheets with 7 nm thicknesses (Fig. 3).
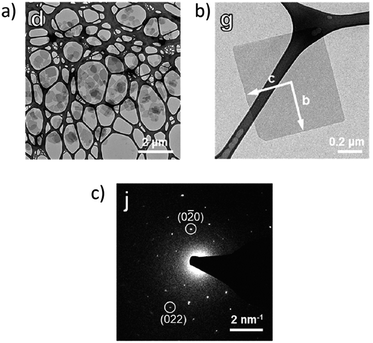 |
| Fig. 3 TEM images of (a) several nanocrystals and (b) a single nanocrystal of Zn(Bim)OAc synthesized in a suspension of CTAB in heptane and hexanol at 25 °C. (c) Corresponding electron diffraction pattern of a single nanocrystal. Adapted with permission.78 Copyright 2018 American Chemical Society. | |
To enable their eventual incorporation into more functional materials and devices, MOF nanosheets must be either transferred to or selectively grown on substrates. Adapting the layer-by-layer process (LbL), Kitagawa et al.79 utilized standard solvothermal techniques to prepare nanoflakes of a range of MOFs. In one example, the authors prepared Cu-TCPP nanoflakes, approximately 15 nm or 33 porphyrin layers in thickness, suspended them in ethanol, and then added them dropwise to the surface of water, thereby assembling a thin film which could be transferred to assorted substrates through a simple stamping process. Characterization of the thin film revealed that it has a crystallinity and orientation similar to that of the SURMOF bulk phase. Coronado et al.80 prepared MOF thin films as thin as 10 nm by dipping functionalized substrates (silicon, gold, and permalloy) into a CoTCPP-pyridine solution, which had been dispersed over a copper(II) nitrate solution. The functionalized substrates successfully directed the transfer of MOF nanosheets onto suitable interfaces for device integration, whereas bare substrates exhibited poor or failed transfer.
As an alternative to the transfer of MOF films onto substrates, MOF or COF thin films can be directly grown on selected regions of a substrate if the desired areas are pre-functionalized with self-assembled monolayers (SAMs).81,82 Wöll et al. demonstrated the utility of functionalized substrates for the bottom-up assembly of MOF thin films.44,83–85 Gold surfaces functionalized with 11-mercapto-1-undecanol were subjected to sequential, layer-by-layer (LbL) depositions of metal and ligand solutions (liquid phase epitaxy), with growth cycles monitored by a quartz crystal microbalance (QCM). Resultant MOF films were 64–80 nm thick and were intentionally loaded with assorted metal ions. Further work on carboxylate-functionalized Au surfaces showed that MOF thin films with similar thicknesses can be prepared by spray coating metal and ligand solutions sequentially. Performing LbL growth on QCMs, Allendorf et al. have shown that both functionalized semiconductor surfaces86,87 and patterned substrates88 are able to facilitate growth of two or three unit cell thick, stacked crystalline MOF sheets of HKUST-1. While monitoring the MOF assembly process with QCMs, Fischer and coworkers89 alternately deposited Zn4O(OAc)6 solution and various 3,5-dialkyl-4-carboxypyrazolate linkers onto functionalized gold sensors to form crystalline thin films of [Zn4O]6+ MOFs. The influence of acetic acid modulators, which are added to either the metal or ligand solutions prior to LbL assembly, on the crystallinity of the thin films was assessed. Marks, Stoddart, and colleagues90 prepared gamma-cyclodextrin (CD) self-assembled monolayers to facilitate the epitaxial growth of CD-MOF polycrystalline thin films on glass substrates. The large-area surface coverage and oriented growth (2 μm) of these films allowed for their direct incorporation into a functional device with enhanced proton conductance and CO2 sensing abilities. Hupp91 also prepared surface-supported, patterned films of ZIF-8 for the formation of hybrid materials with vapor sensing capabilities.
Unfunctionalized substrates can still aid in the formation of well-adhered MOF thin films by acting as metal ion sources in the presence of organic linkers and oxidizing or deprotonating agents. Jeong et al.92 showed that copper and zinc substrates are able to seed the growth of HKUST-1 and ZIF-8, respectively. The HKUST-1 film thus prepared exhibited an increased conductance in the presence of I2 suggesting its utility as a sensor. Interestingly, graphene oxide has been shown as a viable template for MOF growth, binding HKUST-1 and facilitating continuous thin film growth via a rod-like intermediate.93 Kunitake and coworkers94 showed that graphite substrates facilitated the formation of large nanosheets of Cu-H2TCPP with sub-1 nm thicknesses in the presence of acetic acid vapor.
A number of strategies have been explored to streamline and industrialize MOF growth processes using automated deposition methods such as spray coating or spin coating. Behrens and co-workers95 demonstrated the use of ammonia modulation to prepare nanoflakes of Cu3HTTP2 that were subsequently deposited manually/automatically onto substrates by spray-coating to create homogenous oriented films, which could serve as protective coatings. Eddaoudi et al.96 developed a spin coating method for the preparation of thin films of assorted MOFs by multilayer deposition. Their apparatus consisted of four micro-syringes capable of depositing metal ion and linker solutions onto rotating substrates to produce uniform thin films ranging in thickness from several nm to μm (Fig. 4). Farha and Hupp97 precisely controlled the thickness of their MOF films by alternately soaking amino-functionalized silicon substrates in separate solutions containing Zn2+ ions and different ligands. The process was automated to enable precise control over the number of cycles and likewise the film thickness. Thomas et al.98 developed a flow synthesis method that confines the reaction of MOF precursor solutions within droplets, which form upon injecting the solutions into an oil phase. The droplets flow through a heated microchannel, which also prevents layered growth of the MOF crystals, at the end of which ultrathin 2D nanosheets of ZrBTB MOFs are collected. Height profiles reveal the sheets to be as thin as 3–4 nm, corresponding to 3–4 crystallographic layers.
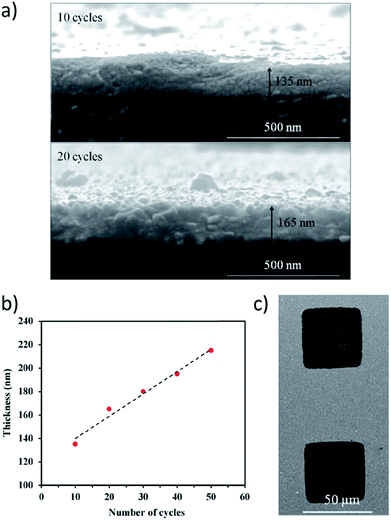 |
| Fig. 4 (a) SEM images of the cross-section of Cu2(bdc)2·xH2O MOF thin films deposited using the four micro-syringe spin-coating method after 10 (top) and 20 (bottom) cycles. (b) Height profiles of thin films produced after 10 to 50 cycles. (c) Substrate-selective growth of MOF on patterned substrates during the spin-coating process. MOF thin films only form within the square patterned regions having COOH-terminated surfaces. Deposition on CH3-terminated areas of the substrate is negligible. Adapted with permission.96 Copyright 2016 American Chemical Society. | |
Substrate-mediated techniques have demonstrated their efficacy for high quality growth of molecular thin films, but more recent solution-based methods focus on adjusting the nucleation kinetics of MOFs and COFs in an effort to control crystal growth. Dichtel and colleagues have recently shown that colloidal suspensions of COF-5 seed crystals can grow larger, high quality single crystals when monomer addition takes place slowly.99 In related work, Dichtel et al. also showed that COF-5 thin films could be controllably prepared in a flow cell.100 Monomers added to a small volume of methanol within their flow cell apparatus formed COF-5 thin films within minutes. The films exhibit minimal contamination with bulk aggregates and their thickness and crystallinity can be controlled by the residence time of the monomer within the apparatus, the monomer concentration, and the reaction temperature. Yaghi et al.101 employed an in situ deprotection step to better control the nucleation stage of imine-based COFs, such as LZU-1. This process favored the nucleation of oriented nanocrystals on silicon substrates and formed thin films, which were approximately 190 nm thick (Fig. 5).
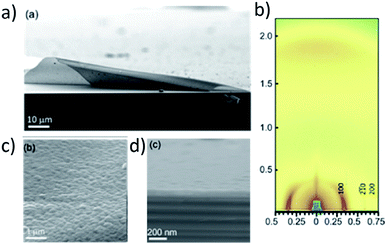 |
| Fig. 5 (a) Highly uniform thin film of LZU-1 synthesized with in situ deprotection. (b) The crystallinity and orientation are confirmed by GIWAXS. (c) The thin film is composed of small crystallites with similar orientation and is 190 nm thick as measured by (d) cross-sectional SEM. Adapted with permission.101 Copyright 2017 American Chemical Society. | |
2.2 Vapor-phase methods
Though preferred by most researchers, solution-based growth struggles to afford a high degree of control over thin film quality just as solvent-based bulk recrystallization of MOFs and COFs remains a perennial challenge. Numerous problems conspire against the formation of consistently high-quality MOF and COF thin films, including the formation of defects, polycrystalline domains, metastable phases, and macroscale cracks. To address many of these concerns, as well as eliminate unwanted solvent effects, the preparation of thin film molecular crystals via gas phase methods has recently gained attention as a promising approach to the deposition of uniform layers of molecular materials with control of thickness. In combination with patterned templates or functionalized substrates, the discovery of molecular framework systems capable of forming ordered crystalline thin films from the gas-phase has the potential to transform 2D molecular crystal studies.
Just as atomic layer deposition (ALD) and chemical vapor deposition (CVD) are commonly used for the synthesis of 2D materials,102,103 molecular layer deposition (MLD) and CVD are now becoming attractive candidates for the preparation of high-quality crystalline MOF and COF thin films.104–107 Ritala et al. prepared MOF-5 thin films by ALD108 through iterative application of zinc acetate and 1,4-benzenedicarboxylic acid precursor pulses within a hot wall reactor. The initially deposited amorphous material was transformed into MOF-5 upon water vapor exposure. Ameloot and coworkers have further demonstrated the promise of solvent-free preparative routes. They showed that heating a zinc oxide film covered with a thin layer of 2-methylimidzaole to 433 K produces ZIF-8 within one minute.109 More recently, Ameloot et al. used CVD110 to deposit zinc oxide on a range of flat and textured substrates, and then treated these substrates with 2-methylimidazole vapor under ALD conditions (Fig. 6a and b). This two-step process yielded continuous 52–104 nm thick ZIF-8 polycrystalline films (Fig. 6c). Boroxine COF monolayers were prepared by Lackinger et al. through sublimation under ultra-high vacuum conditions.111 Characterization with STM revealed hexagonal networks and lattice parameters in good agreement with COF monolayers. The modification of larger precursors was occasionally required to facilitate sublimation and, in some instances, exposure to water vapor was needed to induce COF formation.
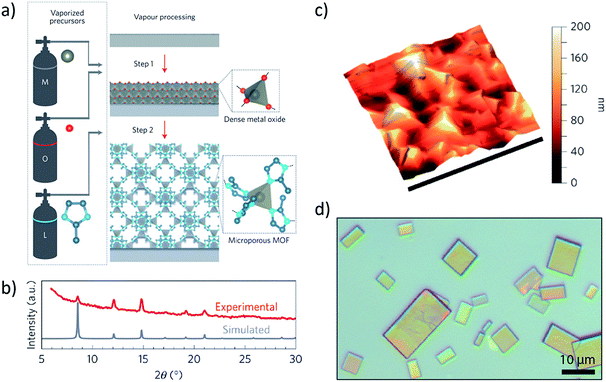 |
| Fig. 6 (a) Schematic representation of CVD synthesis of ZIF-8 through a two-step process involving deposition of a dense metal oxide followed by introduction of organic ligand. (b) Conversion of ZnO to ZIF-8 confirmed via comparison of measured and simulated pXRD patterns. (c) 3D rendered AFM topograph of a polycrystalline film of ZIF-8. (d) Optical images of single crystals of our Mo2(INA)4 MOF synthesized directly through a one-step CVD reaction.112 Adapted by permission from Springer Nature110 Copyright 2016. | |
In our own work, we have utilized a single-step CVD reaction to grow ultra-thin single crystals of a MOF comprised of dimolybdenum paddlewheel clusters.34,112,113 These high quality and phase pure single crystals have exceptionally uniform surfaces, are highly oriented, and can be mechanically exfoliated to yield thin films with thicknesses approaching several nanometers (Fig. 6d). Our work represents the first effort to prepare singly crystalline MOF thin films directly (i.e. not requiring subsequent conversion) from the gas phase. Notably, we discovered unique phase and electronic switching properties in our MOF by monitoring in situ the response of devices we fabricated from single crystals of this MOF.
Challenges remain with regards to the widespread use of vapor phase growth methods for framework materials. Sublimation temperatures of monomers or precursors may be difficult to determine if complete or partial decomposition occurs, and widely different sublimation temperatures of individual components may necessitate multi-step growth processes. Moreover, the initially deposited material may be amorphous and difficult to subsequently crystallize. Finally, coordination of precursors in the gas phase or following their adsorption onto substrates may be difficult in the absence of catalysts. However, we foresee expanded use of gas-phase methods, including CVD, for the preparation and fundamental investigation of high-quality MOF and COF thin films, particularly if novel precursors or frameworks suitable for gas phase growth are developed. We also see an exciting opportunity for facile integration of these diverse materials into devices, including sensors, actuators, and detectors.
3. Characterization
Thorough characterization of 2D or nearly-2D molecular materials is of paramount importance in the quest to discover promising properties that may manifest within these materials, and in the ongoing effort to develop new synthetic strategies to address the exigent challenges discussed above. Several features of a MOF/COF thin film or few-layer nanosheet must be determined in order to inform its subsequent application: (1) film quality, (2) material phase, (3) nanosheet dispersion, (4) stability, and (5) pore size. Most bulk MOF and COF characterization techniques can be translated to the analysis of dimensionally restricted materials without requiring major modification. However, careful attention must be paid to data quality and interpretation of results, because sample variability can introduce systematic errors and because properties measured on bulk materials may be significantly altered when the same material takes on a nanoscale dimension. Though tedious, multiple nanosheets or thin films should be individually characterized to build a robust, statistically-significant dataset. Furthermore, powerful in situ techniques—which may need to be modified or developed specifically for 2D molecular materials studies—will aid in assessing the aforementioned features during synthesis and device fabrication.
