DOI:
10.1039/D2TC01638A
(Paper)
J. Mater. Chem. C, 2022,
10, 8776-8782
Mg(H2O)6[(IO2(OH))2(IO3)]2: a new iodate with a very large band gap and optical anisotropy†
Received
21st April 2022
, Accepted 9th May 2022
First published on 10th May 2022
Abstract
Centimeter-sized large crystals of a new magnesium iodate, Mg(H2O)6[(IO2(OH))2(IO3)]2, have been successfully grown by a facile aqua-solution method. The title compound exhibits an interesting structural feature, namely, [(IO2(OH))2(IO3)]− pseudo-chains interacting with [Mg(H2O)6]2+ octahedra via the hydrogen bonds. Mg(H2O)6[(IO2(OH))2(IO3)]2 possessing a large band gap of 4.10 eV exhibits the largest birefringence (0.230 @1064 nm) among the iodate systems containing alkali, alkaline earth, or rare earth metal cations, indicating that the compound is a promising birefringent material that could be applied in the ultraviolet region. The electron density difference map indicates that the great birefringence of the material is attributed to the synergistic effect of the iodate groups and the coordinated water molecules. This work also successfully provides a new method for functional crystals with large birefringence via a facile aqua-solution method.
Introduction
Iodate is an important family of multifunctional materials that can be applied to various industrial processes such as photonics, optical communications, medical lasers, and data storage fields.1–4 Many functional iodate materials with macroscopic noncentrosymmetric structures revealing interesting properties have been discovered with the aid of stereochemically active lone pair electrons.5 In addition, iodates can exhibit broad transmittance windows up to mid-infrared regions, and high-quality large crystals of iodates are often easily grown. Thus, a number of famous nonlinear optical (NLO) materials such as α-HIO3, α-LiIO3, and KIO3 as well as newly designed iodates satisfying the ever-growing requirements of the industry have been continuously discovered in the past several decades.6–26 In general, basic building units observed from the metal iodates are IO3 and IO4 polyhedra. The units are also able to generate dimers, trimers, and even more complicated groups such as I2O5, I3O8, I4O11, I3O9, etc.3,14,26 Further extension via strong intermolecular interactions can also result in a variety of iodate groups with various frameworks.26 The variable iodate building blocks not only enrich the structural chemistry, but also provide many interesting compounds with outstanding optical properties including a large SHG response and birefringence.27–36
It is well known that birefringence is an important parameter for viable NLO and birefringent materials. To design viable materials with large birefringence, several building blocks with large polarizability and anisotropy such as the coplanar groups (BO3, B3O6, C3O3N3, etc.), lone pair cations (Pb2+, Bi3+, I5+, etc.), and d0 transition metal cations (Nb5+, Mo6+, etc.) with second-order Jahn–Teller effect have been recommended.23,37,38 The higher density and the optimal arrangement of the building blocks through suitable interactions such as hydrogen bonds in the structures also could boost the optical anisotropy of the target compounds. Guided by the above-mentioned strategy, a variety of promising NLO and birefringent crystals have been designed thus far. Specifically, several iodates with large birefringence such as Bi(IO3)F2 and Ba2[VO2F2(IO3)2]IO3 have been constructed by introducing lone pair cations or d0 transition metal cations.8,11 Unfortunately, however, most of the materials possess quite narrow band gaps. Thus, to broaden the band gaps, iodates containing main group metal cations such as Ba2[GaF4(IO3)2]IO3, LiGaF2(IO3)2, and Ba[InF3(IO3)2] have been prepared, where the combination of iodates and distorted octahedra groups was proven to be an effective way to design interesting birefringent materials with large band gaps.39–41 In this paper, we have explored the Mg2+ cation containing iodate system to discover new birefringent materials. By doing so, a new magnesium iodate, Mg(H2O)6[(IO2(OH))2(IO3)]2, has been successfully obtained via the facile aqua-solution method at room temperature. Hydrogen bonding interactions between [Mg(H2O)6]2+ octahedra and iodate groups could generate a novel structural feature.42 In addition, the coordinated aqua ligands may also be expected to contribute to improving the birefringence.43
Experimental section
Reagents
Mg(NO3)2·6H2O (Yakuri, 99%) and HIO3 (Alfa, 99%) were used as reagents as received.
Single crystal growth
Single crystals of Mg(H2O)6[(IO2(OH))2(IO3)]2 were grown by the aqua-solution method at room temperature. Mg(NO3)2·6H2O (1 mmol), HIO3 (3 mmol), and 5 mL of distilled water were mixed in a dish and thoroughly stirred using a glass rod to dissolve the reagents. Transparent needle like crystals were grown for a week in 75% yield based on Mg(NO3)2·6H2O once the water slowly evaporated at room temperature.
