DOI:
10.1039/D3TA01581E
(Paper)
J. Mater. Chem. A, 2023,
11, 12266-12281
Layered double hydroxide/boron nitride nanocomposite membranes for efficient separation and photodegradation of water-soluble dyes†
Received
15th March 2023
, Accepted 24th May 2023
First published on 24th May 2023
Abstract
Widespread access to clean water is becoming increasingly scarce due to various factors, including increasing urbanisation and industrialisation, biodiversity loss, and climate change. The development of innovative and sustainable methods of water purification are urgently required. Herein, we report the development of new, high-performance nanofiltration (NF) membranes based on a recyclable CuAl–CO3 LDH/BNOx nanocomposite. These membranes have demonstrated up to 100% retention of Evans blue, methyl orange, methylene blue and rhodamine B, and exceptional water flux. Furthermore, the presence of CuAl–CO3 LDH induces up to 91% photodegradation of these dyes under visible light conditions in 120 minutes using a household LED lamp, acting as a potential low-cost, viable solution to membrane fouling. Importantly, this is the first report of Evans blue degradation by a visible-light active LDH photocatalyst. We believe that this work represents a significant step forward in advanced nanofiltration technology.
1 Introduction
According to a report published by UNICEF and the WHO in 2019, one third of the world's population does not have access to clean, safe drinking water.1 Dyes, surfactants and heavy metals leached into wastewater from the textile industry are a leading cause of water pollution.2 The release of these dyes into wastewater streams can have numerous detrimental effects to humans, as well as aquatic flora and fauna. This dye pollution has a significant negative impact on biodiversity in our waterways. The high absorption coefficients of these dyes in the visible light region hinders photosynthesis, as light cannot penetrate the water efficiently.3 Additionally, many of the dyes used industrially can be carcinogenic and mutagenic, causing long-term damage when ingested by aquatic life, as well as by humans.4 Azo dyes such as Evans blue are a major concern, with up to 50% of the dye used in the dyeing process being released into wastewater, due to inadequate binding to textiles.5,6 The toxicity of thiazine and triarylmethane dyes also presents significant risk.7,8 Innovative methods of water filtration and treatment are therefore urgently needed in order to achieve United Nations Sustainable Development Goal 6 – which is access to clean water for all by 2030. Nanofiltration (NF) is increasingly being explored as an efficient method for water purification.9,10 NF is typically described as a method of filtration that is capable of rejection of molecules with molecular weights as small as 200 daltons. However, many existing NF membranes suffer from significant, rapid fouling, restricting their lifetime significantly.11 To contribute to and develop a greener, more circular economy and society, the methods we use for water treatment must be sustainable. Reusable, recyclable NF membranes have the opportunity to be game-changers in this area, and significant research has been conducted in recent years on approaches to anti-fouling and fouling-resistant NF membranes.12–14
2D nanomaterials, such as boron nitride, graphene and transition metal dichalcogenides are increasingly being explored as exciting candidates for new NF membranes.15–18 Exceptional adsorption capacities can be achieved by 2D material-based membranes, due to their high surface-to-volume ratios, allowing more surface area for interaction with contaminants.19 The stacking of nanosheets when forming membranes results in the formation of nano-porous channels, often allowing for more rapid solvent transport and higher selectivity.20 Our previous work demonstrated the potential of partially oxidised boron nitride (BNOx) as a nanomaterial for high-performance NF membranes.21 BNOx can be produced by partial hydroxylation of the edges of BN sheets, which can be achieved by simple thermal oxidation, endowing BNOx with a partially negative surface charge.22 The introduction of these OH groups has been demonstrated to have no reduction in the NF performance of the exfoliated material when compared with pristine BN. However, the major drawback of these membranes at present is that they are vulnerable to fouling, and must be disposed of after a single use.
Layered double hydroxides (LDHs), often referred to as hydrotalcite-like materials or anionic clays, are layered ionic materials consisting of positively charged metal hydroxide sheets, intercalated with negatively charged counterions. The positive charge in the sheets originates from the doping of divalent M2+ species such as Cu2+, Ni2+, Mg2+, and Zn2+ with trivalent M3+ species such as Al3+, Fe3+ and Cr3+ in the M(OH)2 matrix, resulting in a partial positive charge.23 The general formula for a LDH is given by:
[M1−x2+Mx3+(OH)2]x+[An−]x/n·mH2O |
where A
n− represents the intercalating, charge balancing counter-anion, and
x is the molar fraction of trivalent cations in the material. Typically, an LDH is successfully synthesised when 0.2 ≤
x ≤ 0.4. A wide variety of anions can occupy the interlayer space – the most typical intercalating species being carbonate, nitrate, sulfate, and halide ions.
In recent years, there has been a resurgence in the study of LDHs, owed in part to their semiconductor nature.24 The use of LDHs can be advantageous over other traditional semiconducting nanomaterials, due to high scalability, low cost and ease of synthesis, as well as high bandgap tunability through variation of the metal cations, as well as the intercalating species.25 Due to these interesting and unique properties, many recent reports have examined the applications of LDHs in heterogeneous catalysis, specifically as electrocatalysts and photocatalysts.26–28 In particular, numerous reports of the design of transition-metal based LDHs for photodegradation of dyes in water remediation applications have emerged in recent years.29,30 Photocatalytically active nanomaterials may represent an innovative route to achieve anti-fouling behaviour in NF membranes. Despite this, to the best of our knowledge, no reports exist of exploiting the photocatalytic activity of LDHs for use as anti-fouling additives in NF membranes.
Herein, we present the development of new, high-performance hybrid CuAl–CO3 LDH/BNOx NF membranes for efficient separation and visible-light-driven photocatalytic degradation of several dyes – Evans blue, methyl orange, methylene blue and rhodamine B. We believe this innovative route to anti-fouling represents a significant step forward in advanced sustainable nanofiltration research.
2 Experimental
2.1. Starting materials
Hexagonal boron nitride (h-BN) powder was purchased from Merck (particle size = 6–30 mm). Evans blue (EB, ≥75%), methylene blue (MB, ≥82%), rhodamine B base (RhB, 97%), terephthalic acid (TA, 98%) and PERDROGEN™ 30% H2O2 (w/w) were purchased from Sigma-Aldrich. Methyl orange (MO, ≥95%) was purchased from VWR International Ltd. Copper nitrate trihydrate (Cu(NO3)2·3H2O, puriss, p.a. 99–104%) and aluminium nitrate nonahydrate (Al(NO3)3·9H2O, ≥98%) were purchased from Honeywell Fluka. Sodium carbonate (Na2CO3 99.5%) was purchased from Acros Organics. Sodium hydroxide (NaOH, general purpose grade) was purchased from Fisher Chemical. Millipore Water (MP H2O) was obtained using an in-house Milli-Q system with resin filters in Trinity College Laboratories. Durapore membrane filter templates (hydrophilic polyvinylidene fluoride, PVDF, with 0.45 μm pore size and 47 mm diameter) were purchased from Merck Millipore Limited. The sonic bath used was Ultrawave model U100H from Ultrawave Ltd. Super premium microscope slides were purchased from VWR. Millipore All-Glass Filter Holder Kit for vacuum filtration was purchased from Merck.
2.2. Instrumentation and characterisation
UV-vis absorption spectra were recorded using an Agilent Cary60 UV-visible spectrophotometer. All measurements were conducted using MP H2O as solvent. Solid state diffuse reflectance (DR) UV-vis-NIR absorption spectra were recorded using a PerkinElmer Lambda 1050 UV-vis-NIR spectrophotometer with 150 mm InGaAs integrating sphere. Samples were prepared for diffuse reflectance (DR) UV-vis by drop-casting some of the colloidal suspension onto a VWR super premium microscope slide cut to size, followed by solvent evaporation using a hot plate at 100 °C. Zeta potential measurements were carried out using a Malvern Panalytical Zetasizer and disposable folded capillary zeta cells. Fourier transform infrared (FT-IR) spectra were recorded using a PerkinElmer Spectrum 100 FT-IR with diamond UATR accessory. Powder X-ray diffraction (pXRD) was carried out using a zero-background holder and a Bruker D2 Phaser (second gen). Measurements were performed using Cu Kα radiation, and a range of 2θ = 5–80°, with fluorescence correction. Scanning electron microscopy (SEM) images were obtained using a Zeiss Ultra Plus Scanning Electron Microscope. Samples were prepared for SEM by adhering a portion of the membranes to carbon tab-covered cross-section SEM stubs. Mercury porosimetry was performed using an Autoscan-33 Porosimeter (Quantachrome, Hampshire, UK) with a default contact angle of 140°. Samples were prepared for transmission electron microscopy (TEM) via drop-casting of dispersed material in water on a 400-mesh lacey carbon Cu TEM grid followed by drying in vacuum overnight. TEM images were obtained using an uncorrected FEI Titan (scanning) transmission electron microscope (accelerating voltage = 300 kV). Spectrum imaging was carried out on the FEI Titan (beam current = approx. 500 pA) using a Bruker Quantax XFlash 6T-30 30 mm2 energy dispersive X-ray spectroscopy (EDX) detector and a Gatan Tridium GIF system (for electron energy loss spectroscopy (EELS)). Photoluminescence (PL) emission measurements were performed using a Horiba Jobin Yvon Fluoromax-4 and 10 mm quartz cuvette with 200 nm cut-off. Fluorescence emission spectra were recorded using excitation and emission slits of 2.5 nm, 1 nm increments, and an integration time of 0.1 s.
2.3. Preparation of bulk BNOx
This method was carried out according to our previous report.21 Bulk h-BN powder (5 g) was partially oxidised in a furnace in air, using a ramp rate of 5 °C, a target temperature of 1000 °C, and was held at the target temperature for 30 min to obtain bulk BNOx powder.