Solution-phase characterization is often used for facile and initial assessment of nanoscale materials. Most analyses can be accomplished immediately after synthesis in the reaction vessel or following sample re-suspension in a nonreactive solvent. Tyndall scattering, UV-visible absorbance spectroscopy, and dynamic light scattering (DLS) are bulk techniques used to assess the dispersity of exfoliated MOF and COF samples. Tyndall effect scattering upon laser irradiation of a material dispersion can be readily quantified and, unsurprisingly, has featured prominently in the analysis of many nanosheet and thin film samples (Fig. 7a).23,114,115 Though this technique confirms a suspension of nanoscale particles, the contribution of particle sub-populations cannot easily be discerned. For size distribution analysis, DLS can be used to obtain the average diameters of MOF and COF nanosheet dispersions.116 UV-visible absorbance (UV-Vis) spectra—solution based or solid-state—may be employed to confirm formation of a MOF or COF thin film.72 Liu et al.117 used UV-vis absorbance to monitor the complexation of zinc ions and TCPP by observing a red shift in the ligand's Soret band. A distinct shoulder on the Soret band developed as Zn-TCPP MOF nanodisks formed, with absorption intensity correlating to particle size as a function of modulator inclusion during the crystallization process. Indicating the prevalence of these techniques, Foster et al. recently used all three techniques—Tyndall scattering, DLS, and UV-vis spectral intensity—to assess their post-synthetic exfoliation method for the preparation of ultrathin, catalytic nanosheets.118
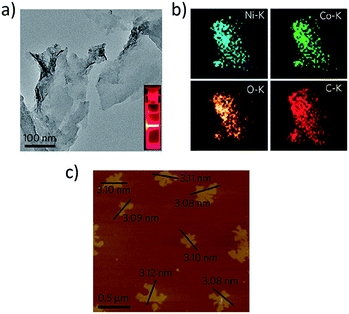 |
| Fig. 7 (a) TEM image of NiCo-UMOFNs with optical image of Tyndall light scattering (inset) upon laser irradiation. (b) EDS elemental mapping of NiCo-UMOFNs obtained within a TEM. (c) AFM map of individual NiCo-UMOFN flakes. Adapted by permission from Springer Nature.115 Copyright 2016. | |
Since single crystal X-ray diffraction is usually impossible due to the inherently restricted dimensionality of nearly-2D molecular films, these materials are frequently characterized with powder X-ray diffraction (pXRD). pXRD allows for relatively straightforward assessment of sample crystallinity, phase, and layering/lattice spacing, but often requires bulk samples, which may not be representative of the individual films, and a known crystal structure to serve as a reference (Fig. 8a and b).75 Though Aida et al.119 were successful in obtaining single crystal structures of their self-exfoliating Cu(II) MOF, pXRD proved useful in studying the accordion-like expansion process their MOF was able to undergo. Upon exposing their MOF to THF vapor, the authors observed an interlayer expansion that was represented by large shifts of the (001) peak in 2θ. This result corroborated previously observed large increases in the interlayer d-spacing from 6.8 Å to 17.0 Å prior to self-exfoliation. Wöll et al.120 employed out-of-plane pXRD to assess the lattice-constants of their liquid-phase epitaxially-grown, heterostructured SURMOFs and to confirm the orientation of their thin film. pXRD can also be used to monitor growth processes and the effects of modulators on film phase, as Ameloot et al. did in monitoring acetic acid vapor modulation on UiO-66 films.121 Synchrotron X-ray diffraction and grazing incidence X-ray diffraction (GIXD) can provide additional information regarding the surface crystallinity and multi-layer composition of a thin film.122,123
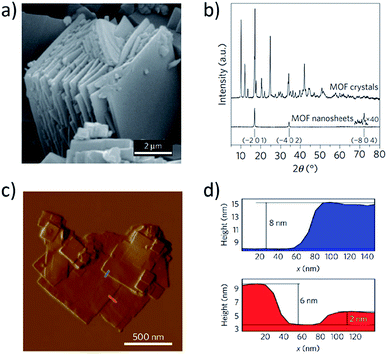 |
| Fig. 8 (a) SEM image of bulk CuBDC MOF crystals comprised of layered nanosheets. (b) pXRD scans of bulk CuBDC MOF crystals and corresponding nanosheets. (c) AFM map of MOF nanosheets. (d) Height profiles taken along the paths indicated in (c). Adapted by permission from Springer Nature.75 Copyright 2015. | |
Though useful, diffraction techniques are not always well suited to provide information regarding film composition. Given its inherent surface sensitivity, X-ray photoelectron spectroscopy (XPS) is often used to identify both the elemental composition and the metal-linker coordination on the surface of molecular nanosheets.39,124,125 Other characterization methods that provide chemical information include Fourier-transform infrared (FT-IR), Raman,72,117,126 and solid state nuclear magnetic resonance (NMR) spectroscopies.127,128 Also commonly used in the MOF and COF community, Brunauer–Emmett–Teller (BET) analysis probes the pore structure of thin films and nanosheets by fitting their gas adsorption–desorption95,104,129,130 isotherms. Calculated surface areas from BET analysis indicate the accessible pore volume of MOF and COF materials and are used to compare these materials on the basis of their gas storage potential or permeability.
In contrast to these aforementioned techniques, which are most often performed on bulk quantities of sample, optical microscopy and atomic force microscopy (AFM) are most informative when deployed on individual specimens or distinct and smaller regions of a sample. Optical microscopies, especially those encompassing dark-field and phase-contrast imaging, are useful for visualizing film quality and surface defects in larger crystals or large-area thin films, though probe depth and resolution are defined by the energy and intensity of the optical beam, parameters of the microscope optics, and the Abbe diffraction limit. Zhao et al. could clearly observe the surface roughness of their millimeter-wide Cu-TCPP thin films by visualizing regions having different textures and color saturations.131 Atomic force microscopy (AFM) is considerably more useful for analyzing the surface roughness and thickness of nanoscale thin films. Most analyses of exfoliated thin film MOFs and COFs utilize AFM to measure the step-heights of individual layers within a multi-layer film (Fig. 7c, 8c and d).57,75,115,132,133 The angstrom-level z (height) resolution of AFM facilitates comparison of AFM acquired step-heights to known crystal lattice spacings, provided that the 2D layers are properly oriented.
Electron microscopy, which encompasses a range of advanced techniques including scanning electron microscopy (SEM), transmission electron microscopy (TEM), scanning transmission electron microscopy (STEM), and, most recently, in situ and aberration-corrected TEM/STEM, is used to obtain high-resolution to atomic-resolution data of ultrathin molecular materials. The insulating nature of many MOFs and COFs presents imaging challenges, though many of these can be overcome through the use of conductive support films and coatings, which provide effective charge dissipation. The complementary resolution levels of SEM and TEM images provide excellent structural insight across multiple lengthscales. SEM often lends morphological insight regarding the likelihood that a sample can be readily exfoliated114 and TEM provides an assessment of the crystallinity and inter-layer alignment within multi-layer films.128 When combined with energy-dispersive X-ray spectroscopy (EDS), SEM or TEM can also provide elemental mapping of the sample's surface (Fig. 7b).39,115,134,135 Yang et al.23 clearly show the flake-like, layered morphology of their bulk Zn2(bim)4 single crystals and provide TEM images of the impressively thin nanosheets obtained after wet-ball milling and ultrasonication (Fig. 1a). Thomas et al.98 similarly show SEM and TEM images of their MOF nanosheets prepared through microdroplet flow reaction. High resolution TEM (HR-TEM) images of their MF-ZrBTB nanosheets show clear lattice fringes with Zr6 clusters consistently spaced apart by the expected lattice distances, thereby suggesting good crystallinity of their nanosheets. Selected-area electron diffraction (SAED) can be performed during TEM data collection to assess the local crystallinity of sufficiently thin samples. Diffractograms obtained through SAED can be compared to known crystal structures in order to index crystalline grains or local phases in MOF or COF samples.116 Advanced dark-field imaging techniques, notably high-angle annular dark-field and precession diffraction-based imaging, can provide exceptionally detailed information as to how the phase, orientation, and defect density vary across a thin sample. Care must be taken to employ imaging conditions (e.g. acceleration voltages and beam currents) that do not degrade the MOF or COF samples, with low-kV imaging being of particular utility in this regard. Though less frequently used, scanning tunneling microscopy (STM) is a powerful technique that allows for the imaging of single MOF or COF layers on metal substrates and provides atom-level resolution and electronic structure information at the monolayer level.136–139
The choice of characterization technique can also be guided by the intended application of the 2D nanosheet. For some systems, magnetic susceptibility measurements are useful for investigating electronic structure and bonding. Gómez-García and coworkers identified ferromagnetic Fe–Fe interactions in their MOF systems likely arising from mixed-valency of the Fe(II)/Fe(III) lattice.56 For these and other functional MOF and COF systems, authors are increasingly providing charge transport measurements.95,140–142 We recently assessed the conductivity of our dimolybdenum isonicotinate crystalline thin film devices. Current–voltage characteristics obtained from these devices identify a conductivity of 2.25 × 10−8 S cm−1, which increases by 8-fold under laser illumination. Notably, we observe a large increase in conductivity to 6.22 × 10−7 S cm−1 while monitoring conversion of the crystal in situ.112
Indeed, the fact that crystalline thin films and nanosheets can change their phases and associated properties mandates the development and use of in situ characterization methods to monitor and potentially control solid-state transitions.143 More importantly, in situ analysis of MOF and COF thin film growth would provide valuable insights regarding plausible mechanisms for formation of these coordinated frameworks. In turn, such studies could inform design and optimization of reactions to achieve higher quality 2D molecular materials. An assortment of in situ X-ray diffraction techniques is currently available but their widespread use is limited.144 For example, in situ high energy, X-ray atomic pair distribution function analysis can monitor nucleation by providing structural information as precursors mix but requires a synchrotron source.145 When paired with in situ TOF-SIMS spectroscopy, however, the assembly and modification of molecular species in solution can be monitored.145 Methods to monitor morphology and crystallinity, such as in situ SEM146 and TEM,147 exist and are employed for 2D atomic materials but have not been readily used for analysis of molecular systems. Weckhuysen et al. developed an in situ AFM method and used it to monitor HKUST-1 thin film growth by observing a 10 × 10 μm section of Cu-BTC solution deposited on a functionalized Au substrate.148 Nucleation of copper and BTC linker was observed and HKUST-1 grain formation could be monitored at several temperatures. In an effort to provide chemical information, Parsons et al. developed an in situ technique for attenuated total reflectance infrared spectroscopy.149 The formation of HKUST-1 was monitored by examining the conversion of ZnO and Cu(NO3)2 to the MOF upon addition of H3BTC. The symmetric and asymmetric stretching modes of the HKUST-1 carboxylate groups were monitored every 0.49 seconds. Continued development of in situ characterization techniques for 2D molecular materials will undoubtedly advance further investigation of these systems.
4. Applications
While researchers continue to explore the potential of true 2D MOFs and COFs, these materials have demonstrated impact in a number of electronics, energy storage, catalysis, and biomedicine applications. The plethora of fundamental studies and applications enabled by 2D atomic crystals bodes well for the potential impact of 2D molecular frameworks, which allow for an exceptional degree of freedom in chemical design. We anticipate that collaboration between the solid-state, inorganic, and condensed matter communities will spawn new research areas with extraordinary potential. Here, we highlight some of the current, innovative applications using 2D or nearly-2D molecular materials, as well as some hybrid materials. Notably, not all cited work focuses exclusively on nanoscale or monolayer molecular materials. Research focused on thicker, microscale thin films is valuable and welcome as findings from these systems provide important insights towards the development and scale-up of more dimensionally restricted molecular materials.
4.1 Catalysis
2D MOF and COF materials are candidate heterogenous catalysts for the hydrogen and oxygen evolution reactions (HER and OER) and for small molecule activation. Marinescu et al.150 deposited thin films of their cobalt dithiolene catalysts onto assorted substrates and reported an overpotential of 530 mV at 10 mA cm−2 for this thin film catalyst that is lower than that for the bulk molecular material. In related work, Feng and co-workers151 prepared a single-layer sheet of their nickel bis(dithiolene) complex and measured an overpotential of 333 mV at 10 mA cm−2. More recently, Marinescu et al.152 reported a record hydrogen evolution activity from one of a series of benzenehexathiolate (BHT) coordination framework films. Compared against NiBHT and FeBHT, CoBHT films generally produced the lowest overpotential of 185 mV with film thicknesses of ∼244 nm. Stacked sheets of CoBHT were observed at thicknesses as low as 20 nm and as high as nearly 1 μm, but these thicknesses were found to produce larger overpotentials.
As part of a further development of electrocatalysts to mediate HER, Nishihara et al.153 prepared bis(iminothiolato)nickel (NiIT) nanosheets, where an increase in conductivity from 3 × 10−6 to 1 × 10−3 S cm−1 was ascribed to preparation of the authors' NiIT material as a nanosheet. The NiIT nanosheets were then employed as HER electrocatalysts and reported to have an onset overpotential of −0.15 V vs. RHE and an operating overpotential of −0.37 V at 10 mA cm−2. Qiao and coworkers154 prepared a catalytically-active hybrid Co-BDC/MoS2 material by exposing exfoliated MoS2 nanosheets to CoCl2·6H2O and BDC solutions. These hybrid nanosheets demonstrate heightened HER activity in alkaline electrolytes with an onset overpotential of 155 mV. This overpotential is lower than would be the case with either component in isolation. To achieve current densities of 10 mA cm−2, the Co-BDC/MoS2 nanosheets required only 248 mV of overpotential. Moreover, even after the acquisition of 2000 cyclic voltammograms, the nanosheets exhibited good stability.
Tang et al.115 prepared ultrathin mixed-metal NiCo MOF nanosheets (approximately three structural layers) and tested their efficacy as catalysts for OER. When the NiCo nanosheets were deposited on glassy-carbon and on copper foam, the overpotentials required to achieve a 10 mA cm−2 current density were measured at 250 mV and 189 mV, respectively. The NiCo MOF nanosheets also exhibit a significantly higher turnover frequency compared to either monometallic (Ni or Co containing) nanosheets or the bulk mixed-metal phase. Zhang et al. produced 2 nm thick nanosheets of their pillared cobalt framework, as previously described,60 and reported low overpotentials (211 mV at 10 mA cm−2) and high turnover frequencies. Similarly, Zhao et al.155 measured a 240 mV overpotential at 10 mA cm−2 for OER with their NiFe MOF nanosheets. When assessing the activity of this material for HER, they observed a 134 mV overpotential. The NiFe-MOF nanosheet was then used as both the anode and the cathode in experiments assessing its efficacy in electrocatalytic water splitting. At an operating voltage of 1.60 V, H2 and O2 bubbles could be seen at the cathode and anode, respectively. Recently, Lang et al.156 also produced nanosheets of mixed metal MOFs for OER. NiFe MOF nanosheets, solvothermally synthesized from Ni(OAc)2·4H2O, Fe3SO4·7H2O, and 1,4-H2BDC, were measured to be 1.67 nm to 2.58 nm thick. The nanosheets produced an overpotential of 221 mV at a current density of 10 mA cm−2, and were judged to perform better than monometallic MOF and commercial Ir/C. While other mixed metal nanosheets were prepared (e.g. comprising Al, Co, Mn, Zn, and Cd), the Fe-containing MOF exhibited the most impressive OER activity and stability, with only minimal changes in activity over 1000 CV cycles.
Dincă et al.157 prepared conductive thin films of Ni3(HITP)2 on electrode surfaces and assessed their efficacy as catalysts for the OER. Films ranging from 120 nm to 300 nm thick, supported on either glassy carbon electrodes or indium tin oxide, produced an overpotential of 0.18 V (relative to Pt) and reduced oxygen with an onset potential of 0.82 V in 0.1 M KOH. Analysis of the thin films before and after reaction revealed marked stability of the catalyst. Yang, Yaghi, and coworkers158 prepared MOF thin films for electrochemical reduction of CO2. The catalytic current density increased upon adjusting the thickness of the cobalt–porphyrin MOF films from 10 nm to 70 nm, and this effect was ascribed to increased cobalt loading within the sample. By monitoring CO and H2 production, current selectivity for CO formation reached 76% at 0.7 V vs. RHE (Fig. 9). Farha and Hupp et al.159 also reported robust MOF thin films for CO2 reduction using the MOF-525 iron–porphyrin framework.
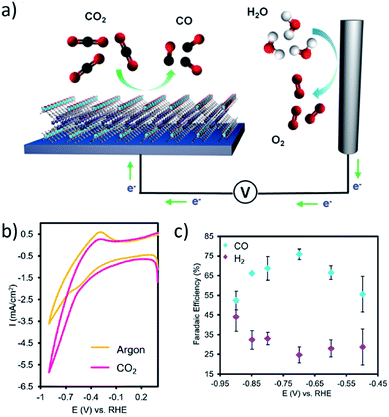 |
| Fig. 9 (a) Schematic showing a thin film cobalt–porphyrin MOF, Al2(OH)2TCPP-Co, performing CO2 reduction. (b) An increase in current is observed in the cyclic voltammogram when the MOF is subjected to a CO2 environment. (c) Selectivity for generation of CO and H2 was tested over the potential range −0.5 to −0.9 V vs. RHE with a reported peak faradaic efficiency for CO of 76%. Adapted with permission.158 Copyright 2015 American Chemical Society. | |
Hybrid catalytic materials are also of great interest. Zhao et al.131 prepared Cu-MOF nanosheet composites with Au nanoparticles decorating the MOF surface. The catalytic behavior of the nanocomposite was investigated by monitoring reduction of 4-nitrophenol to 4-aminophenol by UV-vis absorption spectroscopy. The authors proposed that the layered MOF nanosheet architecture facilitates π–π stacking interactions between the MOF linker molecules and aromatic phenols. These interactions in turn improve electron transfer between the supported Au nanocatalysts and the phenol species. Zhang et al.160 also prepared composites comprised of Au nanoparticles and MOF nanosheets. Their Au NP/CuTCPP(Co) hybrid material oxidized 3,3′,5,5′-tetramethylbenzidine in the presence of hydrogen peroxide and was considered to behave as a peroxidase biomimetic catalyst. Showing the potential benefit of mixing discrete MOF phases for catalytic effect, Dou et al.161 incorporated Fe-MOF nanoparticles within Ni-MOF nanosheets and lowered the overpotential for OER to 256 mV, down from 370 mV for the neat Ni-MOF.
