DOI:
10.1039/D3QI00108C
(Research Article)
Inorg. Chem. Front., 2023,
10, 2414-2422
Electroreduction of CO2 to syngas with controllable H2/CO ratios in a wide potential range over Ni–N co-doped ultrathin carbon nanosheets†
Received
15th January 2023
, Accepted 9th March 2023
First published on 10th March 2023
Abstract
The conversion of CO2 to syngas (H2 and CO) via electrochemical reduction has been considered a promising strategy to mitigate the greenhouse effect. However, it is a great challenge to control H2/CO ratios over a wide voltage window. Herein, a new method of fabricating Ni–N co-doped carbon nanosheets by molten salt-assisted pyrolysis, impregnation and re-carbonization is proposed. Benefiting from their ultrathin structure and tunable Ni–Nx active site content, the H2/CO ratios can be adjusted from 1/2 to 2/1 within a wide applied voltage range (−0.7 to −1.3 V vs. RHE). After electrochemical stability testing for 10 h, the current density and H2/CO ratios remained almost constant, revealing robust long-term stability. This work may benefit the construction of efficient and low-budget electrocatalysts for the production of tunable syngas.
Introduction
With the acceleration of industrialization, the excessive consumption of fossil fuels has caused an energy and environmental crisis.1,2 To alleviate this problem, many emerging technologies such as capture and sequestration, chemical fixation, and electro/photochemical reduction have been proposed.3,4 Of the above technologies, the electrochemical CO2 reduction reaction (CO2RR) has been regarded as a promising strategy for producing fuels and chemicals from renewable energy sources.5 In recent years, considerable advances have been made in the CO2RR, in which the reduction of CO2 to low-carbon hydrocarbons is an intermediate step in the further production of high-value-added products.6,7 Among them, syngas (H2 and CO) is key feedstock for many important chemicals; for example, when the ratio of H2/CO is 2/1, it is an ideal choice for methanol production while the optimal ratio for dimethyl ether production is 1/1.8,9 Therefore, the realization of adjustable syngas production is very promising and also urgently needed.
In recent years, many attempts have been made to produce syngas via the CO2RR. For instance, Kim et al. used Ag/TiO2 as catalysts to produce tunable syngas.10 These catalysts could regulate H2/CO ratios from 0.1 to 1.5 by controlling oxygen vacancy contents in the range of applied potentials from −0.35 to −0.65 V (vs. RHE). He et al. developed Pd–SnO2 nanosheet catalysts for syngas production with controllable H2/CO ratios.11 The large specific surface area of these catalysts exposes abundant active interfaces that can modulate the H2/CO ratios from 0.28 to 4.2, when the potential is varied from −0.5 to −1.1 V (vs. RHE). However, precious metal catalysts have the disadvantages of high cost and scarce raw materials, which limit their large-scale application. Therefore, transition metal catalysts for electrosynthesis of syngas were also studied. Maeng et al. fabricated ZnO nanorod-based catalysts to prepare syngas.12 By the surface modification of the employed catalysts, the H2/CO ratios can be adjusted in the range of 0.25–4.5 within the applied potential range of −1.2 to −2.0 V (vs. Ag/AgCl). Chen et al. synthesized oxide-derived Cu nanowires with two-phase CuO heterostructures for syngas production.13 Misfit dislocation sites on the surface of Cu nanowires promote the selectivity of CO, so that the H2/CO ratios can be regulated from 1 to 3 within a certain potential window (−0.28 to −0.649 V vs. RHE). To further improve the stability of catalysts and reduce the cost, some researchers have developed carbon-based catalysts. Han et al. prepared BPNC catalysts for producing syngas via the CO2RR.14 By changing the precursor ratio of tetraphenylphosphonium tetraphenylborate (C48H40BP) to melamine, the H2/CO ratios can be adjusted from 0.2 to 6.8 in a potential window from −0.5 to −1.0 V (vs. RHE). Li et al. designed a series of nitrogen-doped carbon foam tubular electrodes to prepare syngas with controllable H2/CO ratios.15 These electrodes exhibit adjustable H2/CO ratios (1/3 to 2/1) over a potential range from −0.5 to −1.3 V (vs. RHE). Inspired by the above research, the syngas ratio can be adjusted by regulating the number of active sites for CO production. Compared with the heteroatom-doped carbon catalysts, Ni–N-doped carbon materials have the advantages of higher atomic utilization, higher intrinsic activity and controllable active sites.16–22 Benefiting from those merits, it is possible to control the amount of CO by regulating the number of Ni–N active sites, thereby adjusting the proportion of syngas.