2.4. CuAl–CO3 LDH synthesis
The synthesis was carried out as described in previous work published by our group, adapted from the synthesis by Berner et al.31,32 Briefly, a co-precipitation was carried out by the dropwise addition of Cu(NO3)2·3H2O (1.35 g, 5.58 mmol) and Al(NO3)3·9H2O (2.1 g, 5.58 mmol) in 100 mL of MP H2O to sodium carbonate (0.81 g, 7.5 mmol) in 200 mL of MP H2O.
Drops of 1 M NaOH were added as required to maintain a pH of around 10. The solution was stirred for 4 h and aged overnight at room temperature (approx. 18–25 °C). The resulting material was cleaned via centrifugation (5500 rpm, ×3 MP H2O) and dried in an oven at 80 °C and ground to obtain a powder.
2.5. LDH/BNOx nanocomposite and membrane preparation
As-prepared CuAl–CO3 LDH (60 mg) and BNOx (240 mg) were added to a round-bottomed flask with 100 mL of MP H2O and sonicated for 24 h to obtain the LDH/BNOx nanocomposite. Membranes were prepared by taking 50 mL of the suspension and passing it through a PVDF template (pore size = 0.45 μm, diameter = 47 mm) by vacuum filtration, as described in our previous work.21
2.6. Dye retention and water permeance performance testing
Four water soluble dyes were used for testing the retention of the LDH/BNOx nanocomposite-based NF membranes – Evans blue (EB), methyl orange (MO), methylene blue (MB) and rhodamine B (RhB). Aqueous solutions of each dye were prepared to have a UV-vis absorbance at λmax of 1–1.5 a.u., as shown in Table 1.
Table 1 Concentrations and maximum absorbances of the four dyes used for testing membrane NF performance
Dye |
λ
max (nm) |
Abs. (a.u.) |
Conc. (μM) |
Evans blue |
608 |
1.06 |
15 |
Methyl orange |
460 |
1.50 |
50 |
Methylene blue |
664 |
1.18 |
27 |
Rhodamine B |
558 |
1.49 |
20 |
20 mL of the dye solution was passed through the membrane using vacuum filtration operating at a pressure of 1 bar. A UV-vis absorption spectrum of both the feed and the permeate were acquired. The permeate was further concentrated to obtain a 3 mL aliquot using rotary evaporation and re-dissolution to ensure a higher level of accuracy in the calculations, and a UV-vis absorbance spectrum of the concentrated permeate was also obtained. Eqn (1), which is commonly used for NF, and as described in our previous work, was used to calculate the retention:
| 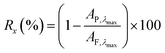 | (1) |
where
Rx is the retention in percentage,
AF,λmax is the absorbance at
λmax of the analyte in the feed, and
AP,λmax is the absorbance at
λmax of the analyte in the permeate.
Water permeance measurements in our dead-end filtration system were carried out both for the PVDF template, as well as the LDH/BNOx membrane according to our previous work, using 20 mL of MP H2O and an operating pressure of 1 bar. Flux was calculated according to eqn (2), as carried out in our previous work21
|  | (2) |
where
F is the flux in L m
−2 h
−1,
V is the volume of H
2O in L,
A is the working area in m
2, and
t is the time taken for the analyte to pass through the membrane (in h). The working area of the fritted glass filtration system is 9.6 × 10
−4 m
2.
2.7. Adsorption tests
An as-prepared LDH/BNOx membrane was cut into two, with one half being placed in a clean, polycarbonate Petri dish. 10 mL of the dye solution was added to the Petri dish, and the Petri dish was closed and placed in the dark. Regular aliquots were taken over the course of 6 up to hours for UV-vis analysis and returned to the Petri dish in a timely manner to minimise the light exposure time. The adsorption was modelled using pseudo-second order (PSO) and intra-particle diffusion (IPD) kinetic models, as shown in eqn (3) and (4), respectively. | 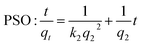 | (3) |
| IPD: qt = kIPD,it0.5 + ci | (4) |
where qt is the amount adsorbed at time t (in mg g−1), q2 is the maximum adsorption capacity for the PSO model, k2 is the apparent PSO rate constant, kIPD,i is the apparent IPD rate constant for a stage i in the IPD process, and ci is the intercept of the plot of qtvs. t0.5 and corresponds to the thickness of the boundary layer. The second half of the membrane was used for the photocatalysis experiments, as described in Section 2.8.
2.8. Photocatalytic degradation testing
An as-prepared LDH/BNOx membrane was cut into two, with one half being placed in a clean, polycarbonate Petri dish. 10 mL of the dye solution was added to the Petri dish, along with 10 μL of 30% w/w H2O2 solution. The open Petri dish was placed under a Parkside (Lidl) 5 W COB LED lamp operating at maximum power for 120 min. Aliquots were taken every 10 min over the course of 120 min for UV-vis analysis and returned to the Petri dish in a timely manner to maximise the light exposure time. The photocatalytic degradation of each dye by the LDH/BNOx membranes was performed in triplicate. The photodegradation process was modelled using pseudo-first order (PFO) degradation kinetics, as described in eqn (5). | 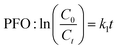 | (5) |
where C0 is the initial dye concentration, Ct is the concentration at time t, and k1 is the apparent PFO rate constant.
2.9. H2O2 controls in absence of catalyst
The experiment was performed as described in Section 2.8, with no membrane added as a photocatalyst, to determine the extent of dye photobleaching with H2O2 in the presence of visible light.
2.10. Confirmation of hydroxyl radical (·OH) generation
The generation of ·OH radicals by the LDH/BNOx were monitored using the terephthalic acid (TA) probe technique, as reported by Page et al.33 4 round-bottomed flasks were filled with 10 mg of TA and 50 mL of 20 mM NaOH aqueous solution to aid with aqueous solubility. 30 mg of LDH/BNOx nanocomposite was added to each flask. One flask was placed under the LED lamp, and the second was placed in the dark. To the third and fourth flasks, 20 μL of H2O2 (30% (w/w)) was added. One of these was placed under the LED lamp, and the other was placed in the dark. The samples were left for three hours, after which the solids were removed by centrifugation at 15
000 rpm for 2 minutes. The supernatant was retained, and photoluminescence emission spectra of the fluorescent product, hydroxyterephthalic acid (hTA), were obtained using an excitation wavelength of 315 nm and an emission range of 350–500 nm.
3 Results and discussion
3.1. Characterisation of LDH/BNOx nanocomposite material and membranes
BNOx was chosen over BN to fabricate the NF membranes due to the negative surface charge which arises as a consequence of the introduction of –OH groups during the thermal oxidation process, as previously reported by our group.21 BNOx therefore has the ability to participate in attractive electrostatic interactions with LDHs, and should result in a uniform, electrostatically-stabilised nanocomposite. LDH nanosheets have an intrinsic positive surface charge, due to the doping of M3+ species in the M(OH)2 units.34 CuAl–CO3 LDH in particular was chosen for this study as it has previously been reported in literature to exhibit photodegradation of azo dyes such as MO, and presents less risk for water treatment than more photoactive Cr-containing LDHs, which can sometimes be prone to oxidative leaching of Cr6+.35 Zeta potential (ζ) measurements of as-prepared CuAl–CO3 LDH and exfoliated BNOx were carried out in aqueous solution (pH 7) to confirm the presence of these complementary surface charges, and average values of ζBNOx = −30.7 mV and ζLDH = +33.6 mV were obtained (Fig. S.1 and S.2†).
A number of different compositions were tested for the formation of the nanocomposite, the details of which are discussed in Section S.2 of the ESI.† An optimal composition of 20
:
80 LDH/BNOx by mass was determined, as this level of LDH loading should be theoretically high enough to induce photocatalysis, but low enough to maintain the integrity and high performance previously reported for BNOx-based NF membranes. The exfoliated LDH/BNOx nanocomposite was prepared by sonication for 24 h, and the membranes were prepared via the common vacuum filtration technique, as described in our previous reports.10,21 The resulting nanocomposite and membranes were characterised by pXRD, FT-IR, SEM, TEM, HAADF-STEM, EDX, EELS, mercury porosimetry and zeta potential. Fig. 1(a) shows a direct comparison between the pXRD patterns of the two components of the membrane, and the membrane itself. The most prominent reflections observed from the membrane sample are the CuAl–CO3 LDH (003) reflection at 2θ = 12.1°, the BN (002) reflection at 2θ = 26.8°, and the B(OH)3 reflection at 2θ = 28.0°.21,32 All observed pXRD reflections in the membrane sample can be indexed to the characteristic reflections of CuAl–CO3 LDH (JCPDS 46-0099), and BNOx (JCPDS 01-073-2095 for boron nitride, and JCPDS 30-0199 for boric acid (B(OH)3)) – with no formation of any undesirable side products.