4.2 Charge transport and storage
The prospect of eliciting efficient and tunable charge transport and storage in 2D MOFs and COFs has driven extensive research over the past decade.16,17,45,162–165 Multiple groups have developed graphene-like MOFs and COFs in hopes of achieving record values for conductivity or capacitance.166–169 Dincă et al. produced one of the most highly conductive MOFs, Ni3(HITP)2, and subsequently prepared films with 500 nm thicknesses. Films deposited on quartz substrates resulted in room temperature conductivities of 40 S cm−1 and also exhibited higher conductivities with increasing temperature (Fig. 10).168 Cánovas et al.170 identified a 2D MOF, Fe3(THT)2(NH4)3, with a room temperature carrier mobility of 220 cm2 V−1 s−1 and a direct bandgap of 0.25 eV. These semiconducting films were prepared by an interfacial growth method that yielded large area, multilayer samples.
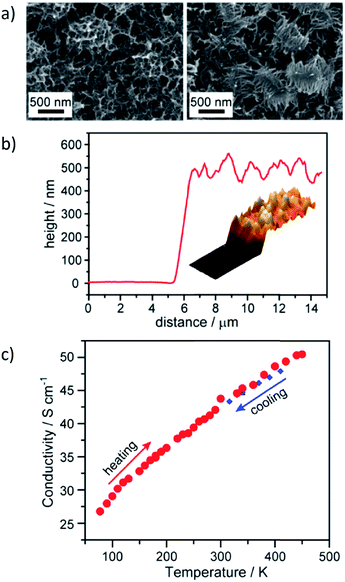 |
| Fig. 10 a) SEM micrographs of Ni3(HITP)2 films with 500 nm thickness measured via (b) AFM. (c) van der Pauw conductivity measurements at various temperatures show reversible increase in conductivity for 500 nm thick films on quartz. Adapted with permission.168 Copyright 2014 American Chemical Society. | |
Gómez-García and coworkers56 further characterized both of their mixed-valence, magnetic Fe(II)/Fe(III) MOFs and reported room temperature conductivities of 0.03 and 0.003 S cm−1 for bulk crystals when measured parallel to the honeycomb MOF structure. Their measurements revealed much lower conductivities (∼10−4 and 10−6 S cm−1) when measuring perpendicular to the honeycomb layer. Though nanosheets of these MOFs were produced, their associated carrier conductivities were not reported. Conversely, Nishihara et al.142 successfully prepared nanosheets of their nickel bis(dithiolene) complex and reported a conductivity of 160 S cm−1 for a single crystalline flake using a four-point probe setup within an SEM. Also using four-point probe measurements, Zhu et al.70 recorded a high conductivity of 1580 S cm−1 for their copper benzenehexathiol (Cu-BHT) coordination polymer. FETs were subsequently prepared from the Cu-BHT films and exhibited high hole- and electron-mobilities, with a single FET device managing to exhibit a hole mobility of 99 cm2 V−1 s−1 and electron mobility of 116 cm2 V−1 s−1. Xu et al.171 also fabricated a FET incorporating a Ni3(HITP)2 membrane prepared via an air–liquid interfacial method. Multiple devices indicated hole mobilities ranging from 38 ± 8 cm2 V−1 s−1 to 48.6 cm2 V−1 s−1. The authors hypothesized that these high values result from extensive charge delocalization within the layers, which are comprised of nickel ions coordinated to ligands with radical character. Close-packing of each vertical layer may also introduce opportunistic π–π interactions.
Pursuing the goal of targeted molecular design of graphene-like MOFs and COFs, Dichtel et al. prepared oriented thin films of a COF comprised of a redox-active monomer, 2,6-diaminoanthraquinone (DAAQ), and 1,3,5-triformylphluroglucinol (TFP).172 Slow injection of TFP into a DAAQ solution yields 60–560 nm thick oriented thin films with areal capacitance values from 1.2 to 3.0 mF cm−2 that are higher than those measured on randomly oriented slurries, thereby suggesting the supercapacitor potential of the former. Over 5000 galvanostatic charge–discharge cycles (GCDC), the films show only 7% capacitance loss. Focusing on their Ni3(HITP)2 material, Dincă et al. prepared the first solely MOF comprised electrochemical double layer capacitors (EDLCs) and showed that their devices reach a normalized gravimetric capacitance of 18 μF cm−2, exceeding the values observed in most carbon materials.173 Additional studies have also utilized exfoliated COF nanosheets and films as ion conductors and charge storage materials.63,64,174–176 Chen et al. prepared a cationic COF skeleton nanosheet to sequester lithium salt anions and increase the concentration of free Li+, and in so doing increased lithium conductivity within the material.174 Furthermore, Fan and coworkers recently showed an ∼3-fold increase in the specific capacitance of CTF-1 triazine COF nanosheets, relative to the bulk COF, and also noted improved capacity retention during cycling of lithium batteries wherein CTF-1 was used as the anode material.64 These results collectively encourage continued efforts to demonstrate the unique potential of MOFs and COFs as active components in charge carrying and storing devices.
Surface-mounted MOF (SURMOF) or COF thin films and 2D sheets can serve as prototypical examples of organized molecular systems for device applications. Ballav et al. prepared a Cu-TCNQ SURMOF on mercaptoundecoanoic acid-functionalized FTO and Au substrates using the layer-by-layer (LbL) method.177 Electron beam lithography facilitated the fabrication of Cu-TCNQ SURMOF thin film devices with consistent conductivity values in the range of 10−5 S cm−1. The SURMOF of Cu-TCNQ also exhibited unique rectifying behavior when exposed to I2 vapor, while bulk Cu-TCNQ under identical exposure conditions did not. Though not explicitly a SURMOF, Allendorf et al.88 prepared a Cu3(BTC)2 thin film on patterned SiO2/Si wafers and soaked the film in a TCNQ/dichloromethane solution to load the MOF with conductive TCNQ. The conductivity of the loaded film was measured to be as high as 7 S m−1, thereby suggesting that loading 2D molecular materials with functional compounds may likewise be a viable strategy for introducing desirable properties.
4.3 Sensing
Two-dimensional MOFs and COFs are attractive candidate sensor materials due to their highly exposed pore and surface areas and the inherent sensitivity of their electronic structures to gating through adsorption or binding of analytes. MOF and COF thin film or nearly-2D materials have the potential to detect gases, solvents, metal ions178,179 and contaminants20,180 if interaction of these analytes with the framework induces, ideally reversible, optical, electronic, or crystallographic changes. In this regard, the Mirica group has recently demonstrated the broad utility of MOFs as sensors by incorporating assorted 2D frameworks into detection platforms. Their self-organized frameworks on textile (SOFT) devices (Fig. 11),181 MOF-loaded shrinkable polymer films,182 and bimetallic 2D MOF device prepared through dropcast183 are good sensors for NO, H2S, NH3, and/or H2O.
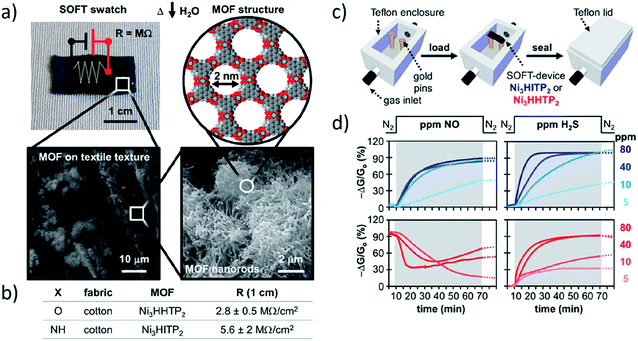 |
| Fig. 11 (a) Conductive SOFT-devices produced from solvothermal condensation of MOF reagents in water onto textiles. Photograph of SOFT-device (top left), SEM images of textile fibers coated by MOF film (bottom left), SEM image of characteristic MOF nanorod structure (bottom right), and space-filling model of MOF (top right). (b) Sheet resistance measurements for SOFT-devices. (c) Schematic of custom enclosure for measuring (d) response of SOFT-sensors when exposed to NO (left) or H2S (right). Adapted with permission.181 Copyright 2017 American Chemical Society. | |
Focusing on smaller scale materials, Allendorf et al. prepared HKUST-1 thin films on substrates by spin coating a DMSO solution containing Cu(NO3)2·3H2O and 1,3,5-benzenetricarboxylic acid in a layer-by-layer manner.184 Between each layering cycle, the deposited HKUST-1 layer was heated for five minutes at 70 °C. As a final step, the MOF was soaked in methanol and activated at 100 °C for 30 min to ready the thin film for sensing tests (Fig. 12a). The purple films were exposed to 0.2–95% relative humidity (RH) at 296 K and reflectance spectroscopy was used to monitor the thin film response. Exposure to humidity, particularly at lower RH, red shifts the reflectance spectrum and correlates to a color change from purple to light blue in the HKUST-1 thin film. Removal of water through a 5 min dry air purge results in reversion of the MOF thin film to its previous state. In this manner, trends in the percent reflectance of the thin film as a function of RH were assessed (Fig. 12b and c). The thin film was even incorporated into a practical LED photodector for low RH testing. Eddaoudi et al. prepared two thin films, NbOFFIVE-1-Ni and ALFFIVE-1-Ni, that enabled detection of CO2 down to 400–5000 ppm.185 In this work, a novel paste-like MOF deposition method was used to prepare homogenous and uniform thin film coatings of both MOFs on interdigitated electrodes (IDE) and QCMs. The IDE sensing assessment showed a decrease in capacitance of the MOFs during their exposure to various CO2 concentrations, while QCM data reflected in situ changes in the MOFs' mass due to surface adsorption of CO2.
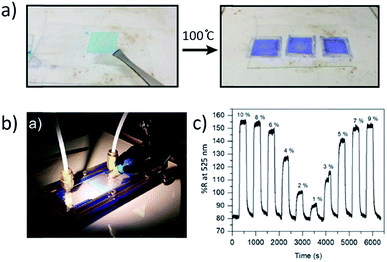 |
| Fig. 12 (a) Color change from blue to purple upon activation of the HKUST-1 thin films, which were deposited through layer-by-layer methods. (b) Photograph of the authors' gas flow cell and reflectance measurement setup. (c) Plot of percent reflectance vs. time of HKUST-1 thin films upon their exposure to 0–10% RH for 5 min, followed by 5 min intervals of dry air purge. Adapted with permission.184 Copyright 2018 American Chemical Society. | |
Many MOF or COF thin films have been prepared to detect small organic molecules. Dincă et al. dropcasted Cu3(HITP)2
186 dispersions to prepare an ammonia vapor sensor with sub-ppm detection capabilities. Xu et al.141 demonstrated a 2–20 nm Cu3(HHTP)2 thin film grown by spray LbL as an excellent room-temperature, chemiresistive ammonia sensor. The thin film selectively detects ammonia and can reliably report on concentrations as low as 100 ppm. Dincă et al. also identified that pellets of their conductive 2D MOFs can be used as sensors for assorted volatile organic compounds,13 suggesting that singly crystalline thin films or nanosheets could be promising sensors. Ethylamine sensing was accomplished by Zn(BDC)(H2O) MOF nanosheets prepared by Jiang et al.187 Here, binding of ethylamine by the MOF was monitored through fluorescence spectroscopy. Banerjee and coworkers36 identified COF nanosheets which exhibit a concentration-dependent fluorescence response in the presence of assorted nitroaromatic compounds such as 2,4,6-trinitrophenol (TNP). Xian et al. also reported on the use of covalent organic nanosheets as fluorescent sensors for TNP detection.188 Sonication of their bulk polyimide COF produced nanosheets as thin as 1 nm, which amounts to only a few molecular layers. In the presence of increasing concentrations of TNP, the fluorescence intensity of the COF nanosheets decreased in a linear fashion. The authors suggest that this observed fluorescence quenching arises from ground state electron transfer from TNP− to the COF framework. Liu et al.117 prepared few-layer Zn-TCPP(BP) crystals by incorporating a 4,4′-biphenyldicarboxylic acid (BP) nucleation modulator during the growth process. The resulting zinc nanodisks catalytically bind then oxidize nitrite ions, exhibiting a low nitrite detection limit of 0.26 μM, owing to the large contact area and layered structure of crystalline Zn-TCPP(BP).
Biologically-inspired MOF sensors are also the focus of considerable research into applications of MOF thin films and nanosheets. Electrochemical glucose detection was reported by Lu and Wu125 with their nickel-based MOF nanosheet on nickel foam. The Ni-MOF/nickel foam electrode exhibited a unique oxidation peak in CV measurements compared to a bare nickel foam electrode. The magnitude of this oxidation peak was enhanced upon addition of a 1.0 mM glucose solution, possibly due to catalytic oxidation of glucose to gluconolactone by the Ni-MOF. Ultimately, the sensor exhibited a low 85 nM detection limit for glucose and a sensitivity of 14
858 μA mM−1 cm−2. Ultrathin imine-linked COF nanosheets, TPA-COF, prepared by Zhang and coworkers were used for DNA detection.189 Solutions of TPA-COF nanosheets were added to mixtures of buffer containing hairpin DNA probes, H1 (fluorescent dye-labeled) and H2. H1 individually interacts with TPA-COF nanosheets and is quenched due to π–π interactions. In the presence of target DNA, a hybridization chain reaction occurs and forms double-stranded DNA (dsDNA). In this case, H1 no longer interacts with TPA-COF nanosheets and fluorescence is recovered. The authors noted that the magnitude of fluorescence quenching is enhanced when nanosheets of TPA-COF are used instead of the bulk COF. Zhang et al. reported similar DNA sensing experiments for their surfactant-formed MOF nanosheets.77 Other nanoscale MOF sensors have been used to detect intracellular pH190 and O2
191 or have served as electrochemical H2O2 sensors.192
4.4 Separations
The pore size and functionality of 2D MOF and COF materials193 can be designed to host specific chemical interactions and to impose specific constraints ideally suited for selective gas194,195 and liquid separation,196–199 enantiomer separation,200–202 and even targeted drug delivery.27 Liquid separations are commonly modeled with dye molecules and have been pursued with MOF and COF membranes. Ang and Hong prepared porphyrin-based Zn-TCP(Fe) nanosheets with carboxylate pendant groups at the nanosheet edges, which cross-link with polycationic polymer molecules.203 The nanosheets were then transferred to nylon supports. The 48 nm membranes exhibit minimal defects and high water permeance. Impressive rejection rates for four organic dyes, methyl red (MR), methylene blue (MnB), methyl orange (MO), and brilliant blue G (BB), and four salts, MgCl2, Na2SO4, NaCl, and MgSO4 were determined. Banerjee et al. prepared self-standing covalent organic membranes204 comprised of platelet-like COF sheets. Though the membranes themselves are thick, 200–700 μm, separation of rose Bengal (RB), methylene blue (MB) and Congo red (CR) aqueous dye solutions was successful, with demonstrated rejection rates of 99%, 96% and 94%, respectively. Active pharmaceutical ingredients (APIs) such as vitamin B12 and tetracycline were rejected at rates as high as 99% and 81%. Such membranes may serve as an affordable and robust solution to current wastewater treatment concerns. Additional work by Banerjee and coworkers205 prepared large area, transparent COF thin films with thicknesses of 50–90 nm from liquid–liquid interfacial synthesis. Rejection rates for an additional set of organic dyes were calculated between 79–99% for these films. Furthermore, the films appeared stable during at least 24 hour periods of continuous aqueous solution exposure. In an alternative application of liquid separations, MOFs and COFs have been targeted as potential materials for water desalination. Grossman et al. previously showed nanoporous graphene was effective at filtering NaCl salt from water206 and expanded their efforts to COFs.207 In a computational investigation, ultrathin films of a 2D triazine COF were assessed and found to reject salt at a 91% rate while allowing water to pass through at a rate of 70 L per cm2 per day per MPa. Calculations have also shown that COF nanosheets can in principle reject 100% of MgCl2 with high waterpermeance.208 Other computational efforts have considered the incorporation of UiO-66 MOFs in membranes for desalination,209 with a recent study by Zhao et al. showing Na+ rejection as high as 74.9% with fair aqueous stability.210 Continued improvement of COF and MOF aqueous stabilities is required before their potential in water desalination is fully realized.
