DOI:
10.1039/D3NA00187C
(Paper)
Nanoscale Adv., 2023,
5, 2921-2932
Surface-modified Ti3C2 MXene nanosheets for mesenchymal stem cell osteogenic differentiation via photothermal conversion
Received
24th March 2023
, Accepted 21st April 2023
First published on 9th May 2023
Abstract
In the field of bone tissue engineering, the practical application of growth factors is limited by various factors such as systemic toxicity, instability, and the potential to induce inflammation. To circumvent these limitations, the use of physical signals, such as thermal stimulation, to regulate stem cells has been proposed as a promising alternative. The present study aims to investigate the potential of the two-dimensional nanomaterial Ti3C2 MXene, which exhibits unique photothermal properties, to induce osteogenic differentiation of bone marrow-derived mesenchymal stem cells (BMSCs) via photothermal conversion. Surface modification of Ti3C2 MXene nanosheets with PVP (Ti3C2–PVP) was employed to enhance their colloidal stability in physiological solutions. Characterization and cellular experiments showed that Ti3C2–PVP nanosheets have favorable photothermal properties and biocompatibility. Our study demonstrated that the induction of photothermal stimulation by co-culturing Ti3C2–PVP nanosheets with BMSCs and subsequent irradiation with 808 nm NIR significantly promoted cell proliferation, adhesion and osteogenic differentiation of BMSCs. In conclusion, the results of this study suggest that Ti3C2–PVP is a promising material for bone tissue engineering applications as it can modulate the cellular functions of BMSCs through photothermal conversion.
Introduction
Bone tissue engineering is a promising approach for treating severe bone defects because it can reduce the complications associated with autograft use, particularly those related to donor site healing.1,2 Bone tissue engineering involves three key elements: seed cells, scaffolds, and growth factors.3In vitro cell culture on tissue engineering materials is an option, but the high cost and complex regulatory requirements of biological components have led to a preference for the recruitment of the patient's own cells from surrounding tissues.4 Bone marrow-derived mesenchymal stem cells (BMSCs) are critical for the generation of cells such as osteoblasts, which play a critical role in maintaining bone tissue homeostasis and repairing bone defects.5 In bone tissue engineering, the use of artificial materials requires the supplementation of growth factors such as FGF, VEGF, IGF, TGF-β, PDGF, and BMP to stimulate the differentiation of BMSCs into osteoblasts.6,7 Although growth factors play a crucial role in bone tissue engineering, their practical application is limited by issues such as systemic toxicity, instability, and the potential to induce inflammation.8
The application of physical signals to regulate stem cell fate has become an area of increasing interest in the fields of biomaterials, tissue engineering, and cell biology.9 Physical signals offer an alternative to the use of growth factors in bone tissue engineering, by providing a safe, targeted, and adjustable method.10 There are six categories of physical signals that can be sensed by cells, including mechanical, optical, thermal, electrical, acoustic, and magnetic.10 Thermal stimulation, as a physical signal, is known to have both positive and negative effects on biological systems. While elevated temperatures above 46 °C have been shown to cause cell death and tissue damage,11 mild thermal stimulation at temperatures between 40 and 42 °C, which is only 3–5 °C above the normal body temperature, has been shown to regulate stem cell differentiation and facilitate bone regeneration.10,12–14
Traditional direct thermal treatments, such as hot compresses, have limitations in that they primarily affect superficial areas and lack precise control over temperature and duration. To further exploit the osteogenic effects of mild thermal stimulation and overcome these limitations, researchers have employed methods to deliver heat to deep body tissues using magnetic fields, infrared radiation, and X-rays.15 Photothermal therapy (PTT) has gained prominence in biomedicine due to its capacity to selectively convert near-infrared (NIR) light, which exhibits good transmissivity, into heat through photothermal conversion. This addresses the challenge of delivering thermal stimulation efficiently to deep, specific lesion sites within the human body.16 PTT is primarily utilized for cancer treatment and antimicrobial therapy,17,18 offering advantages such as the absence of drug resistance and minimal side effects compared to conventional chemotherapy.19
Recent research has generated a growing interest in the utilization of two-dimensional (2D) nanomaterials, an emerging class of photothermal agents with unique physical and chemical properties, to deliver thermal stimulation in the field of bone tissue engineering.15,20,21 Ti3C2 MXene is a kind of 2D nanomaterials which holds great promise due to their biocompatibility, high absorption of NIR, and efficient photothermal conversion ability.22,23 Although some studies have suggested the potential of Ti3C2 MXene to promote osteogenesis in mesenchymal stem cells, the effect of photothermal modulation remains under-investigated.24 Our hypothesis is that Ti3C2 MXene could be an optimal photothermal agent to deliver thermal stimulation in order to induce osteogenic differentiation of BMSCs and potentially reduce the reliance on growth factors.
The aim of this study is to investigate the photothermal modulation of BMSCs by Ti3C2 MXene nanosheets under NIR irradiation. To overcome the limitations of instability and oxidation susceptibility of Ti3C2 MXene,25,26 poly(N-vinylpyrrolidone) (PVP) was used to modify the nanosheets. PVP is an amphiphilic, water-soluble polymer. Demonstrated as non-irritating to skin, eyes, and mucosa, PVP exhibits excellent tissue compatibility.28 Owing to its remarkable solubility in various conventional solvents, low toxicity and chemical stability, the use of PVP for surface modification has been extensively explored in the field of pharmaceutical manufacturing and biomaterial development.27 The surface-modified Ti3C2 (Ti3C2–PVP) nanosheets showed improved stability in physiological solutions, excellent biocompatibility, and enhanced osteogenic activity in cellular experiments. Furthermore, thermal stimulation generated by NIR light was found to modulate the proliferation, adhesion, and osteogenic differentiation of BMSCs. These results are expected to provide a deeper insight into the photothermal modulation of BMSCs by Ti3C2 MXene nanosheets under NIR irradiation and its potential applications in bone tissue engineering.
Experimental
Synthesis of Ti3C2 MXene nanosheets
The preparation of Ti3C2 MXene was performed according to the MILD method.29 First, 0.8 g of LiF was added to 10 mL of 9 M HCl to prepare the etching solution. Next, the Ti3AlC2 MAX powder was slowly added to the etching solution and stirred at a constant temperature of 35 °C for 48 hours. After the reaction, the resulting product was centrifuged and washed several times with deionized water, followed by further hand shaking and centrifugation to obtain a suspension of Ti3C2 MXene nanosheets.
Surface modification of Ti3C2 MXene nanosheets (Ti3C2–PVP)
The process of modifying Ti3C2 MXene nanosheets with PVP was carried out to improve the stability. A mixture of 25 mg of Ti3C2 MXene and 200 mg of PVP with an average molecular weight of 40
000 was dissolved in 100 mL of ethanol. This solution was then stirred overnight at 50 °C. The final product, Ti3C2–PVP, was obtained by centrifugation and multiple washes with water and ethanol. Ti3C2 and Ti3C2–PVP colloidal solutions were diluted to a concentration of 25 μg mL−1 using deionized water, PBS, and Dulbecco's modified Eagle's medium (DMEM). The solutions were placed in vials and allowed to stand undisturbed for one week. Digital photographs were taken to observe and evaluate the stability of Ti3C2 and Ti3C2–PVP.