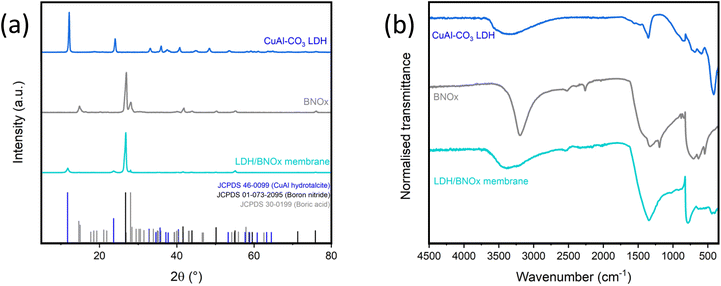 |
| Fig. 1 (a) pXRD patterns and (b) FT-IR spectra of CuAl–CO3 LDHs (blue), BNOx (grey) and LDH/BNOx nanocomposite (turquoise). pXRD reference patterns are provided for CuAl hydrotalcite, boron nitride and boric acid. | |
FT-IR also was performed on the sample, as shown in Fig. 1(b). The FT-IR spectrum of the membrane contains peaks characteristic of both BNOx and CuAl–CO3 LDH. The B–N stretch (750 cm−1) and B–N–B bend (1250–1500 cm−1) can be observed, with the B–N–B bend broadening and losing detail due to overlap with the CuAl–CO3 LDH v3 CO32− stretch (1350 cm−1). Additionally, there is a broad O–H stretch from roughly 3000–3600 cm−1 due to the overlap of the BNOx O–H band at 3000–3200 cm−1 and the CuAl–CO3 LDH O–H stretch centred at 3400 cm−1.22,36
Top-view and cross-sectional SEM imaging was conducted to determine the morphological profile of the membranes, as shown in Fig. 2. It is evident from SEM imaging that the membranes exhibit a very uniform, porous profile, with no obvious layering or separation of the two materials observed. Additional top-view and cross-section SEM images are shown in Fig. S.7 in Section S.3 of ESI.† These membranes have a remarkably similar morphological profile to previously reported BNOx membranes, with a calculated mean thickness of 121 ± 8 μm (Fig. S.8†).
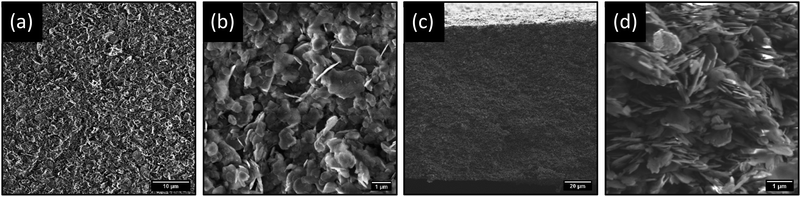 |
| Fig. 2 (a and b) Top-view and (c and d) cross-sectional SEM images of LDH/BNOx membranes. Lower magnification images (a and c) show general uniformity of membranes, and higher magnification (b and d) shows finer membrane structure consisting of networks of nanosheets. | |
From the SEM images, it is apparent that there is a uniform dispersion of larger and smaller nanosheets. Size distribution analysis of the lateral sizes of nanosheets in the membrane structure was performed, revealing a mean lateral size of 0.85 μm and a large standard deviation of 0.38 μm (Fig. S.9†). Furthermore, sheets sampled through size distribution analysis range widely in lateral size from 0.19 to 2.09 μm. This is indicative of a large, broad distribution consisting of smaller and larger flakes. Additionally, TEM imaging was performed on exfoliated BNOx, and suggests that the larger, irregular rounded flakes present in the membrane are representative of BNOx nanosheets, with an average lateral size of 0.95 ± 0.32 μm, and flake sizes ranging from 0.43 to 1.90 μm. (Fig. S.10 and S.11†). This indicates that a sizable population of smaller sheets in the membrane are representative of CuAl–CO3 LDH nanosheets, which is in line with expectations based on our previous reports.32 This broad particle size distribution is an expected consequence of using liquid phase exfoliation in water, without further post-processing steps for size selection.16,37 To further confirm the composition of the LDH/BNOx nanocomposite, HAADF-STEM was conducted in combination with EDX and EELS mapping, and confirms the presence of both individual CuAl–CO3 LDH and BNOx nanosheets in the nanocomposite, as shown in Fig. 3.
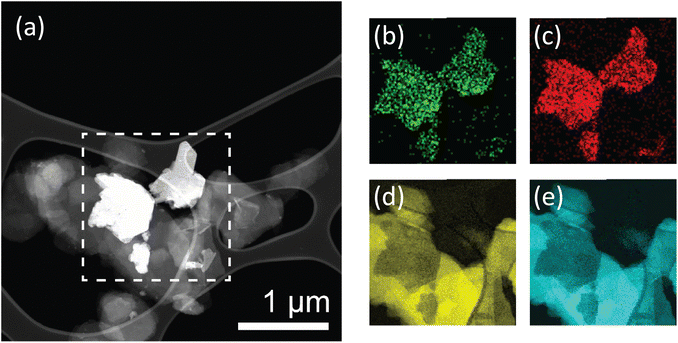 |
| Fig. 3 HAADF-STEM image (a) of LDH/BNOx nanocomposite. Dashed box in (a) is area under analysis in (b)–(e). Elemental mapping of Al (b) and Cu (c) via EDX, and N (d) and B (e) via EELS. Scale bar in (b)–(e) is same as in (a). | |
Mercury porosimetry was conducted on the LDH/BNOx membrane sample to investigate the porosity of the membranes, in the approximate 10 nm to 10 μm range, as shown in Fig. 4. Fig. 4(a) shows the intrusion of mercury into the sample as a function of pore diameter. At initial low pressures, the rate of intrusion is constant. As the pressure builds, mercury is forced into increasingly smaller pores, with an uptake occurring at the 4 μm mark. A gradual filling of pores then occurs to approximately 600 nm. At this point, a second distinct stage of filling is observed, almost linearly, to 150 nm. This region is significant, accounting for approximately 0.25 cm3 g−1 or 38.5% of the total pore volume (0.65 cm3 g−1). A third and final stage of filling occurs at 150 nm, with a steep rise in the curve due to rapid intrusion, before gradually plateauing out at 15 nm, with no further intrusion occurring as all pores are fully filled. The resultant pore size distribution, shown in Fig. 4(b), reveals that the vast majority of pores are <1 μm in size. Pores in the 10–200 nm range dominate the material, with a sharp peak at approximately 100 nm indicating a high concentration of pores within a narrow size range. The porosimetry data is in good agreement with the morphologies observed in the SEM images in Fig. 2, where porosity is observed between the flakes. Broadly speaking, there are strong similarities between the LDH/BNOx membranes developed here, and BN and BNOx-based membranes previously developed by our group.10,21 In particular, a BN membrane exfoliated in N-methyl-2-pyrrolidone exhibited a total pore volume of 0.76 cm3 g−1 and a sharp pore size distribution peak at approximately 100 nm. Like that membrane, we consider the LDH/BNOx membrane developed here to be a low bulk density material with an open, interconnected porous network.
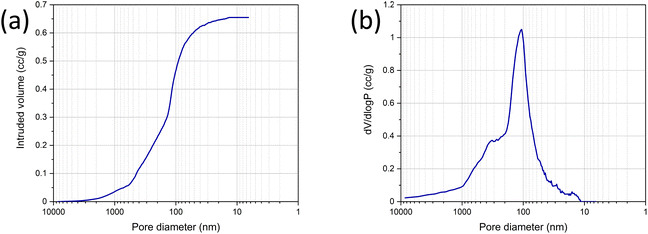 |
| Fig. 4 (a) Mercury intrusion and (b) pore size distribution of the LDH/BNOx membrane sample. | |
Thus, the combination of SEM, TEM, and mercury porosimetry confirms that the LDH nanosheets integrate well into the BNOx-based membranes, and therefore should not cause any major differences in NF performance.
3.2. Nanofiltration performance of LDH/BNOx nanocomposite membranes
Once it was confirmed that the LDH/BNOx nanocomposite could produce uniform membranes of similar morphology and pore characteristics to previously reported BN and BNOx membranes, these membranes were assessed for their ability to remove water-soluble dyes from water. Two azo dyes (EB and MO), a triarylmethane dye (RhB) and a thiazine dye (MB) were tested, to examine the ability of the membranes to retain dyes of various sizes, charges (anionic vs. cationic) and functional groups. The dye retention performance of the membranes was tested by passing 20 mL aliquots of the dye through the membranes, and examining the difference in absorption of the feed and filtrate solutions, as per previous reports.10,21 The UV-vis absorption spectra of feeds and samples of permeates of all four dyes are shown in Fig. 5. The absorbance values at λmax for each dye were recorded, and the retentions of each dye were calculated using eqn (1). The average retentions of each dye are shown in Table 2, along with the number of membranes evaluated in each case.
 |
| Fig. 5 UV-vis spectra demonstrating the retention of 20 mL of (a) 15 μM Evans blue, (b) 50 μM methyl orange, (c) 27 μM methylene blue, and (d) 20 μM rhodamine B by LDH/BNOx nanocomposite membranes. | |
Table 2 Mean retention values of LDH/BNOx membranes for a variety of dyes
Dye tested |
Mean retention (%) |
Number of membranes tested |
Evans blue (15 μM) |
99.7 ± 0.4 |
8 |
Methylene blue (27 μM) |
99.9 ± 0.1 |
5 |
Methyl orange (50 μM) |
96.8 ± 3.1 |
11 |
Rhodamine B (20 μM) |
97.4 ± 2.1 |
5 |
These retention values compare favourably with some of the best in literature for BN/BNOx-based membranes, as well as other 2D material-based membranes.38,39 For example, amino-functionalised h-BN membranes showed excellent retention for larger molecules such as EB (>99%), and much lower values for smaller molecules such as MB (50.3%) and RhB (58.3%). However, the retention can be greatly improved to 94.1% for MB and >99% for RhB by increasing the membrane thickness.18 GO membranes have been demonstrated to have dye rejection values of 98.4%, 98.9% and 86.4% for MO, MB and RhB respectively.40 WS2-based membranes exhibited retentions of 90% for EB.41 It is therefore evident that the LDH/BNOx membranes reported exhibit exceptional retention for larger dyes such as EB (dimensions: 1.2 nm × 3.1 nm), as well as smaller dyes such as MB (dimensions: 1.4 nm × 0.6 nm), and are in line with some of the best results reported in the literature.