Measurements
The single crystal X-ray diffraction data of Mg(H2O)6[(IO2(OH))2(IO3)]2 were collected at room temperature via a Bruker D8 QUEST diffractometer with a graphite-monochromated MoKα radiation source (λ = 0.71073 Å) at the Advanced Bio-Interface Core Research Facility, Sogang University. Data reduction and absorption correction were made using the SAINT and SADABS programs, respectively.44,45 The crystal structure was solved using the OLEX2 package.46 The program PLATON was applied to validate if any higher symmetry is missing there.47 Crystallographic data, structure refinement information, atomic coordinates, equivalent isotropic displacement parameters, and bond valence sums of all atoms except for H atoms are listed in the ESI.† Powder X-ray diffraction data were collected on a Rigaku Mini Flex 600 diffractometer using Cu Kα (λ = 1.5406 Å) radiation with 40 kV and 15 mA at room temperature. The well-ground polycrystalline sample mounted on a sample holder was scanned in the 2θ range of 5–70° at a scan speed of 20° min−1 and a scan step width of 0.02°. The measured diffraction pattern of the title compound matched well with the calculated data generated from single crystal X-ray diffraction (Fig. 1).
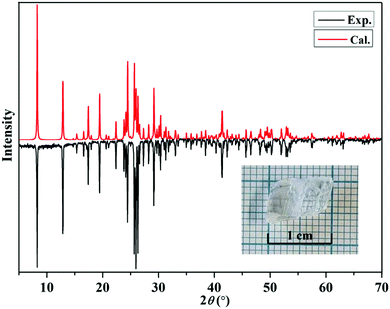 |
| Fig. 1 Experimental and calculated powder XRD patterns of Mg(H2O)6[(IO2(OH))2(IO3)]2. Inset: The picture of an as-grown large crystal. | |
Infrared (IR) spectra were recorded on a Thermo Scientific Nicolet iS50 FT-IR spectrometer in the spectral range of 500 to 4000 cm−1. The ground sample was made to be in contact with the diamond attenuated-total-reflectance crystal.
Ultraviolet-visible (UV-vis) diffuse-reflectance spectra for Mg(H2O)6[(IO2(OH))2(IO3)]2 were obtained on a Lambda 1050 scan UV-vis-NIR spectrophotometer over the spectral range of 200–700 nm at room temperature. The reflection spectra were converted to the absorbance data using the Kubelka–Munk function.48
Thermogravimetric analysis (TGA) was performed using a SCINCO TGA-N 1000 thermal analyzer. The ground polycrystalline sample was loaded into an alumina crucible and heated to 900 °C at a rate of 10 °C min−1 under flowing air. Differential scanning calorimetry data were also obtained on a TA DSC-Q2000 from room temperature to 350 °C at a heating rate of 10 °C min−1 under flowing nitrogen.
First-principles density functional theory calculations were performed using the CASTEP package.49 The band structure, density of states, and the optical properties were calculated by using the Perdew–Burke–Ernzerhof generalized gradient approximation (GGA).50–52 A plane-wave cut-off energy of 925 eV was chosen. To obtain a good convergence of electronic structures and optical properties, the dense k-point sampling less than 0.03 Å−1 was adopted.
Results and discussion
Crystal structure
Mg(H2O)6[(IO2(OH))2(IO3)]2 crystallizes in the monoclinic space group, P21/c. In an asymmetric unit, one [Mg(H2O)6]2+, four IO2(OH), and two IO3 units exist (Fig. S1, ESI†). The unique Mg2+ cation is coordinated by six H2O molecules to form the [Mg(H2O)6]2+ octahedron, in which the Mg–O distances are in the range of 2.036–2.110 Å. While both I(1) and I(3) are linked with two O atoms and one OH− to form IO2(OH) groups, I(2) is connected by three O atoms to form the IO3 trigonal pyramid. The distances between I and O atoms observed in IO2(OH) and IO3 groups range from 1.780 to 1.913 Å. Long atom contacts such as I(1)⋯O(7) [2.453 Å], I(2)⋯O(9) [2.611 Å], and I(3)⋯O(11) [2.461 Å] are also observed. Considering those longer interactions, [(IO2(OH))2(IO3)]− infinite tubules that are running along the c-axis are monitored (Fig. 2a and b). Finally, hydrogen bonding interactions between the [Mg(H2O)6]2+ octahedra and [(IO2(OH))2(IO3)]− tubules complete the whole structure of Mg(H2O)6[(IO2(OH))2(IO3)]2 (Fig. 2c and d). Bond valence sum calculations on the Mg2+, I5+, OH−, and O2− result in values of 2.14, 5.12–5.23, 1.28–1.34, and 1.68–2.03, respectively.42,53