Characterization
The morphology of the samples was studied by scanning electron microscopy (SEM, GEMINI 300, ZEISS, Germany) and transmission electron microscopy (TEM, Talos F200x, FEI, USA). The crystal structure was analyzed by X-ray diffraction (XRD, D8A25, Bruker, Germany). UV-vis-NIR absorption spectra were recorded using a UV-vis-NIR spectrometer (UV-3600i Plus, SHIMADZU, Japan). Particle size and zeta-potential were measured using dynamic light scattering (DLS) and zeta-potential measurements on a Zetasizer Nanoseries instrument (ZS90, Malvern, UK). The photothermal properties of the samples were evaluated under 808 nm NIR light (LASERWAVE, China) and the temperature was recorded using an Infrared Thermal Imager (InfiRay, China).
Cell culture
Mouse bone marrow-derived mesenchymal stem cells (BMSCs) were obtained from Jinyuan Technology (China) and cultured in RPMI-1640 medium (Gibco, USA) that was supplemented with 10% fetal bovine serum (FBS, Gibco, USA) and 1% penicillin/streptomycin (Invitrogen, USA). Cells were maintained in a controlled environment in an incubator at 5% CO2 and 95% relative humidity.
NIR irradiation of BMSCs
The BMSCs were co-cultured with 12.5 μg mL−1 of Ti3C2–PVP nanosheets. The control group consisted of growth medium only. The cells were exposed to exposure to an 808 nm near-infrared laser (LASERWAVE, China) until the temperature reached 42 °C and was maintained at this temperature for a duration of 5 minutes once a day.
Cell proliferation
To evaluate cell proliferation, the BMSCs were seeded and cultured overnight in 96-well plates at a density of 5000 cells per well. Ti3C2–PVP nanosheet colloidal solution was diluted with complete medium at concentrations of 50, 25, and 12.5 μg mL−1, while the control group consisted of pure complete medium. Cell viability was evaluated at 1, 3, 5, and 7 days using the Cell Counting Kit-8 (CCK-8, Dojindo, Japan). Absorbance values were measured using a microplate reader (BioTek, USA) at a wavelength of 450 nm. The relative cell proliferation rate was calculated as (experimental OD − control OD)/control OD.
Live/dead assay
The BMSCs were seeded at a density of 10
000 cells per well in 24-well plates. Twenty-four hours after NIR irradiation, the samples were rinsed three times with PBS, incubated with calcein-AM and propidium iodide (PI) for 30 minutes at 37 °C in the dark, and observed with a fluorescence microscope (Olympus, Japan).
Cell morphology
The BMSCs were cultured in 24-well plates on 14 mm diameter coverslips at a density of 10
000 cells per well. The cell morphology was observed by phase-contrast microscopy (Olympus, Japan) for bright field imaging. For SEM observation, cells were fixed in a 2.5% glutaraldehyde solution overnight at 4 °C. After thorough rinsing with PBS three times, the samples underwent a sequential ethanol dehydration process at increasing concentrations (50%, 75%, 85%, 95%, and 100%). To visualize the cytoskeleton, the samples were washed, fixed, permeabilized, blocked, and incubated with Alexa Fluor 488-phalloidin (Invitrogen, USA) for actin staining and DAPI (Invitrogen, USA) for nuclear staining. The samples were then observed under a fluorescence microscope (Olympus, Japan). Quantification was performed by calculating the area of cell spreading (represented in green) using ImageJ software.
Quantitative reverse transcription-PCR (qRT-PCR)
The BMSCs were cultured in 12-well plates and exposed to 12.5 μg mL−1 Ti3C2–PVP nanosheets and NIR light. Osteogenesis induction medium was prepared by supplementing the complete medium with 10 nM dexamethasone, 10 mM sodium β-glycerol phosphate disodium, and 50 μg mL−1 ascorbic acid. After osteogenesis induction for 14 days, total RNA was extracted using Cell Total RNA Isolation Kit (Vazyme, China). Reverse transcription was performed to generate cDNA using HiScript III All-in-one RT SuperMix (Vazyme, China). Then, a 20 μL quantitative real-time PCR system was established using the Taq Pro Universal SYBR qPCR Master Mix (Vazyme, China). Real-time fluorescence quantitative PCR analysis was performed using a real-time fluorescence quantitative PCR system (Bio-Rad, USA). Relative gene expression levels were determined by the ΔΔCt method and normalized by GAPDH. Primer sequences used in the analysis are listed below:
GAPDH: F-5′-AGGTCGGTGTGAACGGATTTG-3′, R-5′-TGTAGACCATGTAGTTGAGGTCA-3′;
ALP: F-5′-TCCGTGGGCATTGTGACTAC-3′, R-5′-TGGTGGCATCTCGTTATCCG-3′;
OCN: F-5′-GGTAGTGAACAGACTCCGGC-3′, R-5′-GGCGGTCTTCAAGCCATACT-3′;
OPN: F-5′-ATCTCACCATTCGGATGAGTCT-3′, R-5′-TGTAGGGACGATTGGAGTGAAA-3′;
RUNX2: F-5′-GACTGTGGTTACCGTCATGGC-3′, R-5′-ACTTGGTTTTTCATAACAGCGGA-3′;
COL1: F-5′-GCTCCTCTTAGGGGCCACT-3′, R-5′-ATTGGGGACCCTTAGGCCAT-3′.
ALP staining
The BMSCs were cultured in 12-well plates and exposed to 12.5 μg mL−1 Ti3C2–PVP nanosheets and NIR light. After 7 days of osteogenic induction, the samples were fixed in 4% paraformaldehyde for 30 minutes and rinsed with PBS. The BCIP/NBT ALP staining kit from Beyotime Biotechnology (China) was used to perform the staining procedure. The samples were incubated with the working staining solution for 24 hours at room temperature in the dark. The samples were then visualized by microscopy, and the quantification of ALP-positive areas was performed using ImageJ software. Quantification was performed by calculating the ratio of the stained area (represented in blue) to the total area (stained area/total area × 100%).
Alizarin red staining
For alizarin red staining (ARS) procedure, the BMSCs were induced under conditions similar to those employed for the ALP staining. After a period of 14 days of osteogenic induction, the cells were fixed with 4% paraformaldehyde for 30 minutes and then rinsed thoroughly with PBS without Ca2+ and Mg2+. Subsequently, the samples were incubated with 0.1% ARS solution for 30 minutes in the dark. Calcium deposits were quantified by determining the ratio of stained area (red) to total area using ImageJ software.
Statistical analysis
Data were presented as the mean ± standard deviation and analyzed using GraphPad Prism 9 software. The experiment was performed at least three times to ensure reproducibility of the results. Normality of all experimental data was verified by the Shapiro–Wilk test, and in the case that all data conformed to normal distribution, one-way analysis of variance (ANOVA) was used to evaluate the differences between groups. The Student–Newman–Keuls test was utilized to examine the differences between each pair of groups. Statistical significance was determined at a p-value of less than 0.05.