With regards to gas separations, Zhao et al.211 prepared ∼4 nm thick nanosheets from their nickel based MAMS-1 MOF by gentle freeze-thaw exfoliation, which was used to avoid extensive crystal damage. Subsequent hot-drop casting deposited aligned nanosheets onto anodic aluminum oxide substrates. The resulting membranes (which ranged 4–40 nm in thickness) exhibited thermo-switchable H2 permeation and impressive H2/CO2 separation for 2D membranes. Dam and coworkers212 prepared a MOF thin film on the surface of a Pd thin film to serve as a protective, pre-sieving layer for CO/H2 separation. Long et al.213 prepared a mixed-matrix membrane loaded with Mg2(dobdc) MOF nanocrystals. Polymer films loaded with MOF nanocrystals exhibited increased gas permeability for H2, N2, CH4, and CO2 and improved separation of H2/CH4 and H2/N2 mixtures. COFs have also been integrated into mixed-matrix membranes for gas separations applications. Zhao et al. exfoliated bulk, water-stable COFs into monolayers or nanosheets which were subsequently mixed with poly(ether imide) or polybenzimidazole. Their NUS-2@PBI membrane, loaded with 20% COF, showed a 31.4H2/CO2 selectivity.214 Other mixed-matrix membranes prepared with COF-5 nanosheets and Pebax-1657 show high CO2 uptake capacities and 49.3 CO2/N2 selectivity compared to the neat polymer membrane. These results were accomplished with a COF loading as low as 0.4 wt%.215
4.5 Gas uptake
The same features that enable MOF and COF thin films to participate in selective molecular separations render them promising candidates as gas storage materials. Carbon dioxide storage has attracted much attention from the MOF and COF community but remains challenging for MOF and COF nanosheets, which lack bulk porosity for adsorption. Moorthy et al.216 delaminated their bulk imidazole and triptycene-based MOFs into nanosheets by disrupting interlayer hydrogen bonding. CO2 adsorption capacities as high as 124–208 cm3 g−1 were measured for the bulk MOFs and are better than reported uptake values for N2 and H2, but adsorption capacities are not reported for the nanosheets. Do et al.217 formed nanocube, nanosheet, and nanorod morphologies of their Cu2(ndc)2(dabco) MOF by incorporating acetic acid and/or pyridine modulators during synthesis. CO2 uptake for each morphology was improved relative to the bulk, and measured 5.0, 4.8, 4.3, and 3.5 mmol g−1 (at 1 bar and 273 K) for the nanocube, nanosheet, nanorod, and bulk samples, respectively. By modulating crystal growth and repressing certain crystal faces, tailored materials with specific morphologies and facial functionalities can be prepared. Kang and coworkers218 compared CO2 working capacities of ZIF-8, ZIF-L, and dia(Zn)-HCOONa (a hexagonal nanosheet). At elevated temperatures between 50–100 °C, similar working capacities were observed whereas the latter two systems were considered nonporous at 77 K in N2 physisorption experiments. The authors suggest increased framework flexibility at higher temperatures likely correlates to increased CO2 adsorption. Coskun et al. synthesized graphene/ZIF-8 composites219 and investigated their CO2 capture at 303 K and at pressures as high as 35 bar. Rao et al.220 also prepared graphene/MOF composites with their Cd-PBM MOF. The composites, totaling 2.5–3 nm in thickness, appear to undergo uptake of CO2 in a stepwise manner with significant hysteresis. In efforts to improve MOF gas adsorption on the nanoscale, Zhang and coworkers demonstrated higher CO2 uptake by ZIF-L nanosheets supported on halloysite nanotube (HNT) membranes compared to samples without HNT.221 Careful selection of substrates and solid supports are therefore crucial in continued understanding of nanosheet properties.
4.6 Protective coatings
The chemical resilience of many MOF and COF thin films and 2D molecular materials has motivated their use as protective coatings for assorted functional materials.95,222 Multiple biological applications of MOF coatings have been pursued. Falcaro et al.223 crystallized a ZIF-8 film on the surface of a β-galactosidase-coated eukaryotic yeast cell. The ∼100 nm MOF film protected the cell from toxic compounds and still allowed for diffusion of important bioactive molecules such as lactose. Interestingly, the cells were able to resume normal growth upon removal of the MOF. Wöll et al.224 demonstrated two potential SURMOF thin film coatings that were highly stable in water and artificial seawater and were harmless to surrounding fibroblasts, which were used to model a cellular environment. Tsotsalas et al.225 utilized a SURMOF film coating as an intermediate on pathway to a porphyrin polymer thin film. The film demonstrated antibacterial activity toward E. coli in the presence of light, indicating that 1O2 and free radicals likely contributed to bacterial death. Wöll and coworkers also demonstrated the ability to convert SURMOFs to gel coatings.225 In a demonstration of the potential for MOFs to serve as defensive coatings against chemical warfare agents, Farha et al.226 showed that thin films of assorted zirconium metal–organic frameworks were able to photocatalytically oxidize 2-chloroethyl ethyl sulfide (CEES), a mustard gas simulant. Thin films of MOFs UMCM-313 and NU-1000 were prepared on FTO substrates using a LbL technique. Irradiation of the thin films by LEDs produced singlet oxygen, which was responsible for oxidizing the mustard gas simulant to nontoxic products with CEES half-lives of 145 min and 76 min (Fig. 13). Ultimately, the thin films showed a higher TOF than the bulk MOFs. Other studies have shown MOF thin films can also be used as functional coatings for microelectrodes in electrocatalytic reactions such as CO2 reduction.227
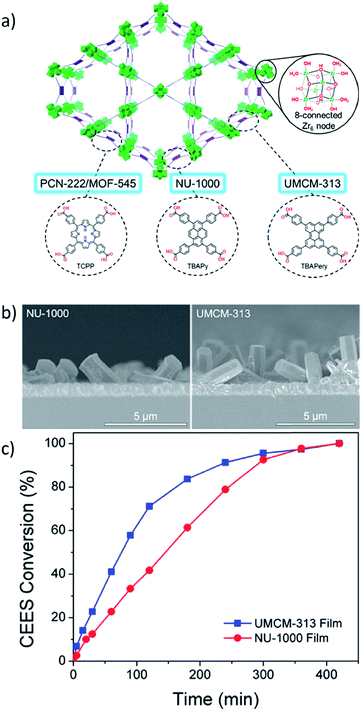 |
| Fig. 13 (a) General structure of three MOFs, PCN-222/MOF-545, NU-1000, and UMCM-313, used as a thin-film protective coating against mustard gas simulant. (b) NU-1000 and UMCM-313 thin films prepared using layer-by-layer techniques on FTO substrates and (c) their reaction profiles for the photocatalytic oxidation of CEES. Adapted with permission.226 Copyright 2018 American Chemical Society. | |
5. Prospects for future research
The future is bright for 2D molecular materials especially if breakthroughs in the synthesis, characterization, and utilization of these materials can mirror the great research strides made in 2D atomic materials over the past decade. 2D molecular frameworks allow for an exceptional degree of freedom in chemical design, a feature that bodes well for uncovering a plethora of new properties and applications. By drawing upon synthetic and analytical expertise from the chemical (e.g. inorganic and physical), physical (e.g. condensed matter), and engineering communities, 2D molecular materials research has the potential to spawn new interdisciplinary research collaborations. Ultimately, 2D molecular lattices, be they 2D MOFs or COFs, are a compelling platform through which to discover new and emergent properties and to expand the library of 2D materials.
Several areas of research should be prioritized to fulfill the myriad opportunities presented by 2D molecular lattices. First, synthetic breakthroughs are needed to consistently prepare high-quality MOF and COF thin films and true 2D lattices. Explicit control of dimensionality and phase is vital, as is attention to preparing these defect-free materials at scale for integration into technology platforms. In this regard, gas-phase methods show promise and need further investigation. Second, characterization techniques capable of high-throughput and in situ analysis are desirable. The often tedious, but necessary, process of characterizing in detail discrete 2D nanosheets or discrete regions of larger samples would benefit from automated methods or faster screening methods. Likewise, continued development of in situ characterization techniques for 2D molecular materials is crucial to advance investigation of these systems. Scanning tunneling microscopy/spectroscopy (STM/S) could emerge as a powerful technique for visualizing the structural and electronic evolution of single MOF or COF layers as they are assembled on surfaces. Finally, rigorous electronic transport measurements performed on devices fabricated from crystalline 2D molecular lattices and thin films are a vital tool for uncovering new physics and new opportunities to create optoelectronic devices. The aforementioned efforts must be pursued alongside existing studies exploring the potential of true 2D MOFs and COFs for electronics, energy storage, catalysis, and biomedicine.
Abbreviations
Zn2(bim)4 | Poly[Zn2(benzimidazole)4] |
PPF-1 | Zn2(tetrakis(4-carboxyphenyl)porphyrin)4 |
Cu2(bdc)2, Cu(bdc) | Cu2(1,4-benzenedicarboxylate)2 |
Cu(BHT) | Cu3(benzenehexathiol)n |
Au3(BHT)2 | Au3(benzenehexathiol)2 |
Ag3(BHT)2 | Ag3(benzenehexathiol)2 |
Zn-TCPP (i.e., PPF-1) | Zn2(tetrakis(4-carboxyphenyl)porphyrin)4 |
Zn(Bim)OAc | Zn(benzimidazole)(acetate) |
Cu-TCPP | Cu2(tetrakis(4-carboxyphenyl)porphyrin)4 |
CoTCPP | 5,10,15,20-Tetrakis(4-carboxyphenyl)porphyrinato-cobalt(II) |
ZIF-8 | Zn(2-methylimidazolate)2 |
HKUST-1 | Cu3(1,3,5-benzenetricarboxylate)2 |
Cu3HTTP2 | Cu-2,3,6,7,19,11-hexahydroxy-triphenylene |
COF-5 | C9H4BO2, from 1,4-phenylene-bis(boronic acid) (PBBA) and 2,3,6,7,10,11-hexahydroxy-triphenylene (HHTP) |
LZU-1 | (C6H4N)n, from 1,3,5-triformylbenzene and 1,4-diaminobenzene |
MOF-5 | Zn4O(1,4-benzenedicarboxylate)3 |
UiO-66 | Zr6O4(OH)4(1,4-benzenedicarboxylate)6 |
MF-ZrBTB | [Zr6O4(OH)4]6[1,3,5-tris(4-carboxyphenyl)benzene acid]3 |
Cu-BTC (HKUST-1) | Cu3(1,3,5-benzenetricarboxylate)2 |
Ni-BHT | Ni-benzenhexathiolate |
Fe-BHT | Fe-benzenhexathiolate |
Co-BHT | Co-benzenhexathiolate |
NiIT | Bis(1-iminobenzene-2-thiolato)nickel(II) |
Co-BDC | Co-1,4-benzenedicarboxylate |
Ni3(HITP)2 | Ni3(2,3,6,7,10,11-hexaiminotriphenylene)2 |
MOF-525 | Zr6O4(OH)4(TpCPP-H2)3 |
Fe3(THT)2(NH4)3 | Fe3(THT, 2,3,6,7,10,11-triphenylenehexathiol)2(NH4)3 |
Cu-BHT | Cu-benzenhexathiolate |
Cu-TCNQ | Cu-tetracyanoquinodimethane |
NbOFFIVE-1-Ni | [NbOF5]2− and Ni(pyrazine)2 |
ALFFIVE-1-Ni | AlF5(H2O)]2− and Ni(pyrazine)2 |
Cu3(HHTP)2 | Cu3(2,3,6,7,10,11-hexahydroxytriphenylene)2 |
Zn(BDC)H2O | Zn-1,4-benzenedicarboxylate |
TPA-COF | [3+3] imine-linked COF prepared from tris(4-aminophenyl)amine and tris(4-formylphenyl)amine |
MAMS-1 | Ni8(5-tert-butyl-1,3-benzenedicarboxylic acid)6(μ-OH)4) |
Mg2(dobdc) | Mg2(2,5-dioxido-1,4-benzenedicarboxylate) |
Cu2(ndc)2(dabco) | Cu2(1,4-naphthalene dicarboxylate)2(1,4-diazabicyclo[2.2.2]-octane) |
ZIF-L | Polymorph of ZIF-8, Zn(2-methylimidazolate)2 |
diaz(Zn)-HCOONa | Polymorph of ZIF-8, Zn(2-methylimidazolate)2 |
Cd-PBM | {Cd4(4,4′-azopyridine)2(pyridine-2,3-dicarboxylate)4(H2O)2·9H2O}n |
UMCM-313 | Zr6 node and 2,5,8,11-tetrakis(4-carboxyphenyl)perylene |
NU-1000 | Zr6 node and 1,3,6,8-tetrakis(p-benzoate)pyrene |
Conflicts of interest
There are no conflicts of interest to declare.
References
- C. Tan, X. Cao, X. J. Wu, Q. He, J. Yang, X. Zhang, J. Chen, W. Zhao, S. Han, G. H. Nam, M. Sindoro and H. Zhang, Recent Advances in Ultrathin Two-Dimensional Nanomaterials, Chem. Rev., 2017, 117, 6225–6331 CrossRef CAS.
- P. Miró, M. Audiffred and T. Heine, An atlas of two-dimensional materials, Chem. Soc. Rev., 2014, 43, 6537–6554 RSC.
- D. L. Duong, S. J. Yun and Y. H. Lee, van der Waals Layered Materials: Opportunities and Challenges, ACS Nano, 2017, 11, 11803–11830 CrossRef CAS PubMed.
- G. R. Bhimanapati, Z. Lin, V. Meunier, Y. Jung, J. Cha, S. Das, D. Xiao, Y. Son, M. S. Strano, V. R. Cooper, L. Liang, S. G. Louie, E. Ringe, W. Zhou, S. S. Kim, R. R. Naik, B. G. Sumpter, H. Terrones and F. Xia,
et al., Recent Advances in Two-Dimensional Materials beyond Graphene, ACS Nano, 2015, 9, 11509–11539 CrossRef CAS PubMed.
- K. S. Novoselov, A. Mishchenko, A. Carvalho and A. H. Castro Neto, 2D materials and van der Waals heterostructures, Science, 2016, 353, aac9439 CrossRef CAS PubMed.
- Z. Meng, R. M. Stolz, L. Mendecki and K. A. Mirica, Electrically-transduced chemical sensors based on two-dimensional nanomaterials, Chem. Rev., 2019, 119, 478–598 CrossRef CAS PubMed.
- A. J. Mannix, B. Kiraly, M. C. Hersam and N. P. Guisinger, Synthesis and chemistry of elemental 2D materials, Nat. Rev. Chem., 2017, 1, 1–15 CrossRef.
- A. K. Geim and I. V. Grigorieva, van der Waals heterostructures, Nature, 2013, 499, 419–425 CrossRef CAS.
- N. Huang, P. Wang and D. Jiang, Covalent organic frameworks: a materials platform for structural and functional designs, Nat. Rev. Mater., 2016, 1, 1–19 Search PubMed.
- S. Y. Ding and W. Wang, Covalent organic frameworks (COFs): from design to applications, Chem. Soc. Rev., 2013, 42, 548–568 RSC.
- H. Furukawa, K. E. Cordova, M. O'Keeffe and O. M. Yaghi, The chemistry and applications of metal-organic frameworks, Science, 2013, 341, 1230444 CrossRef.
- B. Li, H. M. Wen, Y. Cui, W. Zhou, G. Qian and B. Chen, Emerging Multifunctional Metal–Organic Framework Materials, Adv. Mater., 2016, 28, 8819–8860 CrossRef CAS PubMed.
- M. G. Campbell, S. F. Liu, T. M. Swager and M. Dincă, Chemiresistive Sensor Arrays from Conductive 2D Metal-Organic Frameworks, J. Am. Chem. Soc., 2015, 137, 13780–13783 CrossRef CAS.
- S. Kitagawa, R. Kitaura and S. I. Noro, Functional porous coordination polymers, Angew. Chem., Int. Ed., 2004, 43, 2334–2375 CrossRef CAS.
- T. Islamoglu, S. Goswami, Z. Li, A. J. Howarth, O. K. Farha and J. T. Hupp, Postsynthetic Tuning of Metal-Organic Frameworks for Targeted Applications, Acc. Chem. Res., 2017, 50, 805–813 CrossRef CAS.
- W. Zheng, C. S. Tsang, L. Y. S. Lee and K. Y. Wong, Two-dimensional metal-organic framework and covalent-organic framework: synthesis and their energy-related applications, Mater. Today Chem., 2019, 12, 34–60 CrossRef CAS.
- D. D. Medina, T. Sick and T. Bein, Photoactive and Conducting Covalent Organic Frameworks, Adv. Energy Mater., 2017, 7, 1–8 Search PubMed.
- L. E. Kreno, K. Leong, O. K. Farha, M. Allendorf, R. P. Van Duyne and J. T. Hupp, Metal-organic framework materials as chemical sensors, Chem. Rev., 2012, 112, 1105–1125 CrossRef CAS.
- Z. Y. Yeo, S. P. Chai, P. W. Zhu and A. R. Mohamed, An overview: synthesis of thin films/membranes of metal organic frameworks and its gas separation performances, RSC Adv., 2014, 4, 54322–54334 RSC.