The mechanism of dye removal from water by LDHs generally follows two schemes – incorporation of the dye into the LDH interlayer gallery via anion exchange, and dye adsorption to the nanosheet surface.42 Carbonate-intercalated LDHs typically are the most stable sub-type, as the divalent carbonate anion is held strongly in the LDH interlayer, so adsorption via anion exchange in these LDHs is less commonly observed.43 The dominant adsorption mechanism therefore is likely surface adsorption. LDHs have a positive surface charge, so tend to preferentially adsorb anionic species through electrostatic interactions.
Interestingly, no clear trend can be observed between the charge of the dye and the retention in this case. It can be observed that EB (an anionic dye) and MB (a cationic dye) both exhibit exceptional retention of up to 100%, with marginally lower values obtained for MO (anionic) and RhB (cationic). This serves as further evidence that the introduction of LDHs into BNOx-based NF membranes has no significant impact on the NF performance. Our previous reports on BNOx NF membranes indicates the partial negative surface charge may have a small effect on the retention compared to pristine BN, resulting in marginally higher retention of cationic dyes.21 However, the dominant retention mechanism observed is likely a result of the BNOx bonding and structure. Hexagonal BN is isostructural to graphene, with sp2 hybridised alternating B and N atoms in a hexagonal arrangement, resulting in electron delocalisation and a resultant π-system. It has been reported that a major factor in the high retention values and high adsorption capacities of BN and BNOx towards dye molecules is due to π–π interactions.44,45 It is therefore evident here that BNOx is the material responsible for the high retention values in the LDH/BNOx nanocomposite membranes.
Another key measure of membrane performance is the water flux or permeance. While high retention capacity is a vital property of high-performance water purification membranes, it must be ensured that this does not come at the cost of water transport across the membrane. In order to more accurately assess the suitability of our NF system for water purification, solvent flux studies were carried out on both the LDH/BNOx membranes, as well as the PVDF support (summary in Section S.5 of ESI, Tables S.2 and S.3.†), The hydrophilic PVDF template exhibited low resistance to water flow, as expected, with high flux values of 7360 ± 232 L m−2 h−1 at an operating pressure of 1 bar. The pure water permeance of the LDH/BNOx membranes on the PVDF support was measured to be 1626 ± 35 L m−2 h−1, which is slightly higher than previously reported BNOx membranes (1163 L m−2 h−1).21 The water flux is very high for a membrane of this thickness, when compared with the literature. For example, these membranes exhibit water permeance rates comparable with amino-functionalised h-BN membranes with 0.4 μm thickness (1500 L m−2 h−1) reported by Chen et al.18 Das et al. reported 0.35 μm-thick non-functionalised h-BN/PVDF membranes with flux rates of 1240 L m−2 h−1.46 Furthermore, the LDH/BNOx membranes reported here exhibit flow rates more than 230 times higher than commercially available NF270–400 membranes (7 L m−2 h−1 bar−1 or 23.8 L m−2 h−1 @ 3.4 bar).47 The highly efficient flow rate reported here can be attributed to the larger pore size observed for LDH/BNOx membranes compared to their pristine BNOx counterparts, as discussed previously in Section 3.1.
3.3. Adsorption behaviour of LDH/BNOx nanocomposite membranes
An analysis of the adsorption behaviour of the nanocomposite membranes was conducted to better understand the membrane retention mechanism. UV-vis spectra showing the adsorption of each of the dyes over time can be found in Section S.4 of the ESI (Fig. S.12).† Adsorption of dyes on many nanomaterials, including both BN and LDH, is often best described by the PSO kinetic model.48,49 Here, we observe two distinct adsorption behaviours in the case of these nanocomposite membranes, which are seemingly dependent on the charge of the dyes. The anionic dyes (EB and MO) follow an expected PSO adsorption model, as shown in Fig. 6.
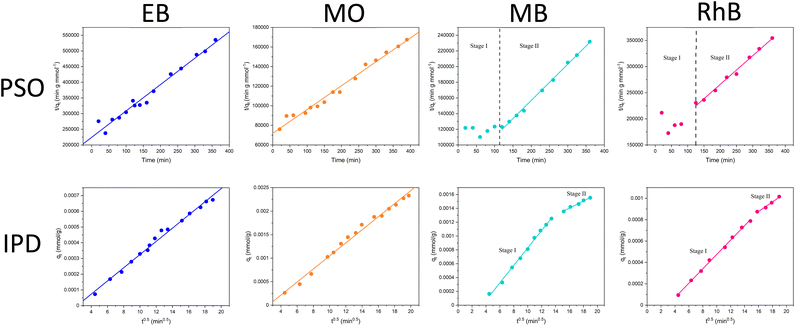 |
| Fig. 6 (Top) Pseudo-second order and (bottom) intra-particle diffusion kinetic models for adsorption of dyes on to LDH/BNOx nanocomposite membranes. | |
LDHs typically have a stronger adsorption capacity for anionic dyes due to attractive electrostatic interactions. However, here we observe the rate remaining constant over the experiment, whereas a change in rate would generally be expected as more LDH sites are occupied. This reaffirms the point that the π–π interactions between the BNOx and the dyes dominate over the electrostatic forces in the retention process, and serves as an explanation as to why there is no observable trend between the charge of the dyes and the retention of the membrane. However, for the cationic dyes, we see a deviation from simple PSO-type adsorption behaviour. The observed adsorption data can be split into two “stages” – stage I with fluctuating values and no clear correlation to the PSO model, and a stage II which fits to the PSO model. To gain further insight into this charge-dependent adsorption behaviour, the intra-particle diffusion (IPD) model was applied to the adsorption of all four dyes by the nanocomposite.50,51 The parameters obtained from the PSO and IPD fitting are shown in Table 3.
Table 3 PSO and IPD parameters from fitting models to dye adsorption on LDH/BNOx nanocomposites
Dye |
PSO kinetics |
IPD kinetics |
k
2 (g mmol−1 min−1) |
q
2 (mmol g−1) |
R
2
2
|
k
IPD,1 (mmol g−1 min−0.5) |
R
IPD,1
2
|
k
IPD,2
|
R
IPD,2
2
|
Evans blue |
3.156 |
0.0012 |
0.9748 |
4.193 × 10−5 |
0.9901 |
— |
— |
Methyl orange |
0.823 |
0.0041 |
0.9843 |
1.389 × 10−4 |
0.9909 |
— |
— |
Methylene blue |
3.339 |
0.0022 |
0.9972 |
1.262 × 10−4 |
0.9964 |
5.112 × 10−5 |
0.9880 |
Rhodamine B |
1.924 |
0.0018 |
0.9914 |
6.432 × 10−5 |
0.9979 |
4.448 × 10−5 |
0.9841 |
For anionic dyes (EB and MO), the data is observed to fit the linear intra-particle diffusion model, with high R2 values. According to the IPD model, if the straight line plot of qtvs. t0.5 does not pass through the origin, then IPD is not the rate limiting step.52,53 It can be observed for all dyes that none of the lines pass through the origin, indicating another adsorption process occurring in conjunction with IPD. This is likely the π–π interactions previously discussed, as well as electrostatic interactions between the dyes and the nanocomposite. However, for the cationic dyes (MB and RhB), a multi-linear adsorption profile can be observed. This is particularly evident in the case of MB. It is once again clear that two distinct adsorption processes occur, denoted stages I and II. Stage I is a sharper region and represents rapid surface adsorption. Stage II can be characterised by a smaller slope and the slower diffusion of the dyes into the pores of the membrane. We propose that this two-stage process arises for adsorption of cationic dyes due to the composition of the nanocomposites. Zeta potential measurements of the LDH/BNOx nanocomposite indicate a partial negative surface charge (ζLDH/BNOx = −8.12 mV) (Fig. S.3†). A larger proportion of partially negatively charged BNOx than LDH in the nanocomposite attracts the cationic dyes to the surface faster, followed by a slow diffusion into the pores and retention by π–π interactions, resulting in two distinct rates arising from the IPD model.
3.4. Photocatalytic degradation of dyes by LDH/BNOx nanocomposite membranes
The excellent retention values of these membranes are in line with our previous reports, as well as other reports for similar systems in literature, and present an efficient route to water purification. However, a main drawback of pristine BN and BNOx-based membranes is fouling. The adsorption of the aforementioned dyes by the membranes leads to saturation of the BNOx nanosheets, which in turn results in lower retention values over time, limiting their reusability. A potential method to overcome this is the integration of photocatalysts in these membranes. Transition metal-containing LDHs have been previously reported to be promising photocatalysts for a variety of reactions, including photodegradation of water soluble dyes.54–56 Therefore it was decided to exploit this property, and test the anti-fouling capabilities of these LDH-containing membranes using a simple, low-cost household visible light source. 10 μL of 30% w/w H2O2 was used to boost the catalytic activity, the reasons for which will be discussed in Section 3.5.57 The catalytic performance of the LDH/BNOx nanocomposite membranes for the degradation of EB, MB, MO and RhB was monitored using UV-visible spectroscopy. Fig. 7 shows the reduction in the maximum absorbances as a result of decolourisation of the solution due to degradation of each of the dyes. It can be observed that each of the dyes is degraded significantly over a 120 minute time frame, with the best result being achieved for MB. It is also important to highlight the significance of the result of degradation of EB by these nanocomposites, as reports of the visible-light photodegradation of EB are difficult to find. To the best of our knowledge, this is the first report of EB degradation using a LDH photocatalyst.