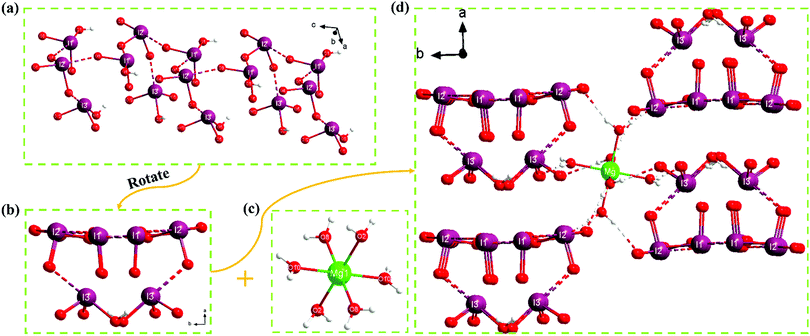 |
| Fig. 2 [(IO2(OH))2(IO3)]− infinite tubules viewed along the (a) b-axis and (b) c-axis, and the (c) [Mg(H2O)6]2+ octahedron in Mg(H2O)6[(IO2(OH))2(IO3)]2. (d) Hydrogen bonding interactions between the [Mg(H2O)6]2+ octahedron and [(IO2(OH))2(IO3)]− infinite tubules. | |
IR spectroscopy
The IR spectrum of the reported compound reveals vibrations occurring from Mg–O, I–O, and O–H bonds (Fig. S2, ESI†). Weak bands at ca. 1413 and 823 cm−1 are associated with the Mg–O bonds.54,55 The bands found at ca. 779, 750, 711, 552, 525, and 517 cm−1 are attributable to the I–O vibrations.32,42,56 The peaks occurring at ca. 3365 and 1632 cm−1 are responsible for O–H bonds.42,43
UV-vis diffuse reflectance spectroscopy
The UV-vis diffuse reflectance spectrum suggests that Mg(H2O)6[(IO2(OH))2(IO3)]2 possesses a short cut-off edge of ca. 262 nm (Fig. 3). The cut-off edge is smaller than most iodates containing alkali or alkaline earth, lone pair, and rare earth metal cations such as LiIO3 (280 nm), RbIO2F2 (295 nm), Ba[FeF4(IO3)] (281 nm), Bi2(IO4)(IO3)3 (376 nm), and Ce(IO3)2F2 (452 nm).10,12,33,57,58 Hence, the reported iodate with a broad band gap could be a potential birefringent crystal that can be applied in the UV region.
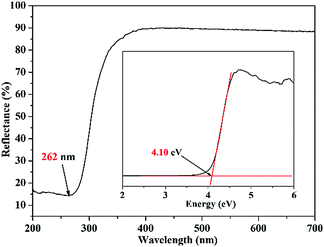 |
| Fig. 3 UV-vis diffuse reflectance spectrum of Mg(H2O)6[(IO2(OH))2(IO3)]2. | |
Thermal analysis
As seen in the TGA diagram, Mg(H2O)6[(IO2(OH))2(IO3)]2 reveals three thermal decomposition steps (Fig. S3, ESI†). Initially, the title compound exhibits a weight loss of 12.5% between 84 and 227 °C attributed to the loss of eight H2O molecules from Mg(H2O)62+ and IO2(OH) groups, which are in agreement with the endothermic peaks occurring at 86 to 204 °C in the DSC diagram. And then, 2 equivalents of I2 and 5 equivalents of O2 are lost at ca. 344 and 433 °C, calculated (experimental): 56.3% (56.0%). Finally, I2 and 5/2 O2 are released between 540 and 710 °C, calculated (experimental): 27.8% (Exp. 27.6%).
Electronic structure calculations
The electronic structure calculations predict a band gap of 2.83 eV for Mg(H2O)6[(IO2(OH))2(IO3)]2, which is significantly smaller than the experimental value of 4.10 eV (Fig. 4 and Fig. S4, ESI†). The underestimating tendency of the band gap energy obtained by the electronic band structure calculations from the GGA functional attributable to the PBE exchange-correlation energy has been well documented.59,60 However, the calculations are still useful in identifying important orbital interactions with a reliable trend. As seen from the band structure, the title compound belongs to an indirect band gap compound because the valence band maximum and the conduction band minimum are located at different points. The conduction band minimum and the valence band maximum are dominated by the I 5p and O 2p orbitals, respectively, with small number of mixing from the I 5s orbitals (Fig. 4). Thus, the IO3 and IO2(OH) groups occupying the highest valence band and the lowest conduction band mainly determine the optical properties of Mg(H2O)6[(IO2(OH))2(IO3)]2.
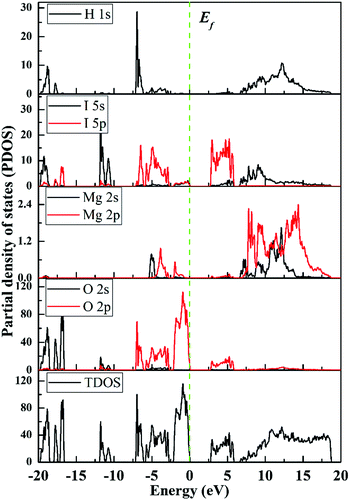 |
| Fig. 4 Density of states for Mg(H2O)6[(IO2(OH))2(IO3)]2. | |
To confirm the longer interactions between the I and O groups (bond lengths ≥ 2.46 Å), the electron localization function was calculated (Fig. 5a).26,61 The obvious gaps between the I1⋯O7, I2⋯O9, and I3⋯O11 strongly suggest that the interactions between them are intermolecular interactions rather than the normal covalent I–O bonds in these structures.