- X. Fang, B. Zong and S. Mao, Metal–Organic Framework-Based Sensors for Environmental Contaminant Sensing, Nano-Micro Lett., 2018, 10, 1–19 CrossRef PubMed.
- L. Sun, M. G. Campbell and M. Dincă, Electrically Conductive Porous Metal-Organic Frameworks, Angew. Chem., Int. Ed., 2016, 55, 3566–3579 CrossRef CAS PubMed.
- H. Wang, Z. Zeng, P. Xu, L. Li, G. Zeng, R. Xiao, Z. Tang, D. Huang, L. Tang, C. Lai, D. Jiang, Y. Liu, H. Yi, L. Qin, S. Ye, X. Ren and W. Tang, Recent progress in covalent organic framework thin films: fabrications, applications and perspectives, Chem. Soc. Rev., 2019, 48, 488–516 RSC.
- Y. Peng, Y. Li, Y. Ban, H. Jin, W. Jiao, Z. Liu and W. Yang, Metal-organic framework nanosheets as building blocks for molecular sieving membranes, Science, 2014, 346, 1356–1359 CrossRef CAS.
- G. Lan, Z. Li, S. S. Veroneau, Y.-Y. Zhu, Z. Xu, C. Wang and W. Lin, Photosensitizing Metal–Organic Layers for Efficient Sunlight-Driven Carbon Dioxide Reduction, J. Am. Chem. Soc., 2018, 140, 12369–12373 CrossRef CAS PubMed.
- J. L. Segura, S. Royuela and M. Mar Ramos, Post-synthetic modification of covalent organic frameworks, Chem. Soc. Rev., 2019, 48, 3903–3945 RSC.
- P. Samanta, A. V. Desai, B. Anothumakkool, M. M. Shirolkar, A. Karmakar, S. Kurungot and S. K. Ghosh, Enhanced proton conduction by post-synthetic covalent modification in a porous covalent framework, J. Mater. Chem. A, 2017, 5, 13659–13664 RSC.
- S. Mitra, H. S. Sasmal, T. Kundu, S. Kandambeth, K. Illath, D. Díaz Díaz and R. Banerjee, Targeted Drug Delivery in Covalent Organic Nanosheets (CONs) via Sequential Postsynthetic Modification, J. Am. Chem. Soc., 2017, 139, 4513–4520 CrossRef CAS PubMed.
- A. Schneemann, V. Bon, I. Schwedler, I. Senkovska, S. Kaskel and R. A. Fischer, Flexible metal–organic frameworks, Chem. Soc. Rev., 2014, 43, 6062–6096 RSC.
- M. Zhao, Y. Huang, Y. Peng, Z. Huang, Q. Ma and H. Zhang, Two-dimensional metal-organic framework nanosheets: synthesis and applications, Chem. Soc. Rev., 2018, 47, 6267–6295 RSC.
- V. Stavila, A. A. Talin and M. D. Allendorf, MOF-based electronic and opto-electronic devices, Chem. Soc. Rev., 2014, 43, 5994–6010 RSC.
- S. Li, K. Yang, C. Tan, X. Huang, W. Huang and H. Zhang, Preparation and applications of novel composites composed of metal-organic frameworks and two-dimensional materials, Chem. Commun., 2016, 52, 1555–1562 RSC.
-
Layered graphene, available at, http://www.graphenomenon.com/ Search PubMed.
-
N. Wang, et al., One-molecule-thick material has big advantages MIT News, 2012, available at, https://http://news.mit.edu/2012/graphene-molybdenum-disulfide-flat-materials-0823 Search PubMed.
- F. J. Claire, S. M. Tenney, M. M. Li, M. A. Siegler, J. S. Wagner, A. S. Hall and T. J. Kempa, Hierarchically Ordered Two-Dimensional Coordination
Polymers Assembled from Redox-Active Dimolybdenum Clusters, J. Am. Chem. Soc., 2018, 140, 10673–10676 CrossRef CAS PubMed.
- D. J. Ashworth and J. A. Foster, Metal-organic framework nanosheets (MONs): a new dimension in materials chemistry, J. Mater. Chem. A, 2018, 6, 16292–16307 RSC.
- G. Das, B. P. Biswal, S. Kandambeth, V. Venkatesh, G. Kaur, M. Addicoat, T. Heine, S. Verma and R. Banerjee, Chemical sensing in two dimensional porous covalent organic nanosheets, Chem. Sci., 2015, 6, 3931–3939 RSC.
- J. Liu, H. Yu, L. Wang, Z. Deng, K. R. Naveed, A. Nazir and F. Haq, Two-dimensional metal-organic frameworks nanosheets: synthesis strategies and applications, Inorg. Chim. Acta, 2018, 483, 550–564 CrossRef CAS.
- W. Liu, R. Yin, X. Xu, L. Zhang, W. Shi and X. Cao, Structural Engineering of Low-Dimensional Metal-Organic Frameworks: Synthesis, Properties, and Applications, Adv. Sci., 2019, 1802373 CrossRef PubMed.
- T. Kambe, R. Sakamoto, K. Hoshiko, K. Takada, M. Miyachi, J. H. Ryu, S. Sasaki, J. Kim, K. Nakazato, M. Takata and H. Nishihara, π-Conjugated nickel bis(dithiolene) complex nanosheet, J. Am. Chem. Soc., 2013, 135, 2462–2465 CrossRef CAS PubMed.
- R. Sakamoto, K. Takada, T. Pal, H. Maeda, T. Kambe and H. Nishihara, Coordination nanosheets (CONASHs): strategies, structures and functions, Chem. Commun., 2017, 53, 5781–5801 RSC.
- I. Stassen, D. De Vos and R. Ameloot, Vapor-Phase Deposition and Modification of Metal–Organic Frameworks: State-of-the-Art and Future Directions, Chem.–Eur. J., 2016, 22, 14452–14460 CrossRef CAS PubMed.
- M. J. Cliffe, E. Castillo-Martínez, Y. Wu, J. Lee, A. C. Forse, F. C. N. Firth, P. Z. Moghadam, D. Fairen-Jimenez, M. W. Gaultois, J. A. Hill, O. V. Magdysyuk, B. Slater, A. L. Goodwin and C. P. Grey, Metal-Organic Nanosheets Formed via Defect-Mediated Transformation of a Hafnium Metal-Organic Framework, J. Am. Chem. Soc., 2017, 139, 5397–5404 CrossRef CAS PubMed.
- M. D. Allendorf, A. Schwartzberg, V. Stavila and A. A. Talin, A roadmap to implementing metal-organic frameworks in electronic devices: challenges and critical directions, Chem.–Eur. J., 2011, 17, 11372–11388 CrossRef CAS PubMed.
- J. Liu and C. Wöll, Surface-supported metal-organic framework thin films: fabrication methods, applications, and challenges, Chem. Soc. Rev., 2017, 46, 5730–5770 RSC.
- M. Ko, L. Mendecki and K. A. Mirica, Conductive two-dimensional metal-organic frameworks as multifunctional materials, Chem. Commun., 2018, 54, 7873–7891 RSC.
- L. Heinke and C. Wöll, Surface-Mounted Metal–Organic Frameworks: Crystalline and Porous Molecular Assemblies for Fundamental Insights and Advanced Applications, Adv. Mater., 2019, 1806324 CrossRef PubMed.
- M. Lischka, R. Dong, M. Wang, N. Martsinovich, M. Fritton, L. Grossmann, W. M. Heckl, X. Feng and M. Lackinger, Competitive Metal Coordination of Hexaaminotriphenylene on Cu(111) by Intrinsic Copper Versus Extrinsic Nickel Adatoms, Chem.–Eur. J., 2019, 25, 1975–1983 CrossRef CAS PubMed.
- S. Kim, H. Wang and Y. M. Lee, 2D Nanosheets and Their Composite Membranes for Water, Gas, and Ion Separation, Angew. Chem., Int. Ed., 2019, 58, 2–18 CrossRef.
- C. Zhang, B.-H. Wu, M.-Q. Ma, Z. Wang and Z.-K. Xu, Ultrathin metal/covalent–organic framework membranes towards ultimate separation, Chem. Soc. Rev., 2019, 48, 3811–3841 RSC.
- S. Yuan, X. Li, J. Zhu, G. Zhang, P. Van Puyvelde and B. Van Der Bruggen, Covalent organic frameworks for membrane separation, Chem. Soc. Rev., 2019, 48, 2665–2681 RSC.
- E. Barankova, X. Tan, L. F. Villalobos, E. Litwiller and K. V. Peinemann, A Metal Chelating Porous Polymeric Support: The Missing Link for a Defect-Free Metal–Organic Framework Composite Membrane, Angew. Chem., Int. Ed., 2017, 56, 2965–2968 CrossRef CAS PubMed.
- M. Wu, H. Ye, F. Zhao and B. Zeng, High-quality metal-organic framework ZIF-8 membrane supported on electrodeposited ZnO/2-methylimidazole nanocomposite: efficient adsorbent for the enrichment of acidic drugs, Sci. Rep., 2017, 7, 1–9 CrossRef PubMed.
- J. Sun, H. T. Kwon and H. K. Jeong, Continuous synthesis of high quality metal–organic framework HKUST-1 crystals and composites via aerosol-assisted synthesis, Polyhedron, 2018, 153, 226–233 CrossRef CAS.
- L. M. Salonen, D. D. Medina, E. Carbó-Argibay, M. G. Goesten, L. Mafra, N. Guldris, J. M. Rotter, D. G. Stroppa and C. Rodríguez-Abreu, A supramolecular strategy based on molecular dipole moments for high-quality covalent organic frameworks, Chem. Commun., 2016, 52, 7986–7989 RSC.
- M. Calik, T. Sick, M. Dogru, M. Döblinger, S. Datz, H. Budde, A. Hartschuh, F. Auras and T. Bein, From Highly Crystalline to Outer Surface-Functionalized Covalent Organic Frameworks-A Modulation Approach, J. Am. Chem. Soc., 2016, 138, 1234–1239 CrossRef CAS PubMed.
- S. Benmansour, A. Abhervé, P. Gómez-Claramunt, C. Vallés-García and C. J. Gómez-García, Nanosheets of Two-Dimensional Magnetic and Conducting Fe(II)/Fe(III) Mixed-Valence Metal-Organic Frameworks, ACS Appl. Mater. Interfaces, 2017, 9, 26210–26218 CrossRef CAS PubMed.
- W. M. Liao, J. H. Zhang, S. Y. Yin, H. Lin, X. Zhang, J. Wang, H. P. Wang, K. Wu, Z. Wang, Y. N. Fan, M. Pan and C. Y. Su, Tailoring exciton and excimer emission in an exfoliated ultrathin 2D metal-organic framework, Nat. Commun., 2018, 9, 1–9 CrossRef PubMed.
- Y. H. Luo, C. Chen, C. He, Y. Y. Zhu, D. L. Hong, X. T. He, P. J. An, H. S. Wu and B. W. Sun, Single-Layered Two-Dimensional Metal-Organic Framework Nanosheets as an in Situ Visual Test Paper for Solvents, ACS Appl. Mater. Interfaces, 2018, 10, 28860–28867 CrossRef CAS PubMed.
- Y. Ding, Y. P. Chen, X. Zhang, L. Chen, Z. Dong, H. L. Jiang, H. Xu and H. C. Zhou, Controlled Intercalation and Chemical Exfoliation of Layered Metal-Organic Frameworks Using a Chemically Labile Intercalating Agent, J. Am. Chem. Soc., 2017, 139, 9136–9139 CrossRef CAS PubMed.
- J. Huang, Y. Li, R. K. Huang, C. T. He, L. Gong, Q. Hu, L. Wang, Y. T. Xu, X. Y. Tian, S. Y. Liu, Z. M. Ye, F. Wang, D. D. Zhou, W. X. Zhang and J. P. Zhang, Electrochemical Exfoliation of Pillared-Layer Metal–Organic Framework to Boost the Oxygen Evolution Reaction, Angew. Chem., Int. Ed., 2018, 57, 4632–4636 CrossRef CAS.
- C. Kutzscher, A. Gelbert, S. Ehrling, C. Schenk, I. Senkovska and S. Kaskel, Amine assisted top-down delamination of the two-dimensional metal-organic framework Cu2(bdc)2, Dalton Trans., 2017, 46, 16480–16484 RSC.
- D. J. Ashworth, A. Cooper, M. Trueman, R. W. M. Al-Saedi, L. D. Smith, A. J. H. M. Meijer and J. A. Foster, Ultrasonic Exfoliation of Hydrophobic and Hydrophilic Metal–Organic Frameworks To Form Nanosheets, Chem.–Eur. J., 2018, 24, 17986–17996 CrossRef CAS PubMed.
- X. Chen, Y. Li, L. Wang, Y. Xu, A. Nie, Q. Li, F. Wu, W. Sun, X. Zhang, R. Vajtai, P. M. Ajayan, L. Chen and Y. Wang, High-Lithium-Affinity Chemically Exfoliated 2D Covalent Organic Frameworks, Adv. Mater., 2019, 1–8, 1901640 CrossRef PubMed.
- Y. Zhu, X. Chen, Y. Cao, W. Peng, Y. Li, G. Zhang, F. Zhang and X. Fan, Reversible intercalation and exfoliation of layered covalent triazine frameworks for enhanced lithium ion storage, Chem. Commun., 2019, 55, 1434–1437 RSC.
- P. Peng, L. Shi, F. Huo, S. Zhang, C. Mi, Y. Cheng and Z. Xiang, In Situ Charge Exfoliated Soluble Covalent Organic Framework Directly Used for Zn-Air Flow Battery, ACS Nano, 2019, 13, 878–884 CrossRef CAS PubMed.
- M. A. Khayum, S. Kandambeth, S. Mitra, S. B. Nair, A. Das, S. S. Nagane, R. Mukherjee and R. Banerjee, Chemically Delaminated Free-Standing Ultrathin Covalent Organic Nanosheets, Angew. Chem., Int. Ed., 2016, 55, 15604–15608 CrossRef CAS PubMed.
- K. Yuan, T. Song, X. Zhu, B. Li, B. Han, L. Zheng, J. Li, X. Zhang and W. Hu, Construction of Large-Area Ultrathin Conductive Metal–Organic Framework Films through Vapor-Induced Conversion, Small, 2019, 15, 1–6 Search PubMed.
- R. Dong, T. Zhang and X. Feng, Interface-Assisted Synthesis of 2D Materials: Trend and Challenges, Chem. Rev., 2018, 118, 6189–6325 CrossRef CAS PubMed.
- M. Shete, P. Kumar, J. E. Bachman, X. Ma, Z. P. Smith, W. Xu, K. A. Mkhoyan, J. R. Long and M. Tsapatsis, On the direct synthesis of Cu(BDC) MOF nanosheets and their performance in mixed matrix membranes, J. Membr. Sci., 2018, 549, 312–320 CrossRef CAS.
- X. Huang, P. Sheng, Z. Tu, F. Zhang, J. Wang, H. Geng, Y. Zou, C. A. Di, Y. Yi, Y. Sun, W. Xu and D. Zhu, A two-dimensional π-d conjugated coordination polymer with extremely high electrical conductivity and ambipolar transport behaviour, Nat. Commun., 2015, 6, 1–8 CrossRef.
- Y. Li, M. Zhang, X. Guo, R. Wen, X. Li, X. Li, S. Li and L. Ma, Growth of high-quality covalent organic framework nanosheets at the interface of two miscible organic solvents, Nanoscale Horiz., 2018, 3, 205–212 RSC.
- M. Pfeffermann, R. Dong, R. Graf, W. Zajaczkowski, T. Gorelik, W. Pisula, A. Narita, K. Müllen and X. Feng, Free-Standing Monolayer Two-Dimensional Supramolecular Organic Framework with Good Internal Order, J. Am. Chem. Soc., 2015, 137, 14525–14532 CrossRef CAS PubMed.
- Z. Wang, Q. Yu, Y. Huang, H. An, Y. Zhao, Y. Feng, X. Li, X. Shi, J. Liang, F. Pan, P. Cheng, Y. Chen, S. Ma and Z. Zhang, PolyCOFs: A New Class of Freestanding Responsive Covalent Organic Framework Membranes with High Mechanical Performance, ACS Cent. Sci., 2019 DOI:10.1021/acscentsci.9b00212.