Results
Synthesis and characterization of Ti3C2–PVP nanosheets
As shown in Fig. 1A, the Ti3C2 MXene nanosheets were synthesized through a process involving the etching of the precursor Ti3AlC2 MAX. To enhance the stability of Ti3C2 nanosheets, surface modification was carried out utilizing PVP. The morphology of Ti3C2 nanosheets was characterized by SEM and TEM. As shown in Fig. 1B and C, the SEM and TEM images revealed that the exfoliated Ti3C2 nanosheets were well-layered and exhibited a typical two-dimensional layered structure with a lateral size of several hundred nanometers. To confirm the complete etching of the Al atomic layer, XRD was performed to analyze the crystal structure of the Ti3C2 MXene. The XRD pattern (Fig. 1D) showed that all the characteristic peaks of Ti3AlC2 had weakened or disappeared, except for the (002) peak which shifted to the lower angle, indicating the successful synthesis of Ti3C2 MXene and the complete etching of the Al atomic layer.
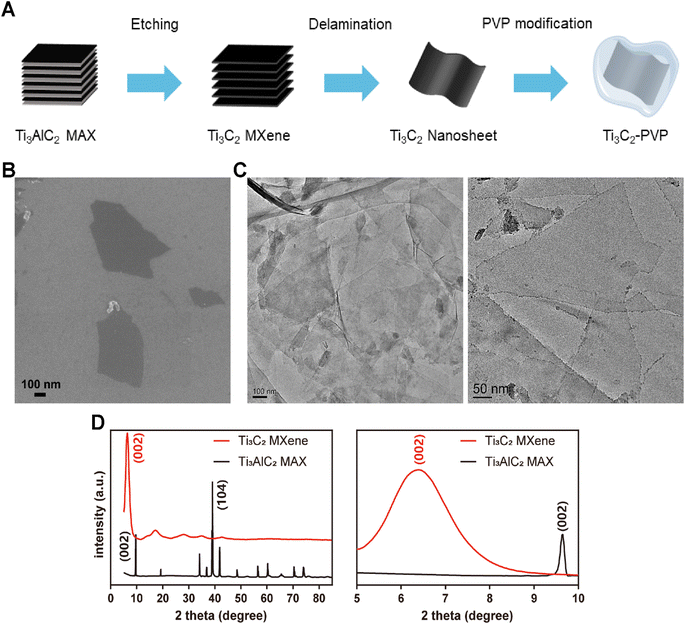 |
| Fig. 1 Characterization. (A) Schematic of the preparation of Ti3C2 MXene nanosheets and PVP modification. (B) SEM image of Ti3C2 nanosheets. (C) TEM images of Ti3C2 nanosheets. (D) XRD pattern of Ti3AlC2 MAX and Ti3C2 MXene. | |
Although Ti3C2 nanosheets exhibited good dispersion and stability in water, the poor stability in saline solution (PBS) and Dulbecco's modified Eagle medium (DMEM) resulted in significant agglomeration as illustrated in Fig. 2A. The modified Ti3C2 nanosheets (Ti3C2–PVP) exhibited improved dispersion and colloidal stability in a variety of simulated physiological media, including water, PBS, and DMEM. The UV-vis-NIR absorption spectra were analyzed to determine the effect of PVP modification on the optical properties of Ti3C2 nanosheets. The results, as shown in Fig. 2B, indicated that the PVP modification did not have a negative effect on the optical properties of Ti3C2 nanosheets, as evidenced by the absorption peak near 800 nm of Ti3C2–PVP. The absorption peak of Ti3C2–PVP was located in the NIR region, highlighting the potential of the nanosheet to absorb 808 nm NIR light.
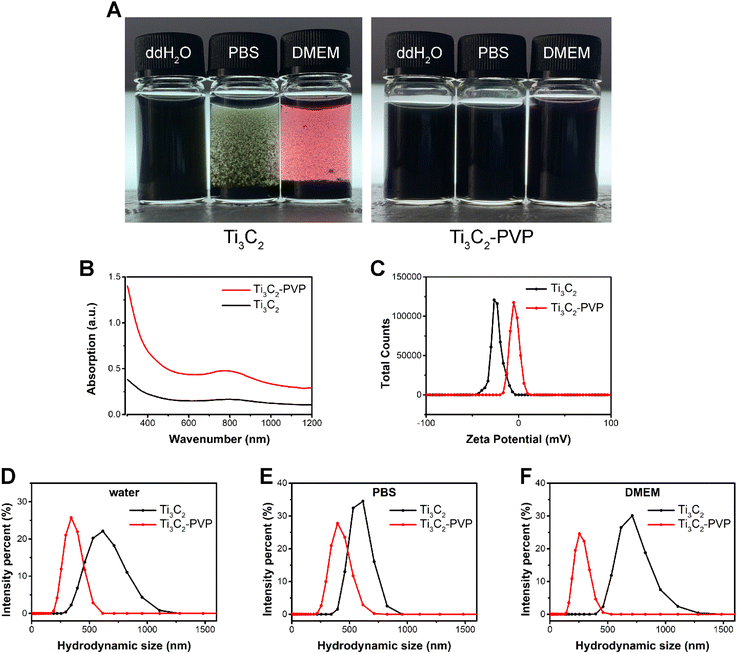 |
| Fig. 2 Stability of Ti3C2–PVP nanosheets. (A) Photographs of Ti3C2 and Ti3C2–PVP nanosheets in water, PBS, and media (DMEM) at least 1 week. (B) UV-vis-NIR absorption spectra of Ti3C2 and Ti3C2–PVP. (C) Zeta potential analysis of Ti3C2 and Ti3C2–PVP in water. (D–F) Hydrodynamic size of Ti3C2 and Ti3C2–PVP in water, PBS, and media (DMEM). | |
In order to determine the effectiveness of the surface modification of Ti3C2–PVP nanosheets, zeta potential analysis was performed. The results (Fig. 2C) showed that the Ti3C2 nanosheets were stable in deionized water and had a zeta potential of −23.33 ± 0.81 mV. After modification with PVP, the zeta potential decreased to −4.717 ± 0.63 mV, indicating that the macromolecular chains of PVP form a layer of spatial resistance on the surface of the Ti3C2 nanosheets. To further evaluate the colloidal stability of Ti3C2–PVP nanosheets in physiological solutions, dynamic light scattering (DLS) was performed to determine the hydrodynamic size distribution. The results (Fig. 2D–F) showed that the hydrodynamic size of Ti3C2 in water, PBS, and DMEM was 611.0 ± 2.718 nm, 554.2 ± 27.53 nm, and 695.2 ± 13.69 nm, respectively. In contrast, Ti3C2–PVP in water, PBS, and DMEM showed smaller nanosheet sizes, which were 351.6 ± 6.313 nm, 412.9 ± 6.350 nm, and 266.7 ± 2.483 nm, respectively.
Photothermal property of Ti3C2–PVP nanosheets
The photothermal heating ability was investigated by measuring the temperature of Ti3C2 and Ti3C2–PVP nanosheets of different concentrations (100, 50, 25, and 12.5 μg mL−1) after exposure to 808 nm NIR laser at a power density of 1.0 W cm−2. As depicted in Fig. 3A and B, the results showed that both Ti3C2 and Ti3C2–PVP colloidal solution achieved a temperature increase to 65 °C (100 μg mL−1) and 40 °C (12.5 μg mL−1), respectively, within 3 minutes of NIR laser exposure. At concentrations of 50 μg mL−1 and 25 μg mL−1, the temperature of Ti3C2–PVP nanosheets decreased slightly to 57.5 and 47.1 °C, respectively, compared to 63.7 and 50.5 °C of Ti3C2 nanosheets.