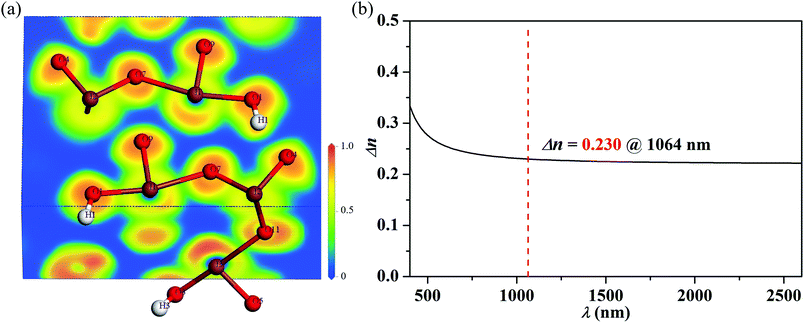 |
| Fig. 5 (a) Electron localization function diagram and (b) calculated birefringence for Mg(H2O)6[(IO2(OH))2(IO3)]2. | |
Birefringence
Based on the plot of calculated birefringence in Fig. 5b, Mg(H2O)6[(IO2(OH))2(IO3)]2 possesses a very large birefringence of 0.230 @1064 nm, which is much larger than those of most reported iodates. In fact, this value is the largest one among the iodate systems containing alkali, alkaline earth, or rare earth metal cations (ESI†). To further investigate the source of the birefringence, the electron density difference map was calculated (Fig. S5, ESI†). By doing so, we found that the strong covalent I–O and O–H bonds existing in IO3, IO2(OH), and H2O should contribute to the large birefringence of Mg(H2O)6[(IO2(OH))2(IO3)]2. Once we substitute Mg2+ for the large Ba2+ cation and remove In3+ in Ba[InF3(IO3)2], we were able to enhance the density of I–O groups from 4.9 × 10−3 Å−3 to 10.8 × 10−3 Å−3. Although Mg(H2O)6[(IO2(OH))2(IO3)]2 consists of a weaker distortive octahedra (Δd = 0.15), the compound still possesses a larger birefringence than those of Ba[InF3(IO3)2] (0.172 @1064 nm; Δd(InO2F4) = 0.35) and LiGaF2(IO3)2 (0.181 @1064 nm; Δd(GaF2O4) = 0.25). The band gap and birefringence for the reported typical iodates are listed in Table 1. As shown in Table 1, Mg(H2O)6[(IO2(OH))2(IO3)]2 exhibits a good balance between the band gap and birefringence. Even compared with several commercial birefringent crystals such as LiNbO3 (0.0836 @633 nm), α-BaB2O4 (0.1222 @532 nm), and CaCO3 (0.171 @633 nm), Mg(H2O)6[(IO2(OH))2(IO3)]2 exhibits a very attractive birefringence that can be applied to a promising UV birefringent crystal.63–65
Table 1 Band gap and birefringence (Δn @1064 nm) for typical iodates
Compound |
band gap (eV) |
Δn @1064 nm |
Ref. |
RbIO3
|
4.0 |
0.063 |
10
|
YI5O14
|
3.82 |
0.091 |
62
|
GdI5O14
|
4.07 |
0.092 |
62
|
α-AgI3O8
|
3.78 |
0.208 |
27
|
β-AgI3O8
|
3.59 |
0.210 |
27
|
NaI3O8
|
3.93 |
0.213 |
26
|
Ba2[FeF4(IO3)2]IO3
|
3.9 |
0.125 |
57
|
LiGaF2(IO3)2
|
4.33 |
0.181 |
40
|
Ba[InF3(IO3)2]
|
4.35 |
0.172 |
41
|
Bi(IO3)F2
|
3.97 |
0.209 |
8
|
Ce(IO3)4
|
2.17 |
0.039 (@546 nm) |
58
|
Mg(H2O)6[(IO2(OH))2(IO3)]2
|
4.10 |
0.230 |
This work |
Conclusion
Crystals of a new magnesium iodate, Mg(H2O)6[(IO2(OH))2(IO3)]2, consisting of Mg(H2O)6, IO3, and IO2(OH) groups have been grown via an aqua-solution method. The reported material reveals an interesting pseudo-3D structural feature that is composed of [Mg(H2O)6]2+ octahedra and [(IO2(OH))2(IO3)]− tubules through hydrogen bonding interactions. The compound possesses a large band gap of 4.10 eV and a short UV cut-off edge of 262 nm. Interestingly, the synergistic effect of distorted [Mg(H2O)6]2+, IO3, and IO2(OH) groups resulted in a very large calculated birefringence of 0.230@1064 nm. Mg(H2O)6[(IO2(OH))2(IO3)]2 maintains a good balance between the band gap and birefringence, which successfully enables the material as a potential birefringent crystal in the UV region. We are in the process of introducing F− anions into a similar framework structure to shorten the UV cut-off edge while maintaining the large birefringence.
Conflicts of interest
There are no conflicts to declare.