- F. Wang, L. Valentino, A. R. Corcos, G. M. Stiehl, B. J. Mariñas, D. C. Ralph, W. R. Dichtel, H. B. Balch and M. Matsumoto, Lewis-Acid-Catalyzed Interfacial Polymerization of Covalent Organic Framework Films, Chem, 2018, 4, 308–317 Search PubMed.
- T. Rodenas, I. Luz, G. Prieto, B. Seoane, H. Miro, A. Corma, F. Kapteijn, F. X. Llabrés I Xamena and J. Gascon, Metal-organic framework nanosheets in polymer composite materials for gas separation, Nat. Mater., 2015, 14, 48–55 CrossRef CAS PubMed.
- I.-F. Chen, C.-F. Lu and W.-F. Su, Highly Conductive 2D Metal–Organic Framework Thin Film Fabricated by Liquid–Liquid Interfacial Reaction Using One-Pot-Synthesized Benzenehexathiol, Langmuir, 2018, 34, 15754–15762 CrossRef CAS PubMed.
- M. Zhao, Y. Wang, Q. Ma, Y. Huang, X. Zhang, J. Ping, Z. Zhang, Q. Lu, Y. Yu, H. Xu, Y. Zhao and H. Zhang, Ultrathin 2D Metal-Organic Framework Nanosheets, Adv. Mater., 2015, 27, 7372–7378 CrossRef CAS PubMed.
- F. Xue, P. Kumar, W. Xu, K. A. Mkhoyan and M. Tsapatsis, Direct Synthesis of 7 nm-Thick Zinc(II)-Benzimidazole-Acetate Metal-Organic Framework Nanosheets, Chem. Mater., 2018, 30, 69–73 CrossRef CAS.
- G. Xu, T. Yamada, K. Otsubo, S. Sakaida and H. Kitagawa, Facile ‘modular assembly’ for fast construction of a highly oriented crystalline MOF nanofilm, J. Am. Chem. Soc., 2012, 134, 16524–16527 CrossRef CAS.
- V. Rubio-Giménez, S. Tatay, F. Volatron, F. J. Martínez-Casado, C. Martí-Gastaldo and E. Coronado, High-Quality Metal-Organic Framework Ultrathin Films for Electronically Active Interfaces, J. Am. Chem. Soc., 2016, 138, 2576–2584 CrossRef PubMed.
- J. L. Zhuang, A. Terfort and C. Wöll, Formation of oriented and patterned films of metal-organic frameworks by liquid phase epitaxy: a review, Coord. Chem. Rev., 2016, 307, 391–424 CrossRef CAS.
- T. Haraguchi, K. Otsubo and H. Kitagawa, Emergence of Surface- and Interface-Induced Structures and Properties in Metal–Organic Framework Thin Films, Eur. J. Inorg. Chem., 2018, 2018, 1697–1706 CrossRef CAS.
- W. Guo, M. Zha, Z. Wang, E. Redel, Z. Xu and C. Wöll, Improving the Loading Capacity of Metal-Organic Framework Thin Films Using Optimized Linkers, ACS Appl. Mater. Interfaces, 2016, 8, 24699–24702 CrossRef CAS PubMed.
- J. Liu, T. Wächter, A. Irmler, P. G. Weidler, H. Gliemann, F. Pauly, V. Mugnaini, M. Zharnikov and C. Wöll, Electric transport properties of surface-anchored metal-organic frameworks and the effect of ferrocene loading, ACS Appl. Mater. Interfaces, 2015, 7, 9824–9830 CrossRef CAS PubMed.
- J. Liu, M. Paradinas, L. Heinke, M. Buck, C. Ocal, V. Mugnaini and C. Wöll, Film Quality and Electronic Properties of a Surface-Anchored Metal-Organic Framework Revealed by using a Multi-technique Approach, ChemElectroChem, 2016, 3, 713–718 CrossRef CAS.
- D. Van Gough, T. N. Lambert, D. R. Wheeler, M. A. Rodriguez, M. T. Brumbach, M. D. Allendorf and E. D. Spoerke, Controlled nucleation and growth of pillared paddlewheel framework nanostacks onto chemically modified surfaces, ACS Appl. Mater. Interfaces, 2014, 6, 1509–1514 CrossRef CAS PubMed.
- V. Stavila, J. Volponi, A. M. Katzenmeyer, M. C. Dixon and M. D. Allendorf, Kinetics and mechanism of metal–organic framework thin film growth: systematic investigation of HKUST-1 deposition on QCM electrodes, Chem. Sci., 2012, 3, 1531 RSC.
- M. Framework, A. A. Talin, A. Centrone, A. C. Ford, M. E. Foster, V. Stavila, P. Haney, R. A. Kinney, V. Szalai, F. E. Gabaly, H. P. Yoon, F. Léonard and M. D. Allendorf, Tunable Electrical Conductivity in Metal-Organic Framework
Thin-Film Devices, Science, 2014, 343, 66–70 CrossRef.
- S. Wannapaiboon, K. Sumida, K. Dilchert, M. Tu, S. Kitagawa, S. Furukawa and R. A. Fischer, Enhanced properties of metal-organic framework thin films fabricated: via a coordination modulation-controlled layer-by-layer process, J. Mater. Chem. A, 2017, 5, 13665–13673 RSC.
- J. F. Stoddart, X. Gong, G. Wang, C. Cheng, C.-W. Kung, A. Facchetti, J.-M. Han, A. C.-H. Sue, T. J. Marks, Z. Liu, K. Cai, O. K. Farha, Y. Y. Botros, Y. Shi, P. Li, H. Chen, Q.-H. Guo and D. Shen, Epitaxial Growth of γ-Cyclodextrin-Containing Metal–Organic Frameworks Based on a Host–Guest Strategy, J. Am. Chem. Soc., 2018, 140, 11402–11407 CrossRef PubMed.
- G. Lu, O. K. Farha, W. Zhang, F. Huo and J. T. Hupp, Engineering ZIF-8 thin films for hybrid MOF-based devices, Adv. Mater., 2012, 24, 3970–3974 CrossRef CAS PubMed.
- H. Ji, S. Hwang, K. Kim, C. Kim and N. C. Jeong, Direct in Situ Conversion of Metals into Metal-Organic Frameworks: A Strategy for the Rapid Growth of MOF Films on Metal Substrates, ACS Appl. Mater. Interfaces, 2016, 8, 32414–32420 CrossRef CAS PubMed.
- D. Kim and A. Coskun, Graphene oxide-templated preferential growth of continuous MOF thin films, CrystEngComm, 2016, 18, 4013–4017 RSC.
- K. Sakata, S. Kashiyama, G. Matsuo, S. Uemura, N. Kimizuka and M. Kunitake, Growth of Two-Dimensional Metal–Organic Framework Nanosheet Crystals on Graphite Substrates by Thermal Equilibrium Treatment in Acetic Acid Vapor, ChemNanoMat, 2015, 1, 259–263 CrossRef CAS.
- B. Hoppe, K. D. J. Hindricks, D. P. Warwas, H. A. Schulze, A. Mohmeyer, T. J. Pinkvos, S. Zailskas, M. R. Krey, C. Belke, S. König, M. Fröba, R. J. Haug and P. Behrens, Graphene-like metal-organic frameworks: morphology control, optimization of thin film electrical conductivity and fast sensing applications, CrystEngComm, 2018, 20, 6458–6471 RSC.
- V. Chernikova, O. Shekhah and M. Eddaoudi, Advanced Fabrication Method for the Preparation of MOF Thin Films: Liquid-Phase Epitaxy Approach Meets Spin Coating Method, ACS Appl. Mater. Interfaces, 2016, 8, 20459–20464 CrossRef CAS PubMed.
- M. C. So, S. Jin, H.-J. Son, G. P. Wiederrecht, O. K. Farha and J. T. Hupp, Layer-by-layer fabrication of oriented porous thin films based on porphyrin-containing metal-organic frameworks, J. Am. Chem. Soc., 2013, 135, 15698–15701 CrossRef CAS PubMed.
- Y. Wang, L. Li, L. Yan, X. Gu, P. Dai, D. Liu, J. G. Bell, G. Zhao, X. Zhao and K. M. Thomas, Bottom-Up Fabrication of Ultrathin 2D Zr Metal-Organic Framework Nanosheets through a Facile Continuous Microdroplet Flow Reaction, Chem. Mater., 2018, 30, 3048–3059 CrossRef CAS.
- A. M. Evans, L. R. Parent, N. C. Flanders, R. P. Bisbey, E. Vitaku, L. X. Chen, N. C. Gianneschi and W. R. Dichtel, Seeded Growth of Single-Crystal Two-Dimensional Covalent Organic Frameworks, Science, 2018, 7883 Search PubMed.
- R. P. Bisbey, C. R. DeBlase, B. J. Smith and W. R. Dichtel, Two-dimensional Covalent Organic Framework Thin Films Grown in Flow, J. Am. Chem. Soc., 2016, 138, 11433–11436 CrossRef CAS PubMed.
- Y. Zhao, L. Guo, F. Gándara, Y. Ma, Z. Liu, C. Zhu, H. Lyu, C. A. Trickett, E. A. Kapustin, O. Terasaki and O. M. Yaghi, A Synthetic Route for Crystals of Woven Structures,
Uniform Nanocrystals, and Thin Films of Imine Covalent Organic Frameworks, J. Am. Chem. Soc., 2017, 139, 13166–13172 CrossRef CAS PubMed.
- P. Serp, P. Kalck and R. Feurer, Chemical vapor deposition methods for the controlled preparation of supported catalytic materials, Chem. Rev., 2002, 102, 3085–3128 CrossRef CAS PubMed.
- Z. Cai, B. Liu, X. Zou and H. Cheng, Chemical Vapor Deposition Growth and Applications of Two-Dimensional Materials and Their Heterostructures, Chem. Rev., 2018, 118, 6091–6133 CrossRef CAS PubMed.
- P. C. Lemaire, J. Zhao, P. S. Williams, H. J. Walls, S. D. Shepherd, M. D. Losego, G. W. Peterson and G. N. Parsons, Copper Benzenetricarboxylate Metal-Organic Framework Nucleation Mechanisms on Metal Oxide Powders and Thin Films formed by Atomic Layer Deposition, ACS Appl. Mater. Interfaces, 2016, 8, 9514–9522 CrossRef CAS.
- E. Ahvenniemi and M. Karppinen, Atomic/molecular layer deposition: a direct gas-phase route to crystalline metal-organic framework thin films, Chem. Commun., 2016, 52, 1139–1142 RSC.
- E. Ahvenniemi and M. Karppinen, In Situ Atomic/Molecular Layer-by-Layer Deposition of Inorganic-Organic Coordination Network Thin Films from Gaseous Precursors, Chem. Mater., 2016, 28, 6260–6265 CrossRef CAS.
- E. Ahvenniemi and M. Karppinen, ALD/MLD processes for Mn and Co based hybrid thin films, Dalton Trans., 2016, 45, 10730 RSC.
- L. D. Salmi, M. J. Heikkilä, E. Puukilainen, T. Sajavaara, D. Grosso and M. Ritala, Studies on atomic layer deposition of MOF-5 thin films, Microporous Mesoporous Mater., 2013, 182, 147–154 CrossRef CAS.
- I. Stassen, N. Campagnol, J. Fransaer, P. Vereecken, D. De Vos and R. Ameloot, Solvent-free synthesis of supported ZIF-8 films and patterns through transformation of deposited zinc oxide precursors, CrystEngComm, 2013, 15, 9308–9311 RSC.
- I. Stassen, M. Styles, G. Grenci, H. Van Gorp, W. Vanderlinden, S. De Feyter, P. Falcaro, D. De Vos, P. Vereecken and R. Ameloot, Chemical vapour deposition of zeolitic imidazolate framework thin films, Nat. Mater., 2016, 15, 304–310 CrossRef CAS PubMed.
- S. Spitzer, A. Rastgoo-Lahrood, K. MacKnapp, V. Ritter, S. Sotier, W. M. Heckl and M. Lackinger, Solvent-free on-surface synthesis of boroxine COF monolayers, Chem. Commun., 2017, 53, 5147–5150 RSC.
-
F. J. Claire, M. A. Solomos, J. Kim and T. Kempa, unpublished work.
- M. M. Li, F. J. Claire, M. A. Solomos, S. M. Tenney, S. A. Ivanov, M. A. Siegler and T. J. Kempa, Molecular chains of coordinated dimolybdenum isonicotinate paddlewheel clusters, RSC Adv., 2019, 9, 16492–16495 RSC.
- B. Garai, A. Mallick, A. Das, R. Mukherjee and R. Banerjee, Self-Exfoliated Metal-Organic Nanosheets through Hydrolytic Unfolding of Metal-Organic Polyhedra, Chem.–Eur. J., 2017, 23, 7361–7366 CrossRef CAS PubMed.
- S. Zhao, Y. Wang, J. Dong, C. T. He, H. Yin, P. An, K. Zhao, X. Zhang, C. Gao, L. Zhang, J. Lv, J. Wang, J. Zhang, A. M. Khattak, N. A. Khan, Z. Wei, J. Zhang, S. Liu, H. Zhao and Z. Tang, Ultrathin metal-organic framework nanosheets for electrocatalytic oxygen evolution, Nat. Energy, 2016, 1, 1–10 Search PubMed.
- D. N. Bunck and W. R. Dichtel, Bulk synthesis of exfoliated two-dimensional polymers using hydrazone-linked covalent organic frameworks, J. Am. Chem. Soc., 2013, 135, 14952–14955 CrossRef CAS PubMed.
- Y. Zhao, L. Jiang, L. Shangguan, L. Mi, A. Liu and S. Liu, Synthesis of porphyrin-based two-dimensional metal-organic framework nanodisk with small size and few layers, J. Mater. Chem. A, 2018, 6, 2828–2833 RSC.
- J. Nicks, J. Zhang and J. A. Foster, Tandem catalysis by ultrathin metal–organic nanosheets formed through post-synthetic functionalisation of a layered framework, Chem. Commun., 2019, 55, 8788–8791 RSC.
- V. K.-M. Au, K. Nakayashiki, H. Huang, S. Suginome, H. Sato and T. Aida, Stepwise Expansion of Layered Metal–Organic Frameworks for Nonstochastic Exfoliation into Porous Nanosheets, J. Am. Chem. Soc., 2018, 141, 53–57 CrossRef PubMed.
- Z. Wang, J. Liu, B. Lukose, Z. Gu, P. G. Weidler, H. Gliemann, T. Heine and C. Wöll, Nanoporous designer solids with huge lattice constant gradients: multiheteroepitaxy of metal-organic frameworks, Nano Lett., 2014, 14, 1526–1529 CrossRef CAS PubMed.
- I. Stassen, M. Styles, T. Van Assche, N. Campagnol, J. Fransaer, J. Denayer, J. C. Tan, P. Falcaro, D. De Vos and R. Ameloot, Electrochemical film deposition of the zirconium metal-organic framework uio-66 and application in a miniaturized sorbent trap, Chem. Mater., 2015, 27, 1801–1807 CrossRef CAS.
- J. W. Colson, A. R. Woll, A. Mukherjee, M. P. Levendorf, E. L. Spitler, V. B. Shields, M. G. Spencer, J. Park and W. R. Dichtel, Oriented 2D Covalent Organic Framework Thin Films on Single-Layer Graphene, Science, 2011, 332, 228–232 CrossRef CAS.
- S. Motoyama, R. Makiura, O. Sakata and H. Kitagawa, Highly Crystalline Nanofilm by Layering of Porphyrin Metal-Organic Framework Sheets, J. Am. Chem. Soc., 2011, 2, 5640–5643 CrossRef.
- R. Matsuoka, R. Toyoda, R. Shiotsuki, N. Fukui, K. Wada, H. Maeda, R. Sakamoto, S. Sasaki, H. Masunaga, K. Nagashio and H. Nishihara, Expansion of the Graphdiyne Family: A Triphenylene-Cored Analogue, ACS Appl. Mater. Interfaces, 2019, 11, 2730–2733 CrossRef CAS PubMed.
- W. Lu and X. Wu, Ni-MOF nanosheet arrays: efficient non-noble-metal electrocatalysts for non-enzymatic monosaccharide sensing, New J. Chem., 2018, 42, 3180–3183 RSC.
- A. Ayala, J. D. Tarver, K. E. Hurst, C. M. Brown, P. A. Parilla, T. Gennett, H. Z. H. Jiang, M. T. Kapelewski, J. R. Long, T. Runčevski and S. A. FitzGerald, Record High Hydrogen Storage Capacity in the Metal–Organic Framework Ni2(m-dobdc) at Near-Ambient Temperatures, Chem. Mater., 2018, 30, 8179–8189 CrossRef.