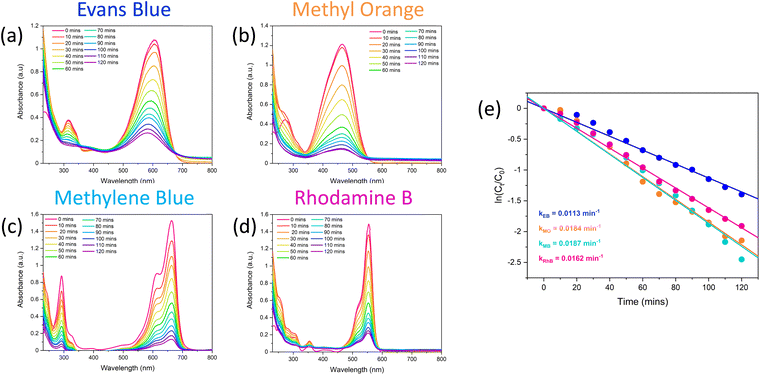 |
| Fig. 7 Reduction in UV-vis absorbance of (a) Evans blue, (b) methyl orange, (c) methylene blue and (d) rhodamine B by visible-light photodegradation in the presence of LDH/BNOx membrane catalysts and 10 μL of H2O2 (30% w/w). (e) PFO models for degradation of Evans blue, methyl orange, methylene blue and rhodamine B. | |
Images of membrane samples after filtration and after photodegradation are shown in Fig. 8. The membranes can be seen to return completely to their original pale-blue colour after the photodegradation process. The dye photodegradation process was modelled by PFO kinetics, as described in Section 2.8 (eqn (4)). The best results for each of the dyes are presented here, however each experiment was conducted in triplicate – the results of the other runs are shown in Section S.5 of ESI (Fig. S.13 and Table S.4†). The kinetics of organic dye degradation is often best described by the Langmuir–Hinshelwood model, which at low dye concentrations – as we have here – can be simplified to a PFO model.58 The apparent PFO rates for the best run of each dye are summarised in Fig. 7(e) and in Table 4. The PFO degradation rate constants obtained for the LDH/BNOx catalytic membrane compare favourably to similar systems reported in the literature. For example, a Co-based LDH synthesised in water, using 40 μL of H2O2 in the photodegradation process, had a calculated rate constant of 3.5 × 10−3 min−1 for degradation of RhB.59 Nayak et al. synthesised MgCr LDH nanoplatelets for sunlight-driven degradation of RhB in the absence of H2O2, and calculated the rate constant to be 0.015 min−1.60 Although their light harvesting capabilities are superior, it is preferred to avoid the use of Cr-containing LDHs in water treatment applications if possible, as leaching of Cr3+ and Cr6+ is a potential hazard depending on the conditions.35 Ahmed et al. reported PFO rate constants of 0.01 min−1 for visible-light degradation of MO by NiAl LDH photocatalysts.29 It is clear therefore that the LDH/BNOx membrane developed here is comparable with existing LDH-based photocatalysts for organic pollutant degradation.
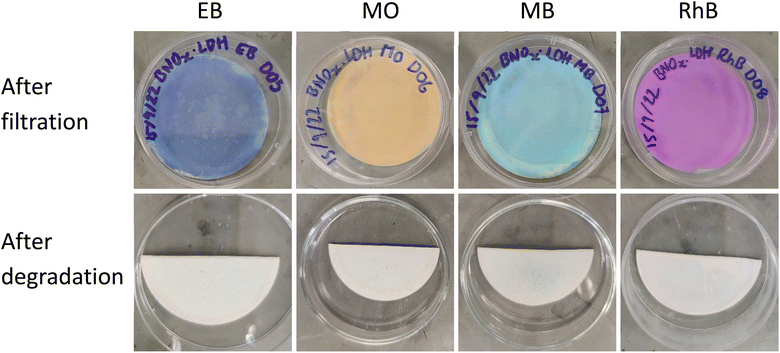 |
| Fig. 8 Pictures of membranes before (top) and after (bottom) photodegradation using 10 μL H2O2 (30% w/w) and a 5 W COB LED. | |
Table 4 Degradation extents at t = 120 min, and PFO model rate constants for degradation of dyes by LDH/BNOx nanocomposite membranes
Dye |
Highest degradation at 120 min (%) |
Highest k1 (min−1) |
R
1
2
|
Average k1 (min−1) |
Evans blue |
75 |
0.0113 |
0.9950 |
0.0091 ± 0.0019 |
Methyl orange |
88 |
0.0184 |
0.9949 |
0.0131 ± 0.0040 |
Methylene blue |
91 |
0.0187 |
0.9934 |
0.0174 ± 0.0021 |
Rhodamine B |
85 |
0.0162 |
0.9980 |
0.0151 ± 0.0008 |
Control experiments were conducted in the absence of catalyst, and showed insignificant extents of dye degradation in comparison with the photocatalytic nanocomposite membrane, and can be found in Section S.5 of ESI (Fig. S.14).† It is clear in the case of EB, MO and RhB that photolysis in the presence of H2O2 and the absence of catalyst is minimal. A non-negligible degree of photolysis was observed in the case of MB, where roughly 20% of the dye was degraded in the absence of catalyst in 120 minutes. However, this was to be expected, as aqueous solutions of methylene blue are prone to photobleaching in the presence of visible light.61
Nevertheless, the presence of the catalyst in this case clearly results in a more rapid degradation of the dye. While the removal of the dyes by photodegradation allows for the material to be reused, the membrane itself suffers from mechanical failure, due in part to the generation of gas bubbles under illumination (Fig. S.15†). SEM imaging of the membranes after photodegradation reveals exfoliation of the membranes, as well the generation of spherical cavities due to evolution of gases during the reaction (Fig. 9). Despite the excellent retention, flux, and catalytic activity of these membranes, it is evident that further research is needed to address the mechanical integrity and ensure the device itself is reusable – not just the material it consists of. Chemical cross-linking of the nanosheets within the membrane would be the ideal next step to address the issues with mechanical stability. Nevertheless, the efficient and rapid visible-light photodegradation of the dyes in these high-retention, high-flux membranes is an exciting and promising result in the search for efficient NF membranes that are resistant to fouling.
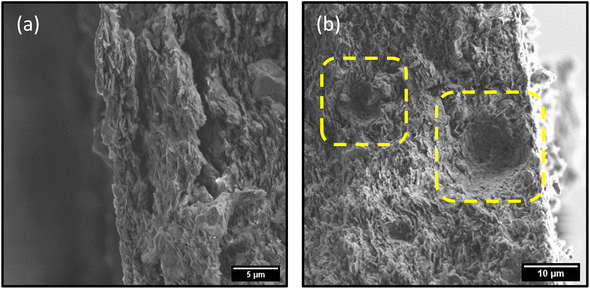 |
| Fig. 9 SEM images depicting (a) exfoliation of membrane and (b) formation of micro-bubble shaped cavities in membrane during photodegradation process. | |
3.5. Proposed photodegradation mechanism
Bulk h-BN is an indirect wide bandgap semiconductor, with reported bandgap values in the deep UV (∼6 eV).62 While an indirect-to-direct bandgap transition has been theorised on reducing the thickness of h-BN from few-layer to monolayer, the band-gap value remains large (6.46 eV). Photocatalysis has been observed in BN, however it is achieved through introduction of defects, and using UVC illumination.63 For these reasons, it is unlikely that there is any significant contribution from BNOx to the visible-light photocatalytic activity observed in this study. The source of photocatalytic degradation can be primarily assigned to the presence of CuAl–CO3 LDH in the nanocomposite in this case.
UV-vis absorption spectra of LDHs can provide key information on their suitability as visible light photocatalysts.25 Due to the intense light scattering exhibited by 2D nanosheets in solution, it is necessary to carry out the UV-vis in solid state using DR UV-vis to identify the key absorption features.64 The DR UV-vis spectrum of CuAl–CO3 LDHs reveals two main absorption features – the first centred around 475 nm, which can be attributed to O2− to Cu2+ ligand to metal charge transfer, and the second at 700 nm, associated with Cu2+ d–d transitions (Fig. S.16†).65,66 These significant absorption features in the visible region indicate the CuAl–CO3 LDH is a good candidate for visible-light photocatalysis.
As LDHs function as doped semiconductors, photocatalysis in LDHs is commonly accepted to be due to formation of electron–hole pairs as a result of light absorption. This behaviour has been well described by Baliarsingh et al., as well as Xia et al. for a variety of LDHs, and we propose a similar mechanism for our system.54,67 Photogenerated holes (h+) react with water in the aqueous dye solution to form hydroxyl radicals (·OH). The photoexcited electrons (e−) react with oxygen adsorbed on the LDH surface from dissolved oxygen in solution, forming superoxide radicals (O2˙−) (eqn (6) and (7)).