Acknowledgements
This research was supported by the National Research Foundation of Korea (NRF) funded by the Ministry of Science and ICT (Grant No. 2018R1A5A1025208 and 2019R1A2C3005530).
Notes and references
- P. S. Halasyamani and K. R. Poeppelmeier, Noncentrosymmetric Oxides, Chem. Mater., 1998, 10, 2753–2769 CrossRef CAS.
- H. Y. Chang, S. H. Kim, P. S. Halasyamani and K. M. Ok, Alignment of lone pairs in a new polar material: Synthesis, characterization, and functional properties of Li2Ti(IO3)6, J. Am. Chem. Soc., 2009, 131, 2426–2427 CrossRef CAS PubMed.
- R. E. Sykora, K. M. Ok, P. S. Halasyamani and T. E. Albrecht-Schmitt, Structural modulation of molybdenyl iodate architectures by alkali metal cations in AMoO3(IO3) (A = K, Rb, Cs): A facile route to new polar materials with large SHG responses, J. Am. Chem. Soc., 2002, 124, 1951–1957 CrossRef CAS PubMed.
- C. L. Hu and J. G. Mao, Recent advances on second-order NLO materials based on metal iodates, Coord. Chem. Rev., 2015, 288, 1–17 CrossRef CAS.
- C. T. Chen, Y. C. Wu and R. K. Li, The anionic group theory of the non-linear optical effect and its applications in the development of new high-quality NLO crystals in the borate series, Int. Rev. Phys. Chem., 1988, 8, 65–91 Search PubMed.
- X. L. Chen and K. M. Ok, Recent Advances in Oxide-based Nonlinear Optical Materials with Wide Infrared Transparency Beyond 6 μm, Chem. – Asian J., 2020, 15, 3709–3716 CrossRef CAS PubMed.
- M. Zhang, C. Hu, T. Abudouwufu, Z. H. Yang and S. L. Pan, Functional Materials Design via Structural Regulation Originated from Ions Introduction: A Study Case in Cesium Iodate System, Chem. Mater., 2018, 30, 1136–1145 CrossRef CAS.
- F. F. Mao, C. L. Hu, X. Xu, D. Yan, B. P. Yang and J. G. Mao, Bi(IO3)F2: The First Metal Iodate Fluoride with a Very Strong Second Harmonic Generation Effect, Angew. Chem., Int. Ed., 2017, 56, 2151–2155 CrossRef CAS PubMed.
- H. Fan, C. S. Lin, K. C. Chen, G. Peng, B. X. Li, G. Zhang, X. F. Long and N. Ye, (NH4)Bi2(IO3)2F5: An Unusual Ammonium-containing Metal Iodate Fluoride Showing Strong Second Harmonic Generation (SHG) Response and Thermochromic Behavior, Angew. Chem., Int. Ed., 2020, 59, 5268–5272 CrossRef CAS PubMed.
- Q. Wu, H. M. Liu, F. C. Jiang, L. Kang, L. Yang, Z. S. Lin, Z. G. Hu, X. G. Chen, X. G. Meng and J. G. Qin, RbIO3 and RbIO2F2: Two Promising Nonlinear Optical Materials in Mid-IR Region and Influence of Partially Replacing Oxygen with Fluorine for Improving Laser Damage Threshold, Chem. Mater., 2016, 28, 1413–1418 CrossRef CAS.
- H. W. Yu, M. L. Nisbet and K. R. Poeppelmeier, Assisting the Effective Design of Polar Iodates with Early Transition- Metal Oxide Fluoride Anions, J. Am. Chem. Soc., 2018, 140, 8868–8876 CrossRef CAS PubMed.
-
V. G. Dmitriev, G. G. Gurzadyan and D. N. Nikogosyan, Handbook of nonlinear optical crystals, Springer, 2013 Search PubMed.
- Z. G. Xia and K. R. Poeppelmeier, Chemistry-Inspired Adaptable Framework Structures, Acc. Chem. Res., 2017, 50, 1222–1230 CrossRef CAS PubMed.
- J. Chen, C. L. Hu, F. Kong and J. G. Mao, High-Performance Second-Harmonic-Generation (SHG) Materials: New Developments and New Strategies, Acc. Chem. Res., 2021, 54, 2775–2783 CrossRef CAS PubMed.
- K. M. Ok, Toward the Rational Design of Novel Noncentrosymmetric Materials: Factors Influencing the Framework Structures, Acc. Chem. Res., 2016, 49, 2774–2785 CrossRef CAS PubMed.
- Y. Wang, B. B. Zhang, Z. H. Yang and S. L. Pan, Cation-Tuned Synthesis of Fluorooxoborates: Approaching the Optimal Deep-Ultraviolet Nonlinear Optical Materials, Angew. Chem., Int. Ed., 2018, 130, 2172–2176 CrossRef.
- G. Q. Shi, Y. Wang, F. F. Zhang, B. B. Zhang, Z. H. Yang, X. L. Hou, S. L. Pan and K. R. Poeppelmeier, Finding the Next Deep-Ultraviolet Nonlinear Optical Material: NH4B4O6F, J. Am. Chem. Soc., 2017, 139, 10645–10648 CrossRef CAS PubMed.