- S. Chandra, S. Kandambeth, B. P. Biswal, B. Lukose, S. M. Kunjir, M. Chaudhary, R. Babarao, T. Heine and R. Banerjee, Chemically stable multilayered covalent organic nanosheets from covalent organic frameworks via mechanical delamination, J. Am. Chem. Soc., 2013, 135, 17853–17861 CrossRef CAS PubMed.
- I. Berlanga, M. L. Ruiz-González, J. M. González-Calbet, J. L. G. Fierro, R. Mas-Ballesté and F. Zamora, Delamination of layered covalent organic frameworks, Small, 2011, 7, 1207–1211 CrossRef CAS.
- D. D. Medina, V. Werner, F. Auras, R. Tautz, M. Dogru, J. Schuster, S. Linke, M. Döblinger, J. Feldmann, P. Knochel and T. Bein, Oriented thin films of a benzodithiophene covalent organic framework, ACS Nano, 2014, 8, 4042–4052 CrossRef CAS.
- G. Li, K. Zhang and T. Tsuru, Two-Dimensional Covalent Organic Framework (COF) Membranes Fabricated via the Assembly of Exfoliated COF Nanosheets, ACS Appl. Mater. Interfaces, 2017, 9, 8433–8436 CrossRef CAS.
- S. Wu, L. Qin, K. Zhang, Z. Xin and S. Zhao, Ultrathin 2D metal-organic framework nanosheets prepared via sonication exfoliation of membranes from interfacial growth and exhibition of enhanced catalytic activity by their gold nanocomposites, RSC Adv., 2019, 9, 9386–9391 RSC.
- N. Lahiri, N. Lotfizadeh, R. Tsuchikawa, V. V. Deshpande and J. Louie, Hexaaminobenzene as a building block for a family of 2D coordination polymers, J. Am. Chem. Soc., 2017, 139, 19–22 CrossRef CAS.
- S. Kumar, J. A. Lee, S. Maheshwari, A. Mittal, C. Sung, M. Cococcioni, L. F. Francis, A. V. Mccormick, K. A. Mkhoyan and M. Tsapatsis, Dispersible Exfoliated Zeolite Nanosheets and Their Application as a Selective Membrane, Science, 2011, 734, 72–76 Search PubMed.
- G. Zhan and H. C. Zeng, Synthesis and Functionalization of Oriented Metal–Organic-Framework Nanosheets : Toward a Series of 2D Catalysts, Adv. Funct. Mater., 2016, 26, 3268–3281 CrossRef CAS.
- T. He, B. Ni, S. Zhang, Y. Gong, H. Wang, L. Gu and J. Zhuang, Ultrathin 2D Zirconium Metal–Organic Framework Nanosheets : Preparation and Application in Photocatalysis, Small, 2018, 14, 1–6 Search PubMed.
- A. Dmitriev, H. Spillmann, N. Lin, J. V. Barth and K. Kern, Modular assembly of two-dimensional metal-organic coordination networks at a metal surface, Angew. Chem., Int. Ed., 2003, 42, 2670–2673 CrossRef CAS PubMed.
- P. Amo-Ochoa, L. Welte, R. González-Prieto, P. J. Sanz Miguel, C. J. Gómez-García, E. Mateo-Martí, S. Delgado, J. Gómez-Herrero and F. Zamora, Single layers of a multifunctional laminar Cu(i,ii) coordination polymer, Chem. Commun., 2010, 46, 3262–3264 RSC.
- L. Xu, X. Zhou, W. Q. Tian, T. Gao, Y. F. Zhang, S. Lei and Z. F. Liu, Surface-confined single-layer covalent organic framework on single-layer graphene grown on copper foil, Angew. Chem., Int. Ed., 2014, 53, 9564–9568 CrossRef CAS PubMed.
- C. Chen, T. Joshi, H. Li, A. D. Chavez, Z. Pedramrazi, P. N. Liu, H. Li, W. R. Dichtel, J. L. Bredas and M. F. Crommie, Local Electronic Structure of a Single-Layer Porphyrin-Containing Covalent Organic Framework, ACS Nano, 2018, 12, 385–391 CrossRef CAS PubMed.
- C. W. Kung, K. Otake, C. T. Buru, S. Goswami, Y. Cui, J. T. Hupp, A. M. Spokoyny and O. K. Farha, Increased Electrical Conductivity in a Mesoporous Metal-Organic Framework Featuring Metallacarboranes Guests, J. Am. Chem. Soc., 2018, 140, 3871–3875 CrossRef CAS PubMed.
- M. S. Yao, X. J. Lv, Z. H. Fu, W. H. Li, W. H. Deng, G. D. Wu and G. Xu, Layer-by-Layer Assembled Conductive Metal–Organic Framework Nanofilms for Room-Temperature Chemiresistive Sensing, Angew. Chem., Int. Ed., 2017, 56, 16510–16514 CrossRef CAS PubMed.
- T. Kambe, R. Sakamoto, T. Kusamoto, T. Pal, N. Fukui, K. Hoshiko, T. Shimojima, Z. Wang, T. Hirahara, K. Ishizaka, S. Hasegawa, F. Liu and H. Nishihara, Redox control and high conductivity of nickel bis(dithiolene) complex π-nanosheet: a potential organic two-dimensional topological insulator, J. Am. Chem. Soc., 2014, 136, 14357–14360 CrossRef CAS.
- Y. Wu, S. J. Moorhouse and D. O'Hare, Time-Resolved in Situ Diffraction Reveals a Solid-State Rearrangement during Solvothermal MOF Synthesis, Chem. Mater., 2015, 27, 7236–7239 CrossRef CAS.
- J. Cravillon, C. A. Schröder, R. Nayuk, J. Gummel, K. Huber and M. Wiebcke, Fast nucleation and growth of ZIF-8 nanocrystals monitored by time-resolved in situ small-angle and wide-angle X-ray scattering, Angew. Chem., Int. Ed., 2011, 50, 8067–8071 CrossRef CAS PubMed.
- M. W. Terban, D. Banerjee, S. Ghose, B. Medasani, A. Shukla, B. A. Legg, Y. Zhou, Z. Zhu, M. L. Sushko, J. J. De Yoreo, J. Liu, P. K. Thallapally and S. J. L. Billinge, Early stage structural development of prototypical zeolitic imidazolate framework (ZIF) in solution, Nanoscale, 2018, 10, 4291–4300 RSC.
- M. M. Biener, J. Biener, R. Schalek and C. M. Friend, Growth of nanocrystalline on Au (111) studied by in situ scanning tunneling microscopy tunneling microscopy, J. Chem. Phys., 2004, 121, 12010–12016 CrossRef CAS PubMed.
- S. H. Dave, C. Gong, A. W. Robertson, J. H. Warner and C. Grossman, Chemistry and Structure of Graphene Oxide via Direct Imaging, ACS Nano, 2016, 10, 7515–7522 CrossRef CAS.
- L. D. B. Mandemaker, M. Filez, G. Delen, H. Tan, X. Zhang, D. Lohse and B. M. Weckhuysen, Time-Resolved in Situ Liquid-Phase Atomic Force Microscopy and Infrared Nanospectroscopy during the Formation of Metal-Organic Framework Thin Films, J. Phys. Chem. Lett., 2018, 9, 1838–1844 CrossRef CAS.
- J. Zhao, B. Kalanyan, H. F. Barton, B. A. Sperling, G. N. Parsons, N. Carolina and U. States, In Situ Time-Resolved Attenuated Total Reflectance Infrared Spectroscopy for Probing Metal−Organic Framework Thin Film Growth, Chem. Mater., 2017, 29, 8804–8810 CrossRef CAS PubMed.
- A. J. Clough, J. W. Yoo, M. H. Mecklenburg and S. C. Marinescu, Two-dimensional metal-organic surfaces for efficient hydrogen evolution from water, J. Am. Chem. Soc., 2015, 137, 118–121 CrossRef CAS PubMed.
- R. Dong, M. Pfeffermann, H. Liang, Z. Zheng, X. Zhu, J. Zhang and X. Feng, Large-Area, Free-Standing, Two-Dimensional Supramolecular Polymer Single-Layer Sheets for Highly Efficient Electrocatalytic Hydrogen Evolution, Angew. Chem., Int. Ed., 2015, 54, 12058–12063 CrossRef CAS PubMed.
- C. A. Downes, A. J. Clough, K. Chen, J. W. Yoo and S. C. Marinescu, Evaluation of the H2 Evolving Activity of Benzenehexathiolate Coordination Frameworks and the Effect of Film Thickness on H2 Production, ACS Appl. Mater. Interfaces, 2018, 10, 1719–1727 CrossRef CAS PubMed.
- X. Sun, K. H. Wu, R. Sakamoto, T. Kusamoto, H. Maeda, X. Ni, W. Jiang, F. Liu, S. Sasaki, H. Masunaga and H. Nishihara, Bis(aminothiolato)nickel nanosheet as a redox switch for conductivity and an electrocatalyst for the hydrogen evolution reaction, Chem. Sci., 2017, 8, 8078–8085 RSC.
- D. Zhu, J. Liu, Y. Zhao, Y. Zheng and S. Z. Qiao, Engineering 2D Metal–Organic Framework/MoS2 Interface for Enhanced Alkaline Hydrogen Evolution, Small, 2019, 15, 1–8 Search PubMed.
- J. Duan, S. Chen and C. Zhao, Ultrathin metal-organic framework array for efficient electrocatalytic water splitting, Nat. Commun., 2017, 8, 1–7 CrossRef PubMed.
- F.-L. Li, P. Wang, X. Huang, D. J. Young, H.-F. Wang, P. Braunstein and J.-P. Lang, Large-Scale, Bottom-Up Synthesis of Binary Metal-Organic Framework Nanosheets for Efficient Water Oxidation, Angew. Chem., Int. Ed., 2019, 58, 7051–7056 CrossRef CAS PubMed.
- E. M. Miner, T. Fukushima, D. Sheberla, L. Sun, Y. Surendranath and M. Dincă, Electrochemical oxygen reduction catalysed by Ni3(hexaiminotriphenylene)2, Nat. Commun., 2016, 7, 1–7 Search PubMed.
- N. Kornienko, Y. Zhao, C. S. Kley, C. Zhu, D. Kim, S. Lin, C. J. Chang, O. M. Yaghi and P. Yang, Metal-Organic Frameworks for Electrocatalytic Reduction of Carbon Dioxide, J. Am. Chem. Soc., 2015, 137, 14129–14135 CrossRef CAS PubMed.
- I. Hod, M. D. Sampson, P. Deria, C. P. Kubiak, O. K. Farha and J. T. Hupp, Fe-Porphyrin-Based Metal-Organic Framework Films as High-Surface Concentration, Heterogeneous Catalysts for Electrochemical Reduction of CO2, ACS Catal., 2015, 5, 6302–6309 CrossRef CAS.
- Y. Huang, M. Zhao, S. Han, Z. Lai, J. Yang, C. Tan, Q. Ma, Q. Lu, J. Chen, X. Zhang, Z. Zhang, B. Li, B. Chen, Y. Zong and H. Zhang, Growth of Au Nanoparticles on 2D Metalloporphyrinic Metal-Organic Framework Nanosheets Used as Biomimetic Catalysts for Cascade Reactions, Adv. Mater., 2017, 29, 1–5 Search PubMed.
- K. Rui, G. Zhao, Y. Chen, Y. Lin, Q. Zhou, J. Chen, J. Zhu, W. Sun, W. Huang and S. X. Dou, Hybrid 2D Dual-Metal–Organic Frameworks for Enhanced Water Oxidation Catalysis, Adv. Funct. Mater., 2018, 28, 1–9 CrossRef.
- W. Xia, A. Mahmood, R. Zou and Q. Xu, Metal-organic frameworks and their derived nanostructures for electrochemical energy storage and conversion, Energy Environ. Sci., 2015, 8, 1837–1866 RSC.
- J. G. Park, M. L. Aubrey, J. Oktawiec, K. Chakarawet, L. E. Darago, F. Grandjean, G. J. Long and J. R. Long, Charge Delocalization and Bulk Electronic Conductivity in the Mixed-Valence Metal-Organic Framework Fe(1,2,3-triazolate)2(BF4)x, J. Am. Chem. Soc., 2018, 140, 8526–8534 CrossRef CAS PubMed.
- P. Silva, S. M. F. Vilela, J. P. C. Tomé and F. A. Almeida Paz, Multifunctional metal–organic frameworks: from academia to industrial applications, Chem. Soc. Rev., 2015, 44, 6774–6803 RSC.
- D. Feng, T. Lei, M. R. Lukatskaya, J. Park, Z. Huang, M. Lee, L. Shaw, S. Chen, A. A. Yakovenko, A. Kulkarni, J. Xiao, K. Fredrickson, J. B. Tok, X. Zou, Y. Cui and Z. Bao, Robust and conductive two-dimensional metal-organic frameworks with exceptionally high volumetric and areal capacitance, Nat. Energy, 2018, 3, 30–36 CrossRef CAS.
- L. S. Xie, L. Sun, R. Wan, S. S. Park, J. A. DeGayner, C. H. Hendon, M. Dincǎ, M. Dincă, J. A. DeGayner, C. H. Hendon, R. Wan, S. S. Park and L. S. Xie, Tunable Mixed-Valence Doping toward Record Electrical Conductivity in a Three-Dimensional Metal-Organic Framework, J. Am. Chem. Soc., 2018, 140, 7411–7414 CrossRef CAS PubMed.
- A. J. Clough, J. M. Skelton, C. A. Downes, A. A. De La Rosa, J. W. Yoo, A. Walsh, B. C. Melot and S. C. Marinescu, Metallic Conductivity in a Two-Dimensional Cobalt Dithiolene Metal-Organic Framework, J. Am. Chem. Soc., 2017, 139, 10863–10867 CrossRef CAS PubMed.
- M. A. Blood-Forsythe, C. R. Wade, A. Aspuru-Guzik, M. Dincă, C. K. Brozek, D. Sheberla, L. Sun and S. Er, High Electrical Conductivity in Ni3(2,3,6,7,10,11-hexaiminotriphenylene)2, a Semiconducting Metal–Organic Graphene Analogue, J. Am. Chem. Soc., 2014, 136, 8859–8862 CrossRef PubMed.
- S. Chandra, D. Roy Chowdhury, M. Addicoat, T. Heine, A. Paul and R. Banerjee, Molecular Level Control of the Capacitance of Two-Dimensional Covalent Organic Frameworks: Role of Hydrogen Bonding in Energy Storage Materials, Chem. Mater., 2017, 29, 2074–2080 CrossRef CAS.
- R. Dong, P. Han, H. Arora, M. Ballabio, M. Karakus, Z. Zhang, C. Shekhar, P. Adler, P. S. Petkov, A. Erbe, S. C. B. Mannsfeld, C. Felser, T. Heine, M. Bonn, X. Feng and E. Cánovas, High-mobility band-like charge transport in a semiconducting two-dimensional metal–organic framework, Nat. Mater., 2018, 17, 1027–1032 CrossRef CAS.
- G. Wu, J. Huang, Y. Zang, J. He and G. Xu, Porous field-effect transistors based on a semiconductive metal-organic framework, J. Am. Chem. Soc., 2017, 139, 1360–1363 CrossRef CAS.
- C. R. Deblase, K. Hernández-Burgos, K. E. Silberstein, G. G. Rodríguez-Calero, R. P. Bisbey, H. D. Abruña and W. R. Dichtel, Rapid and efficient redox processes within 2D covalent organic framework thin films, ACS Nano, 2015, 9, 3178–3183 CrossRef CAS.
- D. Sheberla, J. C. Bachman, J. S. Elias, C. J. Sun, Y. Shao-Horn and M. Dincă, Conductive MOF electrodes for stable supercapacitors with high areal capacitance, Nat. Mater., 2017, 16, 220–224 CrossRef CAS.
- H. Chen, H. Tu, C. Hu, Y. Liu, D. Dong, Y. Sun, Y. Dai, S. Wang, H. Qian, Z. Lin and L. Chen, Cationic Covalent Organic Framework Nanosheets for Fast Li-Ion Conduction, J. Am. Chem. Soc., 2018, 140, 896–899 CrossRef CAS.
- S. Wang, Q. Wang, P. Shao, Y. Han, X. Gao, L. Ma, S. Yuan, X. Ma, J. Zhou, X. Feng and B. Wang, Exfoliation of Covalent Organic Frameworks into Few-Layer Redox-Active Nanosheets as Cathode Materials for Lithium-Ion Batteries, J. Am. Chem. Soc., 2017, 139, 4258–4261 CrossRef CAS PubMed.