Recently, Leverett et al. synthesized undercoordinated Ni–Nx catalysts with a holey graphene framework to adjust the H2/CO ratio by altering the coordination of Ni and N.23 He et al. prepared N-doped carbon-supported single-atom catalysts CoNi-NC and regulated the syngas composition by controlling the doping ratio of Co to Ni.24 Although the above studies have realized the regulation of the syngas ratios to some extent, the process of large-scale application of such catalysts is limited by the narrow adjustment ratio range or high cost of raw materials.
Herein, we synthesized Ni–N co-doped ultrathin carbon nanosheets by a molten salt-assisted pyrolysis, impregnation and re-carbonization strategy. The ultrathin structure of Ni–N co-doped carbon nanosheets can shorten the mass transfer path and provide a sufficient three-phase reaction interface for the CO2RR. The number of Ni–N active sites can be adjusted by varying the carbonization temperature, thus enabling the adjustment of the CO yield and the regulation of the resulting syngas ratios. Therefore, the ratio of H2/CO can be effectively regulated from 1/2 to 2/1 within a wide potential range (−0.70 to −1.30 V vs. RHE), which shows great promise for practical electrosynthesis of tunable syngas.
Results and discussion
The synthetic procedure of Ni/N–C is illustrated in Fig. 1a. In a typical process, coal tar pitch (carbon source) was mixed with salts (NaCl and KCl) and ground uniformly, followed by pyrolysis under an Ar atmosphere. During pyrolysis, the strong sheer force of the molten salts converted the pitch precursors into ultrathin carbon nanosheets. Subsequently, the as-prepared carbon nanosheets were treated with concentrated nitric acid to introduce nitro groups to facilitate Ni2+ adsorption. Then, Ni2+ was introduced into the carbon nanosheets by impregnation. The carbon nanosheets, after adsorption of Ni2+, were then carbonized together with N sources under an Ar atmosphere. The product obtained after acid leaching and drying was labelled Ni/N–C. The Ni–C sample without the N source and the N–C sample without the Ni source were prepared by a similar route to demonstrate the significance of the interaction between N and Ni species. The structural characteristics of the obtained Ni/N–C-900 were examined by scanning electron microscopy (SEM) (Fig. 1b–d) and transmission electron microscopy (TEM) (Fig. 1e–g). Ni/N–C-900 clearly shows an ultrathin lamellar structure, which reduces the ion transport resistance and increases the accessible area of the active sites. Meanwhile, there was no metal aggregation in Ni/N–C-900. The N–C and Ni–C samples exhibit similar sheet-like structures to that of Ni/N–C-900 (Fig. S1†); the influence of material morphology on the catalytic performance can be excluded. Energy dispersive spectroscopy (EDS) mapping displays that N, O and Ni atoms were dispersed uniformly in the carbon nanosheets (Fig. 1h).