- B. B. Zhang, G. Q. Shi, Z. H. Yang, F. F. Zhang and S. L. Pan, Fluorooxoborates: Beryllium-Free Deep-Ultraviolet Nonlinear Optical Materials without Layered Growth, Angew. Chem., Int. Ed., 2017, 56, 3916–3919 CrossRef CAS PubMed.
- M. Mutailipu, M. Zhang, B. B. Zhang, L. Y. Wang, Z. H. Yang, X. Zhou and S. L. Pan, SrB5O7F3 Functionalized with [B5O9F3]6− Chromophores: Accelerating the Rational Design of Deep-Ultraviolet Nonlinear Optical Materials, Angew. Chem., Int. Ed., 2018, 130, 6203–6207 CrossRef.
- X. F. Wang, Y. Wang, B. B. Zhang, F. F. Zhang, Z. H. Yang and S. L. Pan, CsB4O6F: A Congruent-Melting Deep-Ultraviolet Nonlinear Optical Material by Combining Superior Functional Units, Angew. Chem., Int. Ed., 2017, 56, 14119–14123 CrossRef CAS PubMed.
- J. Lu, J. N. Yue, L. Xiong, W. K. Zhang, L. Chen and L. M. Wu, Uniform Alignment of Non-π-Conjugated Species Enhances Deep Ultraviolet Optical Nonlinearity, J. Am. Chem. Soc., 2020, 141, 8093–8097 CrossRef PubMed.
- S. G. Jantz, M. Dialer, L. Bayarjargal, B. Winkler, L. van Wüllen, F. Pielnhofer, J. Brgoch, R. Weihrich and H. A. Höppe, Sn[B2O3F2]—The First Tin Fluorooxoborate as Possible NLO Material, Adv. Opt. Mater., 2018, 6, 10–17 Search PubMed.
- X. L. Chen, B. B. Zhang, F. F. Zhang, Y. Wang, M. Zhang, Z. H. Yang, K. R. Poeppelmeier and S. L. Pan, Designing an Excellent Deep-Ultraviolet Birefringent Material for Light Polarization, J. Am. Chem. Soc., 2018, 140, 16311–16319 CrossRef CAS PubMed.
- G. H. Zou, L. Huang, N. Ye, C. Lin, W. Cheng and H. Huang, CsPbCO3F: A Strong Second-Harmonic Generation Material Derived from Enhancement via p–π Interaction, J. Am. Chem. Soc., 2013, 135, 18560–18566 CrossRef CAS PubMed.
- J. Kee and K. M. Ok, Hydrogen-Bond-Driven Synergistically Enhanced Hyperpolarizability: Chiral Coordination Polymers with Nonpolar Structures Exhibiting Unusually Strong Second-Harmonic Generation, Angew. Chem., Int. Ed., 2021, 60, 20656–20660 CrossRef CAS PubMed.
- I. Gautier-Luneau, Y. Suffren, H. Jamet and J. Pilmé, Reinterpretation of Three Crystal Structures of Alkali Oxoiodate(V) - Description of the [I3O8]− anion and the infinite 2D [I3O8−]∞ Anion, Z. Anorg. Allg. Chem., 2010, 636, 1368–1379 CrossRef CAS.
- X. Xu, C. L. Hu, B. X. Li, B. P. Yang and J. G. Mao,
α-AgI3O8 and β-AgI3O8 with Large SHG Responses: Polymerization of IO3 Groups into the I3O8 Polyiodate Anion, Chem. Mater., 2014, 26, 3219–3230 CrossRef CAS.
- D. Phanon and I. Gautier-Luneau, Promising Material for Infrared Nonlinear Optics: NaI3O8 Salt Containing an Octaoxotriiodate(V) Anion Formed from Condensation of [IO3]− Ions, Angew. Chem., Int. Ed., 2007, 46, 8488–8491 CrossRef CAS PubMed.
- H. Y. Chang, S. H. Kim, K. M. Ok and P. S. Halasyamani, Polar or nonpolar? A+ cation polarity control in A2Ti(IO3)6 (A = Li, Na, K, Rb, Cs, Tl), J. Am. Chem. Soc., 2009, 131, 6865–6873 CrossRef CAS PubMed.
- K. M. Ok and P. S. Halasyamani, The Lone-Pair Cation I5+ in a Hexagonal Tungsten Oxide-Like Framework: Synthesis, Structure, and Second-Harmonic Generating Properties of Cs2I4O11, Angew. Chem., Int. Ed., 2004, 43, 5489–5491 CrossRef CAS PubMed.
- K. M. Ok and P. S. Halasyamani, New Metal Iodates: Syntheses, Structures, and Characterizations of Noncentrosymmetric La(IO3)3 and NaYI4O12 and Centrosymmetric β-Cs2I4O11 and Rb2I6O15(OH)2·H2O, Inorg. Chem., 2005, 44, 9353–9359 CrossRef CAS PubMed.