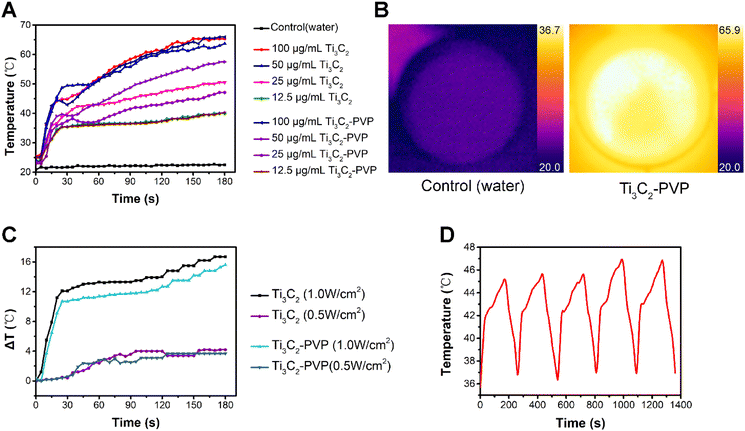 |
| Fig. 3 Photothermal properties of Ti3C2–PVP nanosheets. (A) Photothermal heating curves of Ti3C2 and Ti3C2–PVP colloidal solution with different concentrations (100, 50, 25, 12.5 μg mL−1) under 808 nm NIR irradiation (1.0 W cm−2). (B) Infrared thermal images of water and Ti3C2–PVP colloidal solutions (100 μg mL−1) under 808 nm laser irradiation (1.0 W cm−2). (C) Temperature change curves of Ti3C2–PVP colloidal solutions (12.5 μg mL−1) under 808 nm NIR irradiation at different power densities (0.5, 1.0 W cm−2). (D) Recycling heating curves of Ti3C2–PVP colloidal solutions (12.5 μg mL−1) for five ON/OFF cycles under 808 nm NIR laser (1.0 W cm−2). | |
Furthermore, the photothermal performance of Ti3C2–PVP nanosheets (12.5 μg mL−1) was evaluated at different NIR power densities. The results, shown in Fig. 3C, indicated that after 3 minutes of exposure to NIR light at a power density of 1.0 W cm−2, the temperature of Ti3C2–PVP increased by 15.6 °C, which was comparable to the temperature increase of 16.7 °C for Ti3C2. In addition, at a power density of 0.5 W cm−2, the temperature of Ti3C2–PVP increased by 3.7 °C, while the temperature of Ti3C2 increased by 4.2 °C.
To evaluate the photothermal stability of Ti3C2–PVP nanosheets, we evaluated the temperature change during five consecutive ON/OFF cycles of the NIR laser. The results (Fig. 3D) indicated that Ti3C2–PVP nanosheets colloidal solution showed no decrease in temperature during the ON/OFF cycles, indicating their good photothermal stability and suitability for repeated and effective use in photothermal treatment processes.
Biocompatibility analysis
In order to evaluate the biocompatibility of Ti3C2–PVP nanosheets, the effect on the cell proliferation ability of BMSCs was analyzed using the CCK-8 assay. The BMSCs were co-cultured with Ti3C2–PVP nanosheets for 1, 3, 5, and 7 days, and the results (Fig. 4A) showed that Ti3C2–PVP exhibited good biocompatibility at low concentrations (12.5 and 25 μg mL−1). However, at a concentration of 50 μg mL−1, a significant inhibition of cell proliferation was observed at 1 day. Notably, at a concentration of 12.5 μg mL−1, Ti3C2–PVP nanosheets significantly promoted cell proliferation at 5 days. Considering the biocompatibility and photothermal properties, the concentration of 12.5 μg mL−1 of Ti3C2–PVP nanosheets colloidal solution was selected for subsequent experiments.
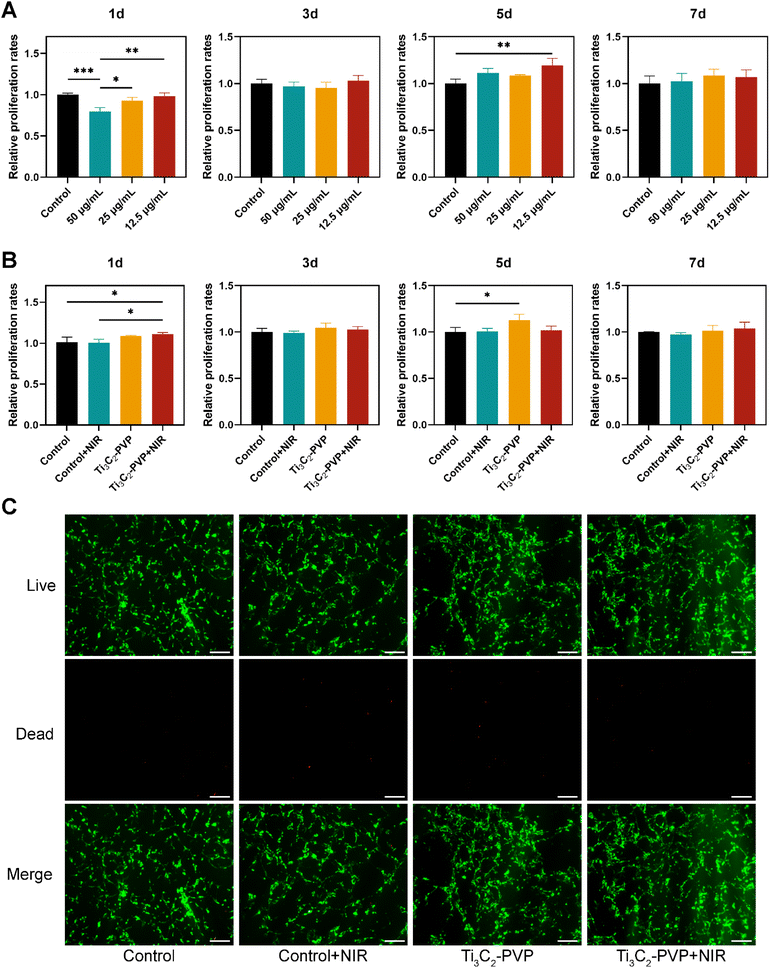 |
| Fig. 4 Biocompatibility assessments. (A) Relative proliferation rates of BMSCs cells co-cultured with Ti3C2–PVP nanosheets at concentrations of 50, 25, 12.5, 0 μg mL−1 for 1, 3, 5 and 7 days. (n = 3, *p < 0.05, **p < 0.01, ***p < 0.001). (B) Relative proliferation rates of BMSCs co-cultured with growth medium and Ti3C2–PVP nanosheets at concentrations of 12.5 μg mL−1 with or without NIR irradiation for 1, 3, 5 and 7 days (*p < 0.05). (C) Live/dead double staining, cells co-cultured with Ti3C2–PVP nanosheets with or without NIR irradiation for 24 h. Red staining represents dead cells while green fluorescence indicates live cells. Scale bars: 200 μm. | |
The effect of the photothermal effect of Ti3C2–PVP nanosheets on cell viability and proliferation was further evaluated by CCK-8 and live/dead staining. The CCK-8 results, as shown in Fig. 4B, indicated that cell proliferation was significantly enhanced at 1 day in the Ti3C2–PVP + NIR group, but no significant difference was observed at 3, 5, and 7 days, when compared with the other groups. Live/dead staining, as shown in Fig. 4C, revealed that all groups showed strong green fluorescence, indicating that most of the cells were viable and only a small number of cells were dead (red fluorescence).