O2˙− radicals can be protonated to form hydroperoxyl radicals
H2O2 is generated by the formation and combination of
radicals. The reaction between photoexcited electrons and H2O2 produces more ·OH radicals which, along with O2˙−, are reactive oxygen species, resulting in degradation of the dyes (eqn (8)–(10)).54
| 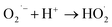 | (8) |
|  | (9) |
| H2O2 + e− → ·OH + OH− | (10) |
Apparent photocatalysis reaction rates in LDHs are limited by both electron–hole pair generation, as well as separation and carrier mobilities.55,68 CO32− intercalation in LDHs is reported to suppress the electron–hole recombination to an extent, thus increasing the apparent reaction rates, however high rates of degradation of dyes under visible-light illumination by pristine binary LDHs, particularly CuAl LDHs, is still rarely reported.54,69 To overcome this hurdle, small amounts of H2O2 were added to the solution, much less than previous reports for similar systems.70 The addition of H2O2 to Cu2+-based photocatalytic systems may improve the photocatalytic performance via two routes. Firstly, H2O2 acts as an electron acceptor, reducing the electron–hole recombination rate, thus enhancing the visible-light-driven generation of ·OH and O2˙−. Secondly, Cu2+-containing solids can also drive the catalytic decomposition of H2O2 to generate ·OH radicals via Fenton-like redox processes (eqn (11) and (12)).71,72
|  | (11) |
| Cu+ + H2O2 → Cu2+ + ·OH + OH− | (12) |
In this system, much lower rates of degradation are observed in the absence of H2O2. This was demonstrated using RhB in Section S.5 of ESI (Fig. S.17)† where 58% of RhB is seen to be removed from solution after 120 minutes, compared to 85% removal upon addition of H2O2. Furthermore, it is difficult to separate the contributions of adsorption and photodegradation processes in the absence of H2O2, as roughly 45% of RhB is adsorbed at that same point in time in dark conditions. To gain insights into the catalytic mechanism, the terephthalic acid (TA) probe technique was used to monitor the formation of ·OH radicals. TA is a highly sensitive ·OH radical probe, reacting to form a fluorescent product – hydroxyterephthalic acid (hTA), which can be monitored using photoluminescence spectroscopy (PL).33,73 Under excitation with 315 nm light, hTA has a characteristic emission band centred around 420 nm, and the intensity of this emission band while used in this context in photocatalytic systems is representative of more efficient generation of ·OH radicals. PL emission spectra were recorded for four different environments – TA + LDH/BNOx + dark, TA + LDH/BNOx + dark + H2O2, TA + LDH/BNOx + light, and TA + LDH/BNOx + light + H2O2, as shown in Fig. 10.
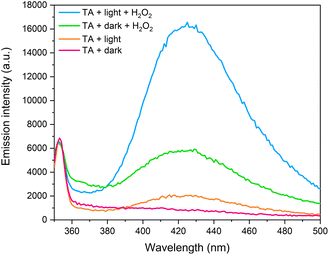 |
| Fig. 10 Photoluminescence emission spectra of hydroxyterephthalic acid generated using LDH/BNOx catalyst. | |
It can be observed that in the absence of light and H2O2, there is no measurable emission that is characteristic of the presence of hTA, meaning there is minimal ·OH radical generation in these conditions. Upon addition of H2O2, the appearance of a significant emission peak can be observed in dark conditions, confirming that the LDH/BNOx acts as a Fenton-type catalyst. Exposure to light in the absence of H2O2 results in the generation of a low intensity emission peak, which is to be expected, as generation of ·OH radicals by LDHs is limited by electron–hole recombination. The most intense signal is observed when the LDH/BNOx catalyst is exposed to light in the presence of H2O2, resulting in the formation of more ·OH radicals through both Fenton-type reactions, as well as from photoexcited electrons and photogenerated holes. Based on these experimental observations, we propose that the catalytic mechanism is a synergistic process, with contributions from Cu2+-based photo-Fenton-type chemistry, as well as the semiconducting behaviour of CuAl–CO3 LDHs.
·OH radicals are expected to initiate degradation of these dyes by disrupting the chromophores, via azo bond cleavage in EB and MO, and ring opening mechanisms in the case of RhB and MB. UV-vis provides indirect evidence of this proposal in the case of EB – a diazo dye – as a decrease in UV-vis absorbance intensity is accompanied by a significant blue shift λmax from 608 nm to 580 nm (Fig. 7(a)). This is indicative of a reduction in the extent of conjugation due to reduction and subsequent cleavage of the azo bond.74 Tentative proposed mechanisms of the initial steps of degradation of each of the dyes are shown in Fig. 11, based on experimental observations for EB, as well as evidence in the literature for similar systems for degradation of the other dyes.75–78
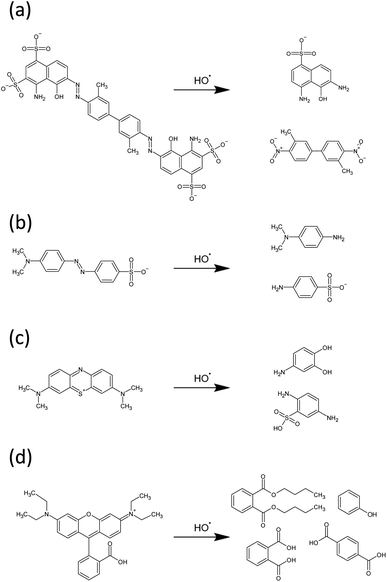 |
| Fig. 11 Proposed mechanisms of initial degradation steps of (a) EB, (b) MO, (c) MB and (d) RhB by hydroxyl radicals. | |
4 Conclusions
Thus, we have developed novel LDH/BNOx nanocomposite membranes for advanced NF applications. The membranes have been produced using multiple processing steps, including co-precipitation, liquid-phase exfoliation, and vacuum filtration techniques. The excellent NF performance of these membranes was demonstrated, with up to 100% dye retention being achieved. The dye retention and solvent flux results are in line with some of the best reported to date. Furthermore, the membranes have demonstrated excellent photocatalytic degradation and removal of dyes under visible-light ambient conditions. This approach should enable us to address the critical membrane fouling problem, allowing for regeneration and re-use of the membranes. However, currently our membranes are fragile and require the presence of the Durapore membrane filter template as a substrate for mechanical stability. Our future work will involve the improvement of the mechanical stability of membranes by chemical cross-linking of the nanosheets to produce membranes with higher mechanical integrity. Nevertheless, we believe this work represents a significant step forward in advanced nanofiltration technology.
Author contributions
Conceptualisation: Y. K. G., Á. C. and N. G. D.; methodology: Y. K. G., Á. C., N. G. D. and D. M. G.; formal analysis: Á. C., N. G. D., D. M. G., T. S., A. R. and A. D.; investigation: Á. C., N. G. D., D. M. G., T. S., A. R. Q. F. and A. D.; resources: Y. K. G.; data curation: Á. C., N. G. D., D. M. G. and T. S.; writing – original draft preparation: Á. C., D. M. G. and Y. K. G.; writing – review and editing: Á. C., N. G. D., D. M. G., T. S., A. R. and Y. K. G.; supervision: Á. C. and Y. K. G.; project administration: Y. K. G.; funding acquisition: Á. C. and Y. K. G.
Conflicts of interest
There are no conflicts to declare.
Acknowledgements
Á. C. and Y. K. G. acknowledge support from the Irish Research Council (grant number: GOIPG/2019/2788). N. G. D. and Y. K. G. acknowledge support from Science Foundation Ireland (SFI) and BiOrbic Bioeconomy Research Centre (grant number: SFI 16/RC/3889). T. S. and V. N. acknowledge support from the SFI Centre for Doctoral Training in Advanced Characterisation of Materials (CDT-ACM) (SFI Award Reference 18/EPSRC-CDT/3581). The authors also wish to thank the Advanced Microscopy Laboratory, Trinity College Dublin for their technical support and the use of their facilities.
References
-
Progress on Household Drinking Water, Sanitation and Hygiene I 2000–2017, New York, 2019 Search PubMed.
- R. Kishor, D. Purchase, G. D. Saratale, R. G. Saratale, L. F. R. Ferreira, M. Bilal, R. Chandra and R. N. Bharagava, Ecotoxicological and health concerns of persistent coloring pollutants of textile industry wastewater and treatment approaches for environmental safety, J. Environ. Chem. Eng., 2021, 9, 105012 CrossRef CAS.
- M. Imran, D. E. Crowley, A. Khalid, S. Hussain, M. W. Mumtaz and M. Arshad, Microbial biotechnology for decolorization of textile wastewaters, Rev. Environ. Sci. Biotechnol., 2015, 14, 73–92 CrossRef CAS.
- J. Khatri, P. V. Nidheesh, T. S. A. Singh and M. S. Kumar, Advanced oxidation processes based on zero-valent aluminium for treating textile wastewater, Chem. Eng. J., 2018, 348, 67–73 CrossRef CAS.
- G. McMullan, C. Meehan, A. Conneely, N. Kirby, T. Robinson, P. Nigam, I. M. Banat, R. Marchant and W. F. Smyth, Microbial decolourisation and degradation of textile dyes, Appl. Microbiol. Biotechnol., 2001, 56, 81–87 CrossRef CAS PubMed.
- B. Lellis, C. Z. Fávaro-Polonio, J. A. Pamphile and J. C. Polonio, Effects of textile dyes on health and the environment and bioremediation potential of living organisms, Biotechnol. Res. Innov., 2019, 3, 275–290 CrossRef.
- M. N. Morshed, S. Al Azad, H. Deb, B. B. Shaun and X. L. Shen, Titania-loaded cellulose-based functional hybrid nanomaterial for photocatalytic degradation of toxic aromatic dye in water, J. Water Process Eng., 2020, 33, 101062 CrossRef.
- S. Srivastava, R. Sinha and D. Roy, Toxicological effects of malachite green, Aquat. Toxicol., 2004, 66, 319–329 CrossRef CAS PubMed.
- N. G. Doménech, F. Purcell-Milton and Y. K. Gun'ko, Recent progress and future prospects in development of advanced materials for nanofiltration, Mater. Today Commun., 2020, 23, 100888 CrossRef.
- N. G. Doménech, F. Purcell-Milton, A. S. Arjona, M.-L. C. García, M. Ward, M. B. Cabré, A. Rafferty, K. McKelvey, P. Dunne and Y. K. Gun'ko, High-Performance Boron Nitride-Based Membranes for Water Purification, Nanomaterials, 2022, 12, 473 CrossRef PubMed.