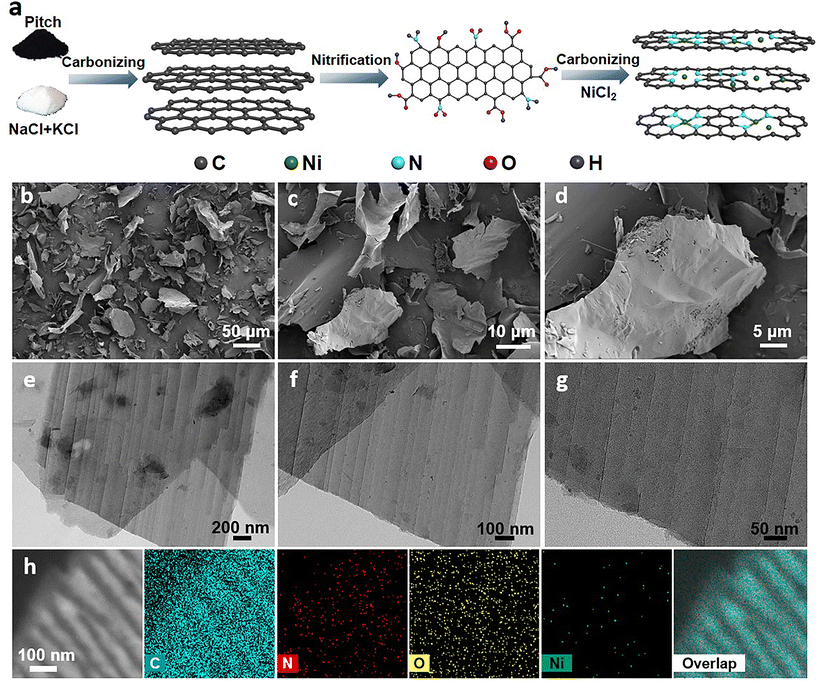 |
| Fig. 1 (a) Schematic diagram of the synthetic procedure of the Ni/N–C catalysts. (b–d) SEM, (e–g) TEM and (h) EDS mapping images of Ni/N–C-900. | |
The microstructures of the Ni/N–C catalysts were further investigated by X-ray diffraction (XRD) (Fig. 2a). The XRD patterns of the Ni/N–C catalysts exhibit only two broad peaks located at around 26° and 44°, attributed to the (002) and (101) facets of graphite,25 further suggesting the absence of metallic Ni nanoparticles in these catalysts. The crystal structure and defects of the prepared catalysts were evaluated with the Raman spectra. All the Ni/N–C catalysts show two characteristic bands at around 1340 cm−1 (D band) and 1580 cm−1 (G band) (Fig. 2b).26–28
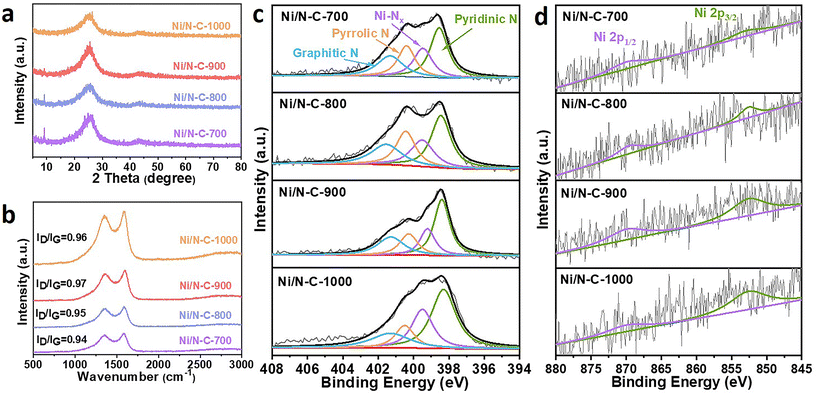 |
| Fig. 2 (a) XRD patterns and (b) Raman spectra of various Ni/N–C catalysts. High-resolution XPS spectra of (c) N 1s and (d) Ni 2p in Ni/N–C-700, 800, 900 and 1000. | |
Specifically, the ID/IG values of Ni/N–C-700, 800, 900 and 1000 are 0.94, 0.95, 0.97 and 0.96, respectively. The close ID/IG values of these four samples indicate that they all have similar graphitized structures, but these values are significantly higher than those of Ni–C (0.91) and N–C (0.93) (Fig. S2†), proving that the introduction of Ni–N active sites leads to an increase of disorder in carbon and thus more defects are obtained.29
To further reveal the elemental composition and coordination environment of Ni/N–C catalysts, X-ray photoelectron spectroscopy (XPS) measurements were carried out. The XPS wide-survey spectra of the Ni/N–C catalysts indicate the presence of C, N, O and Ni elements (Fig. S3†), which is in accordance with the EDS mapping. The C 1s spectra can be fitted into C–C (∼284.6 eV), C–N (∼285.6 eV) and C
O (∼287.8 eV) (Fig. S4†), demonstrating that the N species are successfully doped into the carbon nanosheets.30–32 As shown in Fig. 2c, the N 1s spectra can be deconvoluted into four peaks centered at 398.3 eV (pyridinic-N), 399.5 eV (Ni–N), 400.5 eV (pyrrolic-N) and 401.3 eV (graphitic-N).33,34 The contents of various N species are shown in Fig. S5;† the pyridinic-N was dominant in all samples. More importantly, pyridinic-N has strong adsorption capacity for acidic CO2 molecules, thus enhancing the CO2RR rate.14