Cellular interactions of Ti3C2–PVP nanosheets with BMSCs
Bright field imaging and SEM were used to determine the interaction of Ti3C2–PVP nanosheets with BMSCs. As illustrated in Fig. 5A, after co-culture with Ti3C2–PVP for 24 hours, the cells appeared to be heavily enriched with nanosheets, as indicated by the black sheet-like material present on the cell surface.
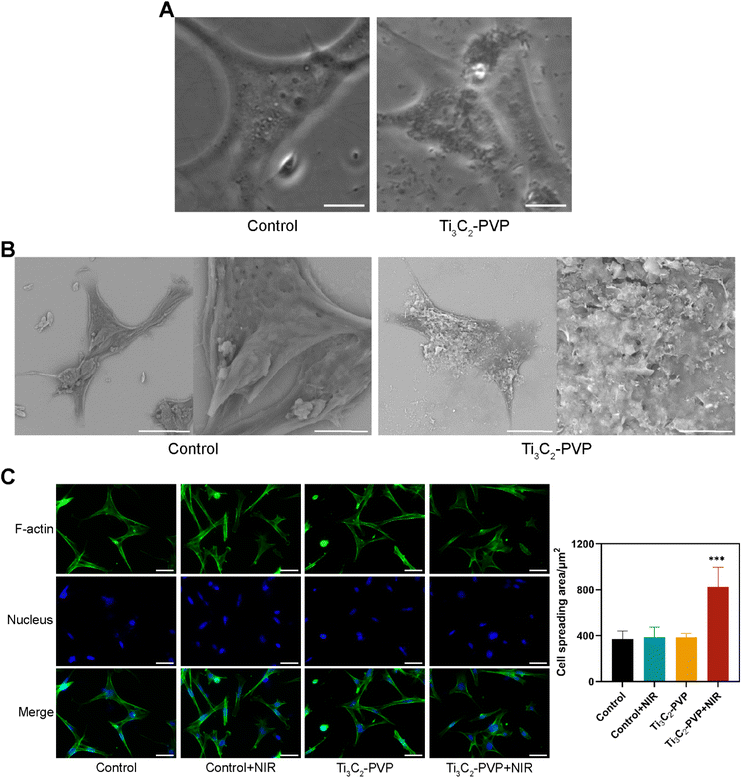 |
| Fig. 5 Cellular interactions of Ti3C2–PVP nanosheets with BMSCs. (A) Bright field imaging of BMSCs co-cultured with Ti3C2–PVP. Scale bars: 10 μm. (B) SEM images of BMSCs co-cultured with Ti3C2–PVP. Scale bars: 30 μm (left), 8 μm (right). (C) Cytoskeleton immunofluorescence image of BMSCs co-cultured with Ti3C2–PVP with or without NIR irradiation and quantitative data of cell spreading area (n = 3, ***p < 0.001, comparison between control and other groups). Scale bars: 50 μm. | |
The SEM results (Fig. 5B) confirmed that Ti3C2–PVP nanosheets tended to bind to the cell membrane, showing significant enrichment compared to the cell culture substrate. The effect of photothermal treatment on the morphology of BMSCs was also examined by staining for cytoskeletal actin. The results, as shown in Fig. 5C, showed that BMSCs in the Ti3C2–PVP + NIR group exhibited a flatter shape and larger spreading area compared to the other groups, suggesting that photothermal treatment promoted the adhesion of BMSCs.
Photothermal osteogenic effect of Ti3C2–PVP nanosheets on BMSCs
To evaluate the photothermal modulation of Ti3C2–PVP on the osteogenic differentiation of BMSCs, the relative mRNA expression levels of osteogenic markers, including ALP, OCN, OPN, RUNX2, and COL1, were evaluated by qRT-PCR after 14 days of osteogenic induction. The results (Fig. 6A–E) showed that the expression levels of ALP, OCN, OPN, RUNX2, and COL1 were significantly increased in the Ti3C2–PVP + NIR group compared with the other groups. Conversely, NIR irradiation alone without Ti3C2–PVP did not affect gene expression. In addition, the expression levels of ALP, OCN, RUNX2, and COL1 were also significantly higher in the Ti3C2–PVP group than in the control group. The findings of the photothermal modulation of osteogenic differentiation were further confirmed by ALP staining (Fig. 7A and B) and alizarin red staining (Fig. 7C and D). After 7 and 14 days of osteogenic induction, the group treated with Ti3C2–PVP and NIR showed the highest levels of ALP activity and the number of calcium salt deposits. Furthermore, the ALP activity and mineralization were also significantly higher in the Ti3C2–PVP group compared with the control group.
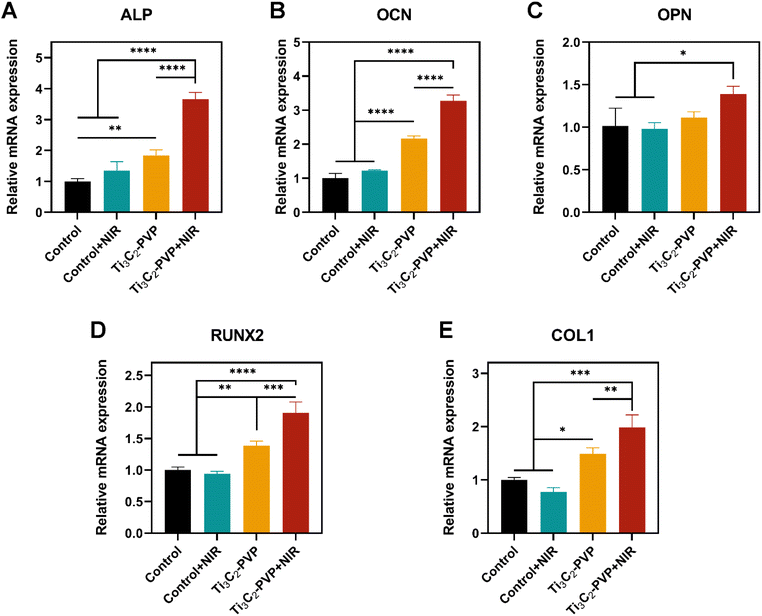 |
| Fig. 6 mRNA levels of the osteogenic differentiation-related genes ALP (A), OCN (B), OPN (C), RUNX2 (D) and COL1 (E) in BMSCs co-cultured with Ti3C2–PVP nanosheets with or without NIR irradiation (n = 3, *p < 0.05, **p < 0.01, ***p < 0.001, ****p < 0.0001). | |
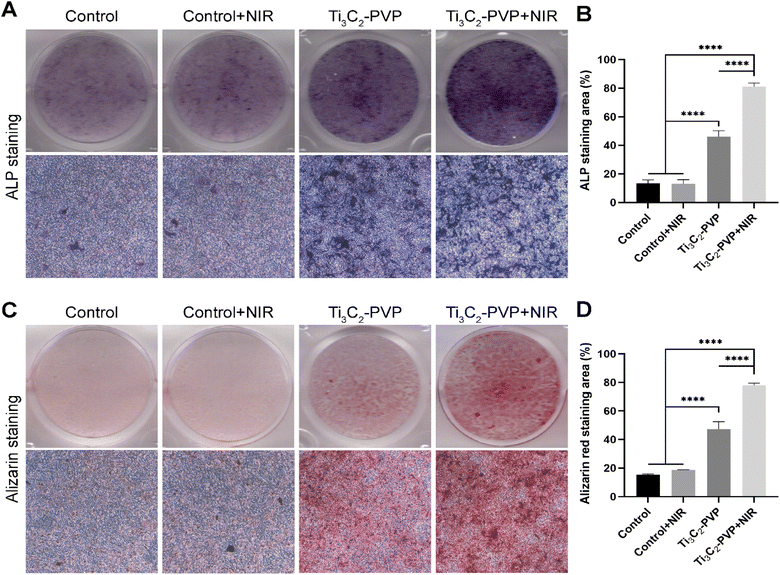 |
| Fig. 7 Ti3C2–PVP nanosheets and photothermal treatment promote the osteogenesis of BMSCs. (A and C) The ALP staining and the alizarin staining of BMSCs co-cultured with Ti3C2–PVP with or without NIR irradiation. (B and D) Corresponding quantitative data of ALP and alizarin staining (n = 3, ****p < 0.0001). | |
Discussion
Two-dimensional nanomaterials and their derivatives have been extensively studied for their potential to regulate stem cell behavior in tissue regeneration.21 Graphene has been widely regarded as a promising biocompatible scaffold for promoting the proliferation of mesenchymal stem cells and accelerating their differentiation into osteoblasts.30 MXenes, a family of two-dimensional materials consisting of transition metal carbides and nitrides, have been increasingly studied for their effects on stem cells.24 Despite the growing interest in the modulation of MXenes on stem cells, no studies have been reported on their photothermal modulation. Further research on the photothermal modulation of MXenes on stem cells would contribute to the advancement of MXene applications and photothermal therapy in regenerative medicine and tissue engineering.