- S. D. Nguyen, J. Yeon, S. H. Kim and P. S. Halasyamani, BiO(IO3): A New Polar Iodate that Exhibits an Aurivillius-Type (Bi2O2)2+ Layer and a Large SHG Response, J. Am. Chem. Soc., 2011, 133, 12422–12425 CrossRef CAS PubMed.
- Z. B. Cao, Y. C. Yue, J. Y. Yao, Z. S. Lin, R. He and Z. G. Hu, Bi2(IO4)(IO3)3: A New Potential Infrared Nonlinear Optical Material Containing [IO4]3− Anion, Inorg. Chem., 2011, 50, 12818–12822 CrossRef CAS PubMed.
- R. E. Sykora, K. M. Ok, P. S. Halasyamani, D. M. Wells and T. E. Albrecht-Schmitt, New One-Dimensional Vanadyl Iodates: Hydrothermal Preparation, Structures, and NLO Properties of A[VO2(IO3)2] (A = K, Rb) and A[(VO)2(IO3)3O2] (A = NH4, Rb, Cs), Chem. Mater., 2002, 14, 2741–2749 CrossRef CAS.
- C. F. Sun, C. L. Hu, X. Xu, B. P. Yang and J. G. Mao, Explorations of New Second-Order Nonlinear Optical Materials in the Potassium Vanadyl Iodate System, J. Am. Chem. Soc., 2011, 133, 5561–5572 CrossRef CAS PubMed.
- C. Wu, X. X. Jiang, Z. J. Wang, L. Lin, Z. S. Lin, Z. P. Huang, X. F. Long, M. G. Humphrey and C. Zhang, Giant Optical Anisotropy in the UV-Transparent 2D Nonlinear Optical Material Sc(IO3)2(NO3), Angew. Chem., Int. Ed., 2021, 60, 3464–3468 CrossRef CAS PubMed.
- X. H. Dong, L. Huang, H. M. Zeng, Z. E. Lin, K. M. Ok and G. H. Zou, High-Performance Sulfate Optical Materials Exhibiting Giant Second Harmonic Generation and Large Birefringence, Angew. Chem., Int. Ed., 2022, 61, e202116790 CAS.
- A. Tudi, S. J. Han, Z. H. Yang and S. L. Pan, Potential optical functional crystals with large birefringence: Recent advances and future prospects, Coord. Chem. Rev., 2022, 459, 214380 CrossRef CAS.
- J. Chen, C. L. Hu, F. F. Mao, J. H. Feng and J. G. Mao, A Facile Route to Nonlinear Optical Materials: Three-Site Aliovalent Substitution Involving One Cation and Two Anions, Angew. Chem., Int. Ed., 2019, 58, 2098–2102 CrossRef CAS PubMed.
- J. Chen, C. L. Hu and J. G. Mao, LiGaF2(IO3)2: A mixed-metal gallium iodate-fluoride with large birefringence and wide band gap, Sci. China Mater., 2021, 64, 400–407 CrossRef CAS.
- X. Q. Jiang, H. P. Wu, H. W. Yu, Z. G. Hu, J. Y. Wang and Y. C. Wu, Synthesis, Structure, Characterization, and Calculation of a Noncentrosymmetric Fluorine-Containing Indium Iodate, Ba[InF3(IO3)2], Cryst. Growth Des., 2021, 21, 4005–4012 CrossRef CAS.
- Y. Li, J. B. Huang and H. M. Chen, Crystal growth, characterization and theoretical studies of the noncentrosymmetric compound Al3(IO3)9·(HIO3)6·18H2O, J. Alloys Compd., 2021, 856, 157852 CrossRef CAS.
- W. B. Cai, J. Q. Chen, S. L. Pan and Z. H. Yang, Enhancement of Band Gap and Birefringence Induced via π-Conjugated Chromophore with “Tail Effect”, Inorg. Chem. Front., 2022, 9, 1224–1232 RSC.
-
Version 7 Saint, 60A Bruker Analytical X-Ray Instruments, Inc., Madison, WI, 2008 Search PubMed.
-
Bruker Suite, Bruker AXS Inc., Madison, USA, 2008.
- O. V. Dolomanov, L. J. Bourhis, R. J. Gildea, J. A.-K. Howard and H. Puschmann, OLEX2: A complete structure solution, refinement and analysis program, J. Appl. Crystallogr., 2009, 42, 339–341 CrossRef CAS.
- A. L. Spek, Structure validation in chemical crystallography, Acta Crystallogr., Sect. D: Biol. Crystallogr., 2009, 65, 148–155 CrossRef CAS PubMed.
- J. Tauc, Absorption edge and internal electric fields in amorphous semiconductors, Mater. Res. Bull., 1970, 5, 721–729 CrossRef CAS.
- S. J. Clark, M. D. Segall, C. J. Pickard, P. J. Hasnip, M. I.-J. Probert, K. Refson and M. C. Payne, First principles methods using CASTEP, Z. Kristallogr., 2005, 220, 567–570 CAS.