- C. R. Mulzer, L. Shen, R. P. Bisbey, J. R. McKone, N. Zhang, H. D. Abruña and W. R. Dichtel, Superior charge storage and power density of a conducting polymer-modified covalent organic framework, ACS Cent. Sci., 2016, 2, 667–673 CrossRef CAS PubMed.
-
S. Rana, R. Rajendra, B. Dhara, P. K. Jha and N. Ballav, Highly Hydrophobic and Chemically Rectifiable Surface-Anchored Metal-Organic Framework Thin-Film Devices, 2016, 3, 1–8.
- L. J. Han, D. Zheng, S. G. Chen, H. G. Zheng and J. Ma, A Highly Solvent-Stable Metal–Organic Framework Nanosheet: Morphology Control, Exfoliation, and Luminescent Property, Small, 2018, 14, 1–7 Search PubMed.
- H. Xu, J. Gao, X. Qian, J. Wang, H. He, Y. Cui, Y. Yang, Z. Wang and G. Qian, Metal-organic framework nanosheets for fast-response and highly sensitive luminescent sensing of Fe3+, J. Mater. Chem. A, 2016, 4, 10900–10905 RSC.
- M. G. Campbell and M. Dincă, Metal–organic frameworks as active materials in electronic sensor devices, Sensors, 2017, 17, 1–11 CrossRef PubMed.
- M. K. Smith and K. A. Mirica, Self-Organized Frameworks on Textiles (SOFT): Conductive Fabrics for Simultaneous Sensing, Capture, and Filtration of Gases, J. Am. Chem. Soc., 2017, 139, 16759–16767 CrossRef CAS PubMed.
- M. K. Smith, K. E. Jensen, P. A. Pivak and K. A. Mirica, Direct Self-Assembly of Conductive Nanorods of Metal-Organic Frameworks into Chemiresistive Devices on Shrinkable Polymer Films, Chem. Mater., 2016, 28, 5264–5268 CrossRef CAS.
- Z. Meng, A. Aykanat and K. A. Mirica, Welding Metallophthalocyanines into Bimetallic Molecular Meshes for Ultrasensitive, Low-Power Chemiresistive Detection of Gases, J. Am. Chem. Soc., 2018, 141, 2046–2053 CrossRef.
- A. M. Ullman, C. G. Jones, F. P. Doty, V. Stavila, A. A. Talin and M. D. Allendorf, Hybrid Polymer/Metal–Organic Framework Films for Colorimetric Water Sensing over a Wide Concentration Range, ACS Appl. Mater. Interfaces, 2018, 10, 24201–24208 CrossRef CAS.
- M. R. Tchalala, Y. Belmabkhout, K. Adil, K. N. Chappanda, A. Cadiau, P. M. Bhatt, K. N. Salama and M. Eddaoudi, Concurrent Sensing of CO2 and H2O from Air Using Ultramicroporous Fluorinated Metal-Organic Frameworks: Effect of Transduction Mechanism on the Sensing Performance, ACS Appl. Mater. Interfaces, 2019, 11, 1706–1712 CrossRef CAS.
- M. G. Campbell, D. Sheberla, S. F. Liu, T. M. Swager and M. Dincă, Cu3(hexaiminotriphenylene)2: an electrically conductive 2D metal-organic framework for chemiresistive sensing, Angew. Chem., Int. Ed., 2015, 54, 4349–4352 CrossRef CAS PubMed.
- Z. Li, L. Qiu, W. Wang, T. Xu, Y. Wu and X. Jiang, Fabrication of nanosheets of a fluorescent metal–organic framework [Zn(BDC)(H2O)]n (BDC = 1,4-benzenedicarboxylate): ultrasonic synthesis and sensing of ethylamine, Inorg. Chem. Commun., 2008, 11, 1375–1377 CrossRef CAS.
-
C. Zhang, S. Zhang, Y. Yan, F. Xia, A. Huang and Y. Xian, Highly Fluorescent Polyimide Covalent Organic Nanosheets as Sensing Probes for the Detection of 2,4,6-Trinitrophenol, 2017, 9, 13415–13421.
- Y. Peng, Y. Huang, Y. Zhu, B. Chen, L. Wang, Z. Lai, Z. Zhang, M. Zhao, C. Tan, N. Yang, F. Shao, Y. Han and H. Zhang, Ultrathin Two-Dimensional Covalent Organic Framework Nanosheets: Preparation and Application in Highly Sensitive and Selective DNA Detection, J. Am. Chem. Soc., 2017, 139, 8698–8704 CrossRef CAS PubMed.
- C. He, K. Lu and W. Lin, Nanoscale metal-organic frameworks for real-time intracellular pH sensing in live cells, J. Am. Chem. Soc., 2014, 136, 12253–12256 CrossRef CAS PubMed.
- R. Xu, Y. Wang, X. Duan, K. Lu, D. Micheroni, A. Hu and W. Lin, Nanoscale Metal-Organic Frameworks for Ratiometric Oxygen Sensing in Live Cells, J. Am. Chem. Soc., 2016, 138, 2158–2161 CrossRef CAS PubMed.
- Y. Wang, M. Zhao, J. Ping, B. Chen, X. Cao, Y. Huang, C. Tan, Q. Ma, S. Wu, Y. Yu, Q. Lu, J. Chen, W. Zhao, Y. Ying and H. Zhang, Bioinspired Design of Ultrathin 2D Bimetallic Metal-Organic-Framework Nanosheets Used as Biomimetic Enzymes, Adv. Mater., 2016, 28, 4149–4155 CrossRef CAS PubMed.
- J. Zhu, J. Hou, A. Uliana, Y. Zhang, M. Tian and B. Van Der Bruggen, The rapid emergence of two-dimensional nanomaterials for high-performance separation membranes, J. Mater. Chem. A, 2018, 6, 3773–3792 RSC.
- Z. Y. Yeo, S. P. Chai, P. W. Zhu and A. R. Mohamed, An overview: synthesis of thin films/membranes of metal organic frameworks and its gas separation performances, RSC Adv., 2014, 4, 54322–54334 RSC.
- D. J. Babu, G. He, L. F. Villalobos and K. V. Agrawal, Crystal Engineering of Metal-Organic Framework Thin Films for Gas Separations, ACS Sustainable Chem. Eng., 2019, 7, 49–69 CrossRef CAS.
- X. Li, Y. Liu, J. Wang, J. Gascon, J. Li and B. Van Der Bruggen, Metal-organic frameworks based membranes for liquid separation, Chem. Soc. Rev., 2017, 46, 7124–7144 RSC.
- H. Yang, L. Yang, H. Wang, Z. Xu, Y. Zhao, Y. Luo, N. Nasir, Y. Song, H. Wu, F. Pan and Z. Jiang, Covalent organic framework membranes through a mixed-dimensional assembly for molecular separations, Nat. Commun., 2019, 10, 1–10 CrossRef PubMed.
- M. Wang, F. Pan, H. Yang, Y. Cao, H. Wang, Y. Song, Z. Lu, M. Sun, H. Wu and Z. Jiang, Constructing channel-mediated facilitated transport membranes by incorporating covalent organic framework nanosheets with tunable microenvironments, J. Mater. Chem. A, 2019, 7, 9912–9923 RSC.
- P. Albacete, A. López-Moreno, S. Mena-Hernando, A. E. Platero-Prats, E. M. Pérez and F. Zamora, Chemical sensing of water contaminants by a colloid of a fluorescent imine-linked covalent organic framework, Chem. Commun., 2019, 55, 1382–1385 RSC.
- Z. G. Gu, W. Q. Fu, X. Wu and J. Zhang, Liquid-phase epitaxial growth of a homochiral MOF thin film on poly(l-DOPA) functionalized substrate for improved enantiomer separation, Chem. Commun., 2016, 52, 772–775 RSC.
- J. Guo, Y. Zhang, Y. Zhu, C. Long, M. Zhao, M. He, X. Zhang, J. Lv, B. Han and Z. Tang, Chiral MOFs Very Important Paper Ultrathin Chiral Metal–Organic-Framework Nanosheets for Efficient Enantioselective Separation, Angew. Chem., Int. Ed., 2018, 6873–6877, DOI:10.1002/anie.201803125.
- X. Wu, X. Han, Q. Xu, Y. Liu, C. Yuan, S. Yang, Y. Liu, J. Jiang and Y. Cui, Chiral BINOL-Based Covalent Organic Frameworks for Enantioselective Sensing, J. Am. Chem. Soc., 2019, 141, 7081–7089 CrossRef CAS PubMed.
- H. Ang and L. Hong, Polycationic Polymer-Regulated Assembling of 2D MOF Nanosheets for High-Performance Nanofiltration, ACS Appl. Mater. Interfaces, 2017, 9, 28079–28088 CrossRef CAS PubMed.
-
S. Kandambeth, B. P. Biswal, H. D. Chaudhari, K. C. Rout, U. K. Kharul and R. Banerjee, Selective Molecular Sieving in Self-Standing Porous Covalent-Organic-Framework Membranes, 2017, 29, 1–9.
- K. Dey, M. Pal, K. C. Rout, S. S. Kunjattu, A. Das, R. Mukherjee, U. K. Kharul and R. Banerjee, Selective Molecular Separation by Interfacially Crystallized Covalent Organic Framework Thin Films, J. Am. Chem. Soc., 2017, 139, 13083–13091 CrossRef CAS PubMed.
- D. Cohen-Tanugi and J. C. Grossman, Water desalination across nanoporous graphene, Nano Lett., 2012, 12, 3602–3608 CrossRef CAS PubMed.
- L. C. Lin, J. Choi and J. C. Grossman, Two-dimensional covalent triazine framework as an ultrathin-film nanoporous membrane for desalination, Chem. Commun., 2015, 51, 14921–14924 RSC.
- W. Zhou, M. Wei, X. Zhang, F. Xu and Y. Wang, Fast Desalination by Multilayered Covalent Organic Framework (COF) Nanosheets, ACS Appl. Mater. Interfaces, 2019, 11, 16847–16854 CrossRef CAS PubMed.
- X. Liu, N. K. Demir, Z. Wu and K. Li, Highly Water-Stable Zirconium Metal-Organic Framework UiO-66 Membranes Supported on Alumina Hollow Fibers for Desalination, J. Am. Chem. Soc., 2015, 137, 6999–7002 CrossRef CAS PubMed.
- X. Wang, L. Zhai, Y. Wang, R. Li, X. Gu, Y. D. Yuan, Y. Qian, Z. Hu and D. Zhao, Improving Water-Treatment Performance of Zirconium Metal-Organic Framework Membranes by Postsynthetic Defect Healing, ACS Appl. Mater. Interfaces, 2017, 9, 37848–37855 CrossRef CAS PubMed.
- X. Wang, C. Chi, K. Zhang, Y. Qian, K. M. Gupta, Z. Kang, J. Jiang and D. Zhao, Reversed thermo-switchable molecular sieving membranes composed of two-dimensional metal-organic nanosheets for gas separation, Nat. Commun., 2017, 8, 1–10 CrossRef PubMed.
- P. Szilágyi, R. J. Westerwaal, M. Lansink, H. I. Van Montfort, B. J. Trze
niewski, M. V. Garcia, H. Geerlings and B. Dam, Contaminant-resistant MOF-Pd composite for H2 separation, RSC Adv., 2015, 5, 89323–89326 RSC.
- Z. P. Smith, J. E. Bachman, T. Li, B. Gludovatz, V. A. Kusuma, T. Xu, D. P. Hopkinson, R. O. Ritchie and J. R. Long, Increasing M2(dobdc) Loading in Selective Mixed-Matrix Membranes: A Rubber Toughening Approach, Chem. Mater., 2018, 30, 1484–1495 CrossRef CAS.
- Z. Kang, Y. Peng, Y. Qian, D. Yuan, M. A. Addicoat, T. Heine, Z. Hu, L. Tee, Z. Guo and D. Zhao, Mixed Matrix Membranes (MMMs) Comprising Exfoliated 2D Covalent Organic Frameworks (COFs) for Efficient CO2 Separation, Chem. Mater., 2016, 28, 1277–1285 CrossRef CAS.
- K. Duan, J. Wang, Y. Zhang and J. Liu, Covalent organic frameworks (COFs) functionalized mixed matrix membrane for effective CO2/N2 separation, J. Membr. Sci., 2019, 572, 588–595 CrossRef CAS.
- P. Chandrasekhar, A. Mukhopadhyay, G. Savitha and J. N. Moorthy, Orthogonal self-assembly of a trigonal triptycene triacid: signaling of exfoliation of porous 2D metal-organic layers by fluorescence and selective CO2 capture by the hydrogen-bonded MOF, J. Mater. Chem. A, 2017, 5, 5402–5412 RSC.
- M. H. Pham, G. T. Vuong, F. G. Fontaine and T. O. Do, Rational synthesis of metal-organic framework nanocubes and nanosheets using selective modulators and their morphology-dependent gas-sorption properties, Cryst. Growth Des., 2012, 12, 3091–3095 CrossRef CAS.
- Y. Lo, C. H. Lam, C. W. Chang, A. C. Yang and D. Y. Kang, Polymorphism/pseudopolymorphism of metal-organic frameworks composed of zinc(II) and 2-methylimidazole: synthesis, stability, and application in gas storage, RSC Adv., 2016, 6, 89148–89156 RSC.
- D. Kim, D. W. Kim, W. G. Hong and A. Coskun, Graphene/ZIF-8 composites with tunable hierarchical porosity and electrical conductivity, J. Mater. Chem. A, 2016, 4, 7710–7717 RSC.
- R. Kumar, K. Jayaramulu, T. K. Maji and C. N. R. Rao, Growth of 2D sheets of a MOF on graphene surfaces to yield composites with novel gas adsorption characteristics, Dalton Trans., 2014, 43, 7383–7386 RSC.
- H. Li, J. Hou, T. D. Bennett, J. Liu and Y. Zhang, Templated growth of vertically aligned 2D metal-organic framework nanosheets, J. Mater. Chem. A, 2019, 7, 5811–5818 RSC.
- P. Á. Szilágyi, R. J. Westerwaal, R. Van De Krol, H. Geerlings and B. Dam, Metal-organic framework thin films for protective coating of Pd-based optical hydrogen sensors, J. Mater. Chem. C, 2013, 1, 8146–8155 RSC.
- K. Liang, J. J. Richardson, C. J. Doonan, X. Mulet, Y. Ju, J. Cui, F. Caruso and P. Falcaro, An Enzyme-Coated Metal–Organic Framework Shell for Synthetically Adaptive Cell Survival, Angew. Chem., Int. Ed., 2017, 56, 8510–8515 CrossRef CAS.
- M. Hanke, H. K. Arslan, S. Bauer, O. Zybaylo, C. Christophis, H. Gliemann, A. Rosenhahn and C. Wöll, The biocompatibility of metal-organic framework coatings: an investigation on the stability of SURMOFs with regard to water and selected cell culture media, Langmuir, 2012, 28, 6877–6884 CrossRef CAS PubMed.
-
M. Tsotsalas, J. Liu, B. Tettmann, S. Grosjean, A. Shahnas, Z. Wang, C. Azucena, M. Addicoat, T. Heine, J. Lahann, J. Overhage, S. Bra, H. Gliemann and C. Wo, Fabrication of Highly Uniform Gel Coatings by the Conversion of Surface-Anchored Metal–Organic Frameworks, 2014, 136, 8–11.
- C. T. Buru, M. B. Majewski, A. J. Howarth, R. H. Lavroff, C. W. Kung, A. W. Peters, S. Goswami and O. K. Farha, Improving the Efficiency of Mustard Gas Simulant Detoxification by Tuning the Singlet Oxygen Quantum Yield in Metal-Organic Frameworks and Their Corresponding Thin Films, ACS Appl. Mater. Interfaces, 2018, 10, 23802–23806 CrossRef CAS PubMed.
- P. De Luna, W. Liang, A. Mallick, O. Shekhah, F. P. García De Arquer, A. H. Proppe, P. Todorović, S. O. Kelley, E. H. Sargent and M. Eddaoudi, Metal-Organic Framework Thin Films on High-Curvature Nanostructures Toward Tandem Electrocatalysis, ACS Appl. Mater. Interfaces, 2018, 10, 31225–31232 CrossRef CAS PubMed.
|
This journal is © The Royal Society of Chemistry 2019 |
Click here to see how this site uses Cookies. View our privacy policy here.