Firstly, we successfully synthesized Ti3C2 MXene nanosheets using a method reported in the literature.29 The morphology of Ti3C2 MXene was confirmed by SEM and TEM imaging, which showed the typical two-dimensional structure of these materials. The XRD pattern was consistent with previous literature reports and indicated that the Ti3C2 MXene was successfully synthesized through chemical etching without any residue of the precursor Ti3AlC2.29 The results of UV-vis-NIR spectra analysis indicated that the modified Ti3C2–PVP nanosheets retained a strong NIR absorption capability, with a characteristic peak at 800 nm, which falls within the biological NIR window (650–950 nm).31 The stability of Ti3C2 MXene nanosheets in physiological solutions is a critical issue due to their aggregation in salt solutions and susceptibility to oxidation.25,26 This instability can negatively affect the material's biocompatibility and limit its practical use in the field of medicine. To address this issue, we used PVP as a surface modifier to improve the stability of the Ti3C2 nanosheets. The visual observations and DLS assays showed that the addition of PVP significantly improved the colloidal stability of the Ti3C2–PVP nanosheets in water, PBS, and DMEM solutions. The improved stability of the Ti3C2 MXene nanosheets in physiological solutions can be attributed to the presence of the organic chain of PVP. This organic chain reduces the strong interaction between the surface of the nanomaterial and biomolecules or ions, thereby providing a protective layer.32 This protective layer helps to maintain the stability of the nanomaterial in complex physiological environments, making it more suitable for biomedical applications.
Secondly, it was observed that the surface modification of Ti3C2–PVP nanosheets did not compromise their photothermal properties, as evidenced by the temperature increase induced by NIR irradiation of the Ti3C2–PVP colloidal solutions. Even at a low NIR power of 0.5 W cm−2 and a low concentration of 12.5 μg mL−1, the colloidal solutions of Ti3C2–PVP nanosheets were able to induce a temperature increase of 3.7 °C. In contrast to photothermal therapy for antitumor and antimicrobial purposes, which often require higher temperatures, photothermal therapy for tissue regeneration is limited to lower temperatures to minimize tissue damage, typically a temperature increase of only 3–5 °C above the body temperature.10,12–14 Therefore, the photothermal properties of Ti3C2–PVP nanosheets are well-suited for applications in regenerative medicine.
Thirdly, we found that the cytocompatibility of Ti3C2–PVP nanosheets is concentration-dependent. While high concentrations (50 μg mL−1) were observed to inhibit cell proliferation, low concentrations (12.5 μg mL−1) were found to promote cell proliferation. Notably, we found that high concentrations of Ti3C2–PVP nanosheets exhibited cytotoxicity only on the first day but did not inhibit cell proliferation at subsequent time points (3, 5, and 7 days). The biocompatibility of MXene materials is still under debate, with some studies suggesting that MXene is negligible at cytotoxic concentrations of up to 500 mg L−1,33 while others have reported a decrease in cell viability at a concentration of 1.5 μg mL−1.34 Our results showed that Ti3C2–PVP tended to accumulate on the cell membrane rather than on the substrate of 2D cell cultures, which may also contribute to cytotoxicity at high concentrations. Thus, from our perspective, Ti3C2–PVP nanosheets have good biocompatibility for tissue engineering applications.
Based on previous studies,35–37 we targeted a temperature of 42 °C for photothermal osteogenesis. The temperature of the co-cultures of BMSCs and Ti3C2–PVP was regulated by adjusting the NIR power and monitored with an infrared imager. Our results showed that the photothermal treatment promoted cell proliferation in the early stages. Similar results have been reported in the literature, where photothermal treatment at 40–43 °C promoted the proliferation of preosteoblasts at 24 and 48 h.13 In addition, we observed that cells exposed to Ti3C2–PVP and NIR exhibited a flatter morphology, better spreading and osteoblastic morphology. This observation is in consistent with previous reports, indicating that wider cell spreading and formation of many filopodia can promote osteogenic differentiation of stem cells.38
Fourthly, to evaluate the osteogenic potential of Ti3C2–PVP nanosheets, we utilized qRT-PCR, ALP staining, and ARS staining techniques to measure the expression of osteogenesis-related genes, including ALP, OCN, OPN, RUNX2, and COL1,39 as well as the ALP activity and mineralization of BMSCs. Our results showed that Ti3C2–PVP significantly promoted the osteogenic differentiation of BMSCs. These findings are consistent with several recent studies that have confirmed the good osteogenic activity of Ti3C2 MXene.40–46 The mechanism by which Ti3C2 MXene promotes osteogenic differentiation of MSCs may involve the activation of the Wnt/β-catenin signaling pathway.24
Next, our study presented novel insights into the modulation of osteogenic differentiation of BMSCs through photothermal conversion. We found that the photothermal effect of Ti3C2–PVP under NIR light further promoted the mRNA expression of osteogenic markers, ALP activity, and mineralization of BMSCs. Gentle photothermal stimulation has been widely demonstrated to promote osteogenesis.47–49 Heat shock protein (HSP) plays a key role in the regulation of photothermal osteogenesis.13,35,50 HSPs are a group of highly conserved molecular chaperones whose expression is generally increased in response to high temperature or other physicochemical stimuli.51 In recent years, studies have reported an important role of HSPs in bone metabolism.52,53 Thermal stimulation upregulates HSP expression and promotes osteogenesis through several signaling pathways, including ERK, Wnt/β-catenin, MAPK, and PI3K-Akt.50,54
In the field of photothermal treatment, the parameters used for tissue regeneration can vary. Previous studies have employed different times of NIR irradiation, ranging from longer periods of approximately 1 hour to shorter periods of approximately 60 seconds.35,50 In our study, we selected a 5 minute daily irradiation frequency, based on previous reports. During the 14 day experiment, the viability of BMSCs was maintained, indicating the safety of our photothermal treatment.