The Ni contents of the various samples characterized by XPS are 0.09 at% (Ni/N–C-700), 0.10 at% (Ni/N–C-800), 0.16 at% (Ni/N–C-900), and 0.13 at% (Ni/N–C-1000) (Table S1†), respectively, indicating that 900 °C is a potentially ideal temperature for preparing the Ni/N–C catalysts with relatively high Ni content. Meanwhile, we calculated the relative contents of nitrogen species in various Ni/N–C catalysts. Fig. S5† shows that the relative contents of pyridinic-N are 35.7, 33.3, 27.6 and 25.9%, when the carbonization temperature is 700, 800, 900 and 1000 °C, respectively. Meanwhile, the corresponding relative contents of pyrrolic-N are 21.3, 20.0, 17.6 and 17.3%, respectively. Obviously, the contents of both pyridinic-N and pyrrolic-N in the samples decrease by increasing the carbonization temperature. In contrast, the relative contents of graphitic-N in Ni/N–C-700, 800, 900 and 1000 are 21.2, 22.3, 24.2 and 31.7%, respectively. The possible reason is that the graphitic-N is more stable and is lost at a lower rate with the increasing temperature than the unstable pyridinic and pyrrolic-N. It is noteworthy that the relative content of Ni–Nx reaches its maximum at 900 °C (30.6%), while higher carbonization temperature will reduce the number of Ni–Nx species (1000 °C, 25.1%). All these results show that 900 °C is an optimum temperature for the formation of Ni–Nx species (1.18 at%) and the maintenance of a high total nitrogen content (3.85 at%), which are beneficial for improving the catalytic performance. Thus, the number of Ni–Nx active sites can be modulated by changing the carbonization temperature, thus further adjusting the H2/CO ratios.35,36 Although the main active sites in Ni/N–C catalysts are Ni–Nx coordinated structures, graphitic-N, pyridinic-N and pyrrolic-N also play important roles in the CO2RR, in which pyridinic-N is a dominant factor. Therefore, the Ni–Nx active sites and N species have synergistic effects on the CO2RR activity in the Ni/N–C electrocatalysts.37,38
The high-resolution spectra of Ni 2p are displayed in Fig. 2d, where the Ni 2p3/2 peak at 855.5 eV is between those of Ni0 (852.5–853.0 eV) and Ni2+ (856 eV),39–41 revealing that the Ni species are in an unsaturated chemical state and the potential presence of coordinated Ni–N sites in the catalysts. In addition, the Ni contents were also detected by inductively coupled plasma-optical emission spectroscopy (ICP-OES), where the Ni/N–C-900 catalyst (0.00936 wt%) possesses higher Ni content than those of Ni/N–C-700 (0.00230 wt%), Ni/N–C-800 (0.00283 wt%) and Ni/N–C-1000 (0.00381 wt%) (Table S2†), which agrees well with the XPS results. The high-resolution N 1s and Ni 2p spectra of N–C and Ni–C samples are shown in Fig. S6,† demonstrating the absence of Ni–Nx active sites. The trace amount of N species (0.00188 wt%) in the Ni–C sample comes from coal tar pitch, which is insufficient to form Ni–Nx active sites with Ni sources.
The electrochemical