- J. P. Perdew, K. Burke and M. Ernzerhof, Generalized gradient approximation made simple, Phys. Rev. Lett., 1996, 77, 3865–3868 CrossRef CAS PubMed.
- K. Liu, H. Fan, P. Ren and C. Yang, Structural, electronic and optical properties of BiFeO3 studied by first-principles, J. Alloys Compd., 2011, 509, 1901–1905 CrossRef CAS.
- W. Wang, H. Fan and Y. Ye, Effect of electric field on the structure and piezoelectric properties of poly(vinylidene fluoride) studied by density functional theory, Polymer, 2010, 51, 3575–3581 CrossRef CAS.
- M. Mutailipu, M. Zhang, H. P. Wu, Z. H. Yang, Y. H. Shen, J. L. Sun and S. L. Pan, Ba3Mg3(BO3)3F3 polymorphs with reversible phase transition and high performances as ultraviolet nonlinear optical materials, Nat. Commun., 2018, 9, 1–10 CrossRef CAS PubMed.
- C. K. Huang and P. F. Kerr, Infrared study of the carbonate minerals, Am. Mineral., 1960, 45, 311–324 CAS.
- T. T. Tran, J. He, J. M. Rondinelli and P. S. Halasyamani, RbMgCO3F: A New Beryllium-Free Deep-Ultraviolet Nonlinear Optical Material, J. Am. Chem. Soc., 2015, 137, 10504–10507 CrossRef CAS PubMed.
- M. Q. Gai, Y. Wang, T. H. Tong, Z. H. Yang and S. L. Pan, ZnIO3F: Zinc Iodate Fluoride with Large Birefringence and Wide Band Gap, Inorg. Chem., 2020, 59, 4172–4175 CrossRef CAS PubMed.
- Q. M. Huang, C. L. Hu, B. P. Yang, Z. Fang, Y. Huang and J. G. Mao, Ba2[FeF4(IO3)2]IO3: a promising nonlinear optical material achieved by chemical-tailoring-induced structure evolution, Chem. Commun., 2021, 57, 11525–11528 RSC.
- T. H. Wu, X. X. Jiang, C. Wu, H. Y. Sha, Z. J. Wang, Z. S. Lin, Z. P. Huang, X. F. Long, M. G. Humphrey and C. Zhang, From Ce(IO3)4 to CeF2(IO3)2: fluorinated homovalent substitution simultaneously enhances SHG response and bandgap for mid-infrared nonlinear optics, J. Mater. Chem. C, 2021, 9, 8987–8993 RSC.
- L. J. Sham and M. Schlüter, Density-Functional Theory of the Energy Gap, Phys. Rev. Lett., 1983, 51, 1888–1891 CrossRef.
- A. J. Cohen, P. Mori-Sánchez and W. Yang, Fractional charge perspective on the band gap in density-functional theory, Phys. Rev. B: Condens. Matter Mater. Phys., 2008, 77, 115–123 CrossRef.
- Y. W. An, Y. Zhong, T. Q. Sun, H. J. Wang, Z. P. Hu, H. D. Liu, S. G. Liu, Y. F. Kong and J. J. Xu, Synthesis, structure and characterization of M(IO3)2(HIO3) (M = Ca, Sr) as new anhydrous alkaline earth metal bis-iodate hydrogeniodate compounds, Dalton Trans., 2019, 48, 13074–13080 RSC.
- J. Chen, C. L. Hu, F. F. Mao, B. P. Yang, X. H. Zhang and J. G. Mao, REI5O14 (RE = Y and Gd): Promising SHG Materials Featuring the Semicircle-Shaped I5O143− Polyiodate Anion, Angew. Chem., Int. Ed., 2019, 58, 11666–11669 CrossRef CAS PubMed.
- D. E. Zelmon, D. L. Small and D. Jundt, Infrared corrected Sellmeier coefficients for congruently grown lithium niobate and 5 mol% magnesium oxide–doped lithium niobate, J. Opt. Soc. Am. B, 1997, 14, 3319–3322 CrossRef CAS.
- G. Ghosh, Dispersion-equation coefficients for the refractive index and birefringence of calcite and quartz crystals, Opt. Commun., 1999, 163, 95–102 CrossRef CAS.
- G. Q. Zhou, J. Xu, X. D. Chen, H. Y. Zhong, S. T. Wang, K. Xu, P. Z. Deng and F. X. Gan, Growth and spectrum of a novel birefringent α-BaB2O4 crystal, J. Cryst. Growth, 1998, 191, 517–519 CrossRef CAS.
Footnote |
† Electronic supplementary information (ESI) available: Crystallographic data, IR spectrum, TGA diagram, band structure, and electron density difference map of Mg(H2O)6[(IO2(OH))2(IO3)]2. CCDC 2167789. For ESI and crystallographic data in CIF or other electronic format see DOI: https://doi.org/10.1039/d2tc01638a |
|
This journal is © The Royal Society of Chemistry 2022 |
Click here to see how this site uses Cookies. View our privacy policy here.