- E. M. Vrijenhoek, S. Hong and M. Elimelech, Influence of membrane surface properties on initial rate of colloidal fouling of reverse osmosis and nanofiltration membranes, J. Membr. Sci., 2001, 188, 115–128 CrossRef CAS.
- L. Yang, X. Zhang, J. Rahmatinejad, B. Raisi and Z. Ye, Triethanolamine-based zwitterionic polyester thin-film composite nanofiltration membranes with excellent fouling-resistance for efficient dye and antibiotic separation, J. Membr. Sci., 2023, 670, 121355 CrossRef CAS.
- J. H. Jhaveri and Z. V. P. Murthy, A comprehensive review on anti-fouling nanocomposite membranes for pressure driven membrane separation processes, Desalination, 2016, 379, 137–154 CrossRef CAS.
- X. Chen, M. Qiu, H. Ding, K. Fu and Y. Fan, A reduced graphene oxide nanofiltration membrane intercalated by well-dispersed carbon nanotubes for drinking water purification, Nanoscale, 2016, 8, 5696–5705 RSC.
- L. Sun, H. Huang and X. Peng, Laminar MoS2 membranes for molecule separation, Chem. Commun., 2013, 49, 10718–10720 RSC.
- Á. Coogan and Y. K. Gun'ko, Solution-based “bottom-up” synthesis of group VI transition metal dichalcogenides and their applications, Mater. Adv., 2021, 2, 146–164 RSC.
- S. Homaeigohar and M. Elbahri, Graphene membranes for water desalination, NPG Asia Mater., 2017, 98(9), e427 CrossRef.
- C. Chen, J. Wang, D. Liu, C. Yang, Y. Liu, R. S. Ruoff and W. Lei, Functionalized boron nitride membranes with ultrafast solvent transport performance for molecular separation, Nat. Commun., 2018, 91(9), 1–8 Search PubMed.
- J. Fatima, A. N. Shah, M. B. Tahir, T. Mehmood, A. A. Shah, M. Tanveer, R. Nazir, B. L. Jan and S. Alansi, Tunable 2D Nanomaterials; Their Key Roles and Mechanisms in Water Purification and Monitoring, Front. Environ. Sci., 2022, 10, 210 Search PubMed.
- J. Zhang, Z. Li, K. Zhan, R. Sun, Z. Sheng, M. Wang, S. Wang and X. Hou, Two dimensional nanomaterial-based separation membranes, Electrophoresis, 2019, 40, 2029–2040 CrossRef CAS PubMed.
- N. G. Doménech, Á. Coogan, F. Purcell-Milton, M. L. C. García, A. S. Arjona, M. B. Cabré, A. Rafferty, K. Mckelvey, P. W. Dunne and Y. K. Gun'ko, Partially oxidised boron nitride as a 2D nanomaterial for nanofiltration applications, Nanoscale Adv., 2022, 4, 4895–4904 RSC.
- Y. Andriani, J. Song, P. C. Lim, D. H. L. Seng, D. M. Y. Lai, S. L. Teo, J. Kong, X. Wang, X. Zhang and S. Liu, Green and efficient production of boron nitride nanosheets via oxygen doping-facilitated liquid exfoliation, Ceram. Int., 2019, 45, 4909–4917 CrossRef CAS.
- Q. Wang and D. Ohare, Recent advances in the synthesis and application of layered double hydroxide (LDH) nanosheets, Chem. Rev., 2012, 112, 4124–4155 CrossRef CAS PubMed.
- G. Arrabito, A. Bonasera, G. Prestopino, A. Orsini, A. Mattoccia, E. Martinelli, B. Pignataro and P. G. Medaglia, Layered Double Hydroxides: A Toolbox for Chemistry and Biology, Crystals, 2019, 9, 361 CrossRef CAS.
- C. G. Silva, Y. Bouizi, V. Fornés and H. García, Layered double hydroxides as highly efficient photocatalysts for visible light oxygen generation from water, J. Am. Chem. Soc., 2009, 131, 13833–13839 CrossRef PubMed.
- J. Yu, F. Yu, M. F. Yuen and C. Wang, Two-dimensional layered double hydroxides as a platform for electrocatalytic oxygen evolution, J. Mater. Chem. A, 2021, 9, 9389–9430 RSC.
- C. Hobbs, S. Jaskaniec, E. K. McCarthy, C. Downing, K. Opelt, K. Güth, A. Shmeliov, M. C. D. Mourad, K. Mandel and V. Nicolosi, Structural transformation of layered double hydroxides: an in situ TEM analysis, npj 2D Mater. Appl., 2018, 2, 1–10 CrossRef CAS.
- P. Miao, J. Zhao, R. Shi, Z. Li, Y. Zhao, C. Zhou and T. Zhang, Layered Double Hydroxide Engineering for the Photocatalytic Conversion of Inactive Carbon and Nitrogen Molecules, ACS ES&T Engg, 2022, 2, 1088–1102 Search PubMed.
- M. S. Ahmed, M. S. J. Khan, S. B. Khan, K. Akhtar, E. M. Bakhsh, A. Khalil, S. A. Khan, A. M. Asiri and T. Kamal, Ni–Al-layered double-hydroxide photocatalyst for the visible light-assisted photodegradation of organic dye pollutants, Appl. Nanosci., 2022, 12, 3597–3606 CrossRef CAS.
- D. Saliba, S. E. El Jamal, A. Jonderian, M. Ammar, M. Hmadeh and M. Al-Ghoul, Tuning the structural properties of cadmium–aluminum layered double hydroxide for enhanced photocatalytic dye degradation, RSC Adv., 2020, 10, 43066–43074 RSC.
- S. Berner, P. Araya, J. Govan and H. Palza, Cu/Al and Cu/Cr based layered double hydroxide nanoparticles as adsorption materials for water treatment, J. Ind. Eng. Chem., 2018, 59, 134–140 CrossRef CAS.
- Á. Coogan, L. Hughes, F. Purcell-Milton, S. Cardiff, V. Nicolosi and Y. K. Gun'ko, Two-Dimensional Chiroptically Active Copper Oxide Nanostructures, J. Phys. Chem. C, 2022, 126, 18980–18987 CrossRef.
- S. E. Page, W. A. Arnold and K. McNeill, Terephthalate as a probe for photochemically generated hydroxyl radical, J. Environ. Monit., 2010, 12, 1658–1665 RSC.
- C. Wei, X. Yan, Y. Zhou, W. Xu, Y. Gan, Y. Zhang and N. Zhang, Morphological Control of Layered Double Hydroxides Prepared by Co-Precipitation Method, Crystals, 2022, 12, 1713 CrossRef CAS.
- A. V. Radha and P. V. Kamath, Oxidative leaching of chromium from layered double hydroxides: mechanistic studies, Bull. Mater. Sci., 2004, 27, 355–360 CrossRef CAS.
- T. Tanaka, Y. Kameshima, S. Nishimoto and M. Miyake, Determination of carbonate ion contents in layered double hydroxides by FTIR spectrometry, Anal. Methods, 2012, 4, 3925–3927 RSC.
- C. Backes, B. M. Szydłowska, A. Harvey, S. Yuan, V. Vega-Mayoral, B. R. Davies, P. L. Zhao, D. Hanlon, E. J. G. Santos, M. I. Katsnelson, W. J. Blau, C. Gadermaier and J. N. Coleman, Production of highly monolayer enriched dispersions of liquid-exfoliated nanosheets by liquid cascade centrifugation, ACS Nano, 2016, 10, 1589–1601 CrossRef CAS PubMed.
- M. Xu, Q. Tang, Y. Liu, J. Shi, W. Zhang, C. Guo, Q. Liu, W. Lei and C. Chen, Charged Boron Nitride Nanosheet Membranes for Improved Organic Solvent Nanofiltration, ACS Appl. Mater. Interfaces, 2023, 15, 12524–12533 CrossRef CAS PubMed.
- M. Sajid, S. M. S. Jillani, N. Baig and K. Alhooshani, Layered double hydroxide-modified membranes for water treatment: recent advances and prospects, Chemosphere, 2022, 287, 132140 CrossRef CAS PubMed.
- H. Jee, J. Jang, Y. Kang, T. Eisa, K. J. Chae, I. S. Kim and E. Yang, Enhancing the Dye-Rejection Efficiencies and Stability of Graphene Oxide-Based Nanofiltration Membranes via Divalent Cation Intercalation and Mild Reduction, Membranes, 2022, 12, 402 CrossRef CAS PubMed.
- L. Sun, Y. Ying, H. Huang, Z. Song, Y. Mao, Z. Xu and X. Peng, Ultrafast molecule separation through layered WS2 nanosheet membranes, ACS Nano, 2014, 8, 6304–6311 CrossRef CAS PubMed.
- G. Darmograi, B. Prelot, G. Layrac, D. Tichit, G. Martin-Gassin, F. Salles and J. Zajac, Study of Adsorption and Intercalation of Orange-Type Dyes into Mg-Al Layered Double Hydroxide, J. Phys. Chem. C, 2015, 119, 23388–23397 CrossRef CAS.
- R. Sasai, H. Sato, M. Sugata, T. Fujimura, S. Ishihara, K. Deguchi, S. Ohki, M. Tansho, T. Shimizu, N. Oita, M. Numoto, Y. Fujii, S. Kawaguchi, Y. Matsuoka, K. Hagura, T. Abe and C. Moriyoshi, Why Do Carbonate Anions Have Extremely High Stability in the Interlayer Space of Layered Double Hydroxides? Case Study of Layered Double Hydroxide Consisting of Mg and Al (Mg/Al = 2), Inorg. Chem., 2019, 58, 10928–10935 CrossRef CAS PubMed.