The study revealed that the photothermal effect of Ti3C2 represents a promising and emerging strategy in tissue engineering, though certain limitations exist in the current investigation. Specifically, in the absence of inorganic or polymer scaffold materials, Ti3C2–PVP nanosheets are susceptible to metabolic degradation by the body fluid environment, resulting in an unstable delivery of photothermal stimulation in the bone defect area when used alone. Therefore, limited by the physical properties of Ti3C2–PVP nanosheets, this study only explored their photothermal regulation of BMSCs, and animal experiments to investigate the pro-osteogenic efficiency of Ti3C2–PVP nanosheets in vivo were not conducted. Consequently, in future research, we plan to fabricate degradable composite films or implants by incorporating Ti3C2–PVP with biocompatible polymer materials. This will enable us to investigate the in vivo application strategies of Ti3C2–PVP and its photothermal modulation capability.
Conclusions
In this study, surface modification of Ti3C2 MXene nanosheets with PVP (Ti3C2–PVP) was employed to enhance their colloidal stability in physiological solutions. Ti3C2 nanosheets were found to exhibit good biocompatibility and desirable photothermal properties, making them a promising candidate for regenerative medicine applications. The possibility of using thermal stimulation instead of growth factors in bone tissue engineering was explored by co-culturing Ti3C2–PVP nanosheets with BMSCs and irradiating with 808 nm NIR. Our results showed that the photothermal stimulation generated by Ti3C2–PVP nanosheets was able to promote cell proliferation, adhesion, and osteogenic differentiation of BMSCs. In conclusion, photothermal conversion of Ti3C2–PVP nanosheets can modulate the cellular functions of BMSCs and has potential for bone tissue engineering applications.
Author contributions
Jiebing Zhang: conceptualization, data curation, investigation, methodology, visualization, writing – original draft. Shuang Tang: data curation, formal analysis. Ning Ding: methodology. Ping Ma: resources, software. Zutai Zhang: conceptualization, funding acquisition, writing – review & editing. Project administration.
Conflicts of interest
There are no conflicts to declare.
Acknowledgements
This study was supported by Beijing Stomatological Hospital, Capital Medical University Young Scientist Program (No. YSP202009).
References
- R. T. Annamalai, X. Hong, N. G. Schott, G. Tiruchinapally, B. Levi and J. P. Stegemann, Biomaterials, 2019, 208, 32–44 CrossRef CAS PubMed
.
- C. J. Norris, G. J. Meadway, M. J. O'Sullivan, I. P. Bond and R. S. Trask, Adv. Funct. Mater., 2011, 21, 3624–3633 CrossRef CAS
.
- G. Zhu, T. Zhang, M. Chen, K. Yao, X. Huang, B. Zhang, Y. Li, J. Liu, Y. Wang and Z. Zhao, Bioact. Mater., 2021, 6, 4110–4140 CrossRef CAS PubMed
.
- X. Hu, Y. Wang, Y. Tan, J. Wang, H. Liu, Y. Wang, S. Yang, M. Shi, S. Zhao, Y. Zhang and Q. Yuan, Adv. Mater., 2017, 29, 1605235 CrossRef PubMed
.
- T. Yin and L. Li, J. Clin. Investig., 2006, 116, 1195–1201 CrossRef CAS PubMed
.
- T. J. Blokhuis and J. J. Arts, Injury, 2011, 42(suppl. 2), S26–S29 CrossRef PubMed
.
- H. S. Azevedo and I. Pashkuleva, Adv. Drug Deliv. Rev., 2015, 94, 63–76 CrossRef CAS PubMed
.
- C. J. Kowalczewski and J. M. Saul, Front. Pharmacol., 2018, 9, 513 CrossRef PubMed
.
- H. A. Rather, D. Jhala and R. Vasita, Mater. Sci. Eng., C, 2019, 103, 109761 CrossRef CAS PubMed
.
- Y. Kong, J. Duan, F. Liu, L. Han, G. Li, C. Sun, Y. Sang, S. Wang, F. Yi and H. Liu, Chem. Soc. Rev., 2021, 50, 12828–12872 RSC
.
- A. J. McGrath, Y. H. Chien, S. Cheong, D. A. Herman, J. Watt, A. M. Henning, L. Gloag, C. S. Yeh and R. D. Tilley, ACS Nano, 2015, 9, 12283–12291 CrossRef CAS
.
- S. Sayed, O. Faruq, M. Hossain, S. B. Im, Y. S. Kim and B. T. Lee, Mater. Sci. Eng., C, 2019, 105, 110027 CrossRef CAS PubMed
.
- X. Zhang, G. Cheng, X. Xing, J. Liu, Y. Cheng, T. Ye, Q. Wang, X. Xiao, Z. Li and H. Deng, J. Phys. Chem. Lett., 2019, 10, 4185–4191 CrossRef CAS PubMed
.
- R. Nørgaard, M. Kassem and S. I. Rattan, Ann. N. Y. Acad. Sci., 2006, 1067, 443–447 CrossRef PubMed
.
- S. K. Sharma, N. Shrivastava, F. Rossi, L. D. Tung and N. T. K. Thanh, Nano Today, 2019, 29, 100795 CrossRef CAS
.
- R. Kurapati, K. Kostarelos, M. Prato and A. Bianco, Adv. Mater., 2016, 28, 6052–6074 CrossRef CAS
.
- K. Yang, S. Zhang, G. Zhang, X. Sun, S.-T. Lee and Z. Liu, Nano Lett., 2010, 10, 3318–3323 CrossRef CAS PubMed
.
- J. R. Melamed, R. S. Edelstein and E. S. Day, ACS Nano, 2015, 9, 6–11 CrossRef CAS
.
- J.-W. Xu, K. Yao and Z.-K. Xu, Nanoscale, 2019, 11, 8680–8691 RSC
.
- S. A. Leon, S. O. Asbell, H. H. Arastu, G. Edelstein, A. J. Packel, S. Sheehan, I. Daskal, G. G. Guttmann and I. Santos, Int. J. Hyperthermia, 1993, 9, 77–87 CrossRef CAS PubMed
.
- A. Murali, G. Lokhande, K. A. Deo, A. Brokesh and A. K. Gaharwar, Mater. Today, 2021, 50, 276–302 CrossRef CAS PubMed
.
- J. Xuan, Z. Wang, Y. Chen, D. Liang, L. Cheng, X. Yang, Z. Liu, R. Ma, T. Sasaki and F. Geng, Angew. Chem., Int. Ed., 2016, 55, 14569–14574 CrossRef CAS PubMed
.
- K. Huang, Z. Li, J. Lin, G. Han and P. Huang, Chem. Soc. Rev., 2018, 47, 5109–5124 RSC
.