- I. Itskou, A. L'Hermitte, S. Marchesini, T. Tian and C. Petit, How to Tailor Porous Boron Nitride Properties for Applications in Interfacial Processes, Acc. Mater. Res., 2023, 4, 143–155 CrossRef CAS PubMed.
- T. Chen, M. Li and J. Liu, π-π Stacking Interaction: A Nondestructive and Facile Means in Material Engineering for Bioapplications, Cryst. Growth Des., 2018, 18, 2765–2783 CrossRef CAS.
- R. Das, P. Solís-Fernández, D. Breite, A. Prager, A. Lotnyk, A. Schulze and H. Ago, High flux and adsorption based non-functionalized hexagonal boron nitride lamellar membrane for ultrafast water purification, Chem. Eng. J., 2021, 420, 127721 CrossRef CAS.
-
Nanofiltration (NF), https://www.dupont.com/water/technologies/nanofiltration-nf.html, accessed, 2 May 2023 Search PubMed.
- R. S. Bangari, A. Yadav, P. Awasthi and N. Sinha, Experimental and theoretical analysis of simultaneous removal of methylene blue and tetracycline using boron nitride nanosheets as adsorbent, Colloids Surf., A, 2022, 634, 127943 CrossRef CAS.
- G. Rathee, A. Awasthi, D. Sood, R. Tomar, V. Tomar and R. Chandra, A new biocompatible ternary Layered Double Hydroxide Adsorbent for ultrafast removal of anionic organic dyes, Sci. Rep., 2019, 91(9), 1–14 Search PubMed.
- W. J. Weber Jr and J. C. Morris, Kinetics of Adsorption on Carbon from Solution, J. Sanit. Eng. Div., 1963, 89, 31–59 CrossRef.
- T. Sekine and K. Nakatani, Intraparticle diffusion and adsorption isotherm for sorption in silica gel studied by single-microparticle injection and microabsorption methods, Langmuir, 2002, 18, 694–697 CrossRef CAS.
- T. Hou, H. Zhang, D. He, Q. Liu, Z. Zhang, L. Xiao, W. Li and M. Barnes, Enhanced adsorption behaviors of Co2+ on robust chitosan hydrogel microspheres derived from an alkali solution system: kinetics and isotherm analysis, RSC Adv., 2018, 8, 36858–36868 RSC.
- A. Pholosi, E. B. Naidoo and A. E. Ofomaja, Intraparticle diffusion of Cr(VI) through biomass and magnetite coated biomass: a comparative kinetic and diffusion study, S. Afr. J. Chem. Eng., 2020, 32, 39–55 Search PubMed.
- N. Baliarsingh, K. M. Parida and G. C. Pradhan, Effects of Co, Ni, Cu, and Zn on photophysical and photocatalytic properties of carbonate intercalated MII/Cr LDHs for enhanced photodegradation of methyl orange, Ind. Eng. Chem. Res., 2014, 53, 3834–3841 CrossRef CAS.
- L. Mohapatra and K. Parida, A review on the recent progress, challenges and perspective of layered double hydroxides as promising photocatalysts, J. Mater. Chem. A, 2016, 4, 10744–10766 RSC.
- N. Ahmed, Y. Shibata, T. Taniguchi and Y. Izumi, Photocatalytic conversion of carbon dioxide into methanol using zinc–copper–M(III) (M = aluminum, gallium) layered double hydroxides, J. Catal., 2011, 279, 123–135 CrossRef CAS.
- X. Yuan and W. Li, Graphitic-C3N4 modified ZnAl-layered double hydroxides for enhanced photocatalytic removal of organic dye, Appl. Clay Sci., 2017, 138, 107–113 CrossRef CAS.
- K. Abderrazek, F. S. Najoua and E. Srasra, Synthesis and characterization of [Zn–Al] LDH: study of the effect of calcination on the photocatalytic activity, Appl. Clay Sci., 2016, 119, 229–235 CrossRef CAS.
- H. Fazal, A. Iqbal, Y. Cao, J. Zai, N. Ali, Y. Zhang, X. Wu, X. Zhang and X. Qian, Porous urchin-like 3D Co(II)Co(III) layered double hydroxides for high performance heterogeneous Fenton degradation, CrystEngComm, 2021, 23, 1234–1242 RSC.
- S. Nayak, K. Parida and O. D. Pavel, MgCr-LDH Nanoplatelets as Effective Oxidation Catalysts for Visible Light-Triggered Rhodamine B Degradation, Catalysts, 2021, 11, 1072 CrossRef CAS.
- S. J. M. Nassar, C. Wills and A. Harriman, Inhibition of the Photobleaching of Methylene Blue by Association with Urea, ChemPhotoChem, 2019, 3, 1042–1049 CrossRef CAS.
- G. Cassabois, P. Valvin and B. Gil, Hexagonal boron nitride is an indirect bandgap semiconductor, Nat. Photonics, 2016, 104(10), 262–266 CrossRef.
- L. Duan, B. Wang, K. Heck, S. Guo, C. A. Clark, J. Arredondo, M. Wang, T. P. Senftle, P. Westerhoff, X. Wen, Y. Song and M. S. Wong, Efficient Photocatalytic PFOA Degradation over Boron Nitride, Environ. Sci. Technol. Lett., 2020, 7, 613–619 CrossRef CAS.
- A. Harvey, C. Backes, J. B. Boland, X. He, A. Griffin, B. Szydlowska, C. Gabbett, J. F. Donegan and J. N. Coleman, Non-resonant light scattering in dispersions of 2D nanosheets, Nat. Commun., 2018, 91(9), 1–11 Search PubMed.
- S. Velu, K. Suzuki, M. Okazaki, M. P. Kapoor, T. Osaki and F. Ohashi, Oxidative Steam Reforming of Methanol over CuZnAl(Zr)-Oxide Catalysts for the Selective Production of Hydrogen for Fuel Cells: Catalyst Characterization and Performance Evaluation, J. Catal., 2000, 194, 373–384 CrossRef CAS.
- S. Velu, K. Suzuki, S. Hashimoto, N. Satoh, F. Ohashi and S. Tomura, The effect of cobalt on the structural properties and reducibility of CuCoZnAl layered double hydroxides and their thermally derived mixed oxides, J. Mater. Chem., 2001, 11, 2049–2060 RSC.
- S. J. Xia, F. X. Liu, Z. M. Ni, J. L. Xue and P. P. Qian, Layered double hydroxides as efficient photocatalysts for visible-light degradation of Rhodamine B, J. Colloid Interface Sci., 2013, 405, 195–200 CrossRef CAS PubMed.
- Z. R. Chen, Y. Q. Zhu, S. M. Xu, Y. Zhao, Q. Peng and H. Yan, Theoretical study on the anisotropic photo-induced carrier mobilities in layered double hydroxide-based photocatalysts, J. Mater. Chem. A, 2021, 9, 20466–20482 RSC.
- T. E. Eriksen, J. Lind and G. Merényi, On the acid-base equilibrium of the carbonate radical, Radiat. Phys. Chem., 1985, 26, 197–199 CrossRef CAS.
- J. Li, S. Zhang, Y. Chen, T. Liu, C. Liu, X. Zhang, M. Yi, Z. Chu and X. Han, A novel three-dimensional hierarchical CuAl layered double hydroxide with excellent catalytic activity for degradation of methyl orange, RSC Adv., 2017, 7, 29051–29057 RSC.
- Z. Z. Yang, C. Zhang, G. M. Zeng, X. F. Tan, H. Wang, D. L. Huang, K. H. Yang, J. J. Wei, C. Ma and K. Nie, Design and engineering of layered double hydroxide based catalysts for water depollution by advanced oxidation processes: a review, J. Mater. Chem. A, 2020, 8, 4141–4173 RSC.
- A. D. Bokare and W. Choi, Review of iron-free Fenton-like systems for activating H2O2 in advanced oxidation processes, J. Hazard. Mater., 2014, 275, 121–135 CrossRef CAS PubMed.
- M. Myilsamy, M. Mahalakshmi, V. Murugesan and N. Subha, Enhanced photocatalytic activity of nitrogen and indium co-doped mesoporous TiO2 nanocomposites for the degradation of 2,4-dinitrophenol under visible light, Appl. Surf. Sci., 2015, 342, 1–10 CrossRef CAS.
- K. T. Chung, The significance of azo-reduction in the mutagenesis and carcinogenesis of azo dyes, Mutat. Res., Genet. Toxicol., 1983, 114, 269–281 CrossRef CAS PubMed.
- R. T. Kapoor, M. Danish, R. S. Singh, M. Rafatullah and A. K. Abdul, Exploiting microbial biomass in treating azo dyes contaminated wastewater: mechanism of degradation and factors affecting microbial efficiency, J. Water Process Eng., 2021, 43, 102255 CrossRef.
- S. Wang, Y. Jia, L. Song and H. Zhang, Decolorization and Mineralization of Rhodamine B in Aqueous Solution with a Triple System of Cerium(IV)/H2O2/Hydroxylamine, ACS Omega, 2018, 3, 18456–18465 CrossRef CAS PubMed.
- Q. Wang, S. Tian and P. Ning, Degradation mechanism of methylene blue in a heterogeneous fenton-like reaction catalyzed by ferrocene, Ind. Eng. Chem. Res., 2014, 53, 643–649 CrossRef CAS.
- V. S. Antonin, S. Garcia-Segura, M. C. Santos and E. Brillas, Degradation of Evans Blue diazo dye by electrochemical processes based on Fenton's reaction chemistry, J. Electroanal. Chem., 2015, 747, 1–11 CrossRef CAS.
|
This journal is © The Royal Society of Chemistry 2023 |