- D. Cui, N. Kong, L. Ding, Y. Guo, W. Yang and F. Yan, Adv. Healthc. Mater., 2021, 10, e2101215 CrossRef PubMed
.
- H. Lin, X. Wang, L. Yu, Y. Chen and J. Shi, Nano Lett., 2017, 17, 384–391 CrossRef CAS
.
- Y. Chae, S. J. Kim, S. Y. Cho, J. Choi, K. Maleski, B. J. Lee, H. T. Jung, Y. Gogotsi, Y. Lee and C. W. Ahn, Nanoscale, 2019, 11, 8387–8393 RSC
.
- X. Liu, Y. Xu, Z. Wu and H. Chen, Macromol. Biosci., 2013, 13, 147–154 CrossRef CAS PubMed
.
- L. E. Smith, S. Rimmer and S. MacNeil, Biomaterials, 2006, 27, 2806–2812 CrossRef CAS PubMed
.
- M. Alhabeb, K. Maleski, B. Anasori, P. Lelyukh, L. Clark, S. Sin and Y. Gogotsi, Chem. Mater., 2017, 29, 7633–7644 CrossRef CAS
.
- T. R. Nayak, H. Andersen, V. S. Makam, C. Khaw, S. Bae, X. Xu, P. L. Ee, J. H. Ahn, B. H. Hong, G. Pastorin and B. Özyilmaz, ACS Nano, 2011, 5, 4670–4678 CrossRef CAS PubMed
.
- M. F. Tsai, S. H. Chang, F. Y. Cheng, V. Shanmugam, Y. S. Cheng, C. H. Su and C. S. Yeh, ACS Nano, 2013, 7, 5330–5342 CrossRef CAS PubMed
.
- K. M. Koczkur, S. Mourdikoudis, L. Polavarapu and S. E. Skrabalak, Dalton Trans., 2015, 44, 17883–17905 RSC
.
- D. Zhang, W. Zheng, X. Li, A. Li, N. Ye, L. Zhang, Y. Liu, X. Liu, R. Zhang, M. Wang, J. Cheng, H. Yang and M. Gong, Carbon, 2021, 178, 810–821 CrossRef CAS
.
- S. Kyrylenko, O. Gogotsi, I. Baginskiy, V. Balitskyi, V. Zahorodna, Y. Husak, I. Yanko, M. Pernakov, A. Roshchupkin, M. Lyndin, B. B. Singer, V. Buranych, A. Pogrebnjak, O. Sulaieva, O. Solodovnyk, Y. Gogotsi and M. Pogorielov, ACS Appl. Mater. Interfaces, 2022, 14, 28683–28696 CrossRef CAS PubMed
.
- L. Tong, Q. Liao, Y. Zhao, H. Huang, A. Gao, W. Zhang, X. Gao, W. Wei, M. Guan, P. K. Chu and H. Wang, Biomaterials, 2019, 193, 1–11 CrossRef CAS PubMed
.
- J. Chen, Z. D. Shi, X. Ji, J. Morales, J. Zhang, N. Kaur and S. Wang, Tissue Eng., Part A, 2013, 19, 716–728 CrossRef CAS PubMed
.
- C. P. Ye, B. C. Heng, H. Liu, W. S. Toh and T. Cao, Cell Biochem. Funct., 2007, 25, 267–276 CrossRef CAS PubMed
.
- R. Peng, X. Yao, B. Cao, J. Tang and J. Ding, Biomaterials, 2012, 33, 6008–6019 CrossRef CAS PubMed
.
- S. Khoshniat, A. Bourgine, M. Julien, M. Petit, P. Pilet, T. Rouillon, M. Masson, M. Gatius, P. Weiss, J. Guicheux and L. Beck, Bone, 2011, 48, 894–902 CrossRef CAS PubMed
.
- R. Huang, X. Chen, Y. Dong, X. Zhang, Y. Wei, Z. Yang, W. Li, Y. Guo, J. Liu, Z. Yang, H. Wang and L. Jin, ACS Appl. Bio Mater., 2020, 3, 2125–2131 CrossRef CAS PubMed
.
- X. Mi, Z. Su, Y. Fu, S. Li and A. Mo, Biomed. Mater., 2022, 17, 035002 CrossRef PubMed
.
- Y. Fu, J. Zhang, H. Lin and A. Mo, Mater. Sci. Eng., C, 2021, 118, 111367 CrossRef CAS PubMed
.
- K. Chen, Y. Chen, Q. Deng, S.-H. Jeong, T.-S. Jang, S. Du, H.-E. Kim, Q. Huang and C.-M. Han, Mater. Lett., 2018, 229, 114–117 CrossRef CAS
.
- Y. Fu, S. Huang, Z. Feng, L. Huang, X. Zhang, H. Lin and A. Mo, ACS Biomater. Sci. Eng., 2023, 9, 900–917 CrossRef CAS PubMed
.
- S. Pan, J. Yin, L. Yu, C. Zhang, Y. Zhu, Y. Gao and Y. Chen, Adv. Sci., 2020, 7, 1901511 CrossRef CAS PubMed
.
- S. H. Lee, S. Jeon, X. Qu, M. S. Kang, J. H. Lee, D.-W. Han and S. W. Hong, Nano Converg., 2022, 9, 38 CrossRef CAS PubMed
.
- Y. Zhao, X. Peng, D. Wang, H. Zhang, Q. Xin, M. Wu, X. Xu, F. Sun, Z. Xing, L. Wang, P. Yu, J. Xie, J. Li, H. Tan, C. Ding and J. Li, Adv. Sci., 2022, 9, 2204535 CrossRef CAS PubMed
.
- Y. Wu, Q. Liao, L. Wu, Y. Luo, W. Zhang, M. Guan, H. Pan, L. Tong, P. K. Chu and H. Wang, ACS Nano, 2021, 15, 17854–17869 CrossRef CAS PubMed
.
- Q. Fan, J. Bai, H. Shan, Z. Fei, H. Chen, J. Xu, Q. Ma, X. Zhou and C. Wang, Bioact. Mater., 2021, 6, 4014–4026 CrossRef CAS PubMed
.
- H. Shan, X. Zhou, B. Tian, C. Zhou, X. Gao, C. Bai, B. Shan, Y. Zhang, S. Sun, D. Sun, Q. Fan, X. Zhou, C. Wang and J. Bai, Biomaterials, 2022, 284, 121482 CrossRef CAS PubMed
.
- P. G. Richardson, C. S. Mitsiades, J. P. Laubach, S. Lonial, A. A. Chanan-Khan and K. C. Anderson, Br. J. Haematol., 2011, 152, 367–379 CrossRef CAS PubMed
.
- K. Hang, C. Ye, E. Chen, W. Zhang, D. Xue and Z. Pan, Cell Stress Chaperones, 2018, 23, 1153–1164 CrossRef PubMed
.
- L. Li, L. Wang, Q. D. You and X. L. Xu, J. Med. Chem., 2020, 63, 1798–1822 CrossRef CAS PubMed
.
- L. Tan, Y. Hu, M. Li, Y. Zhang, C. Xue, M. Chen, Z. Luo and K. Cai, Chem. Eng. J., 2022, 431, 133382 CrossRef CAS
.
|
This journal is © The Royal Society of Chemistry 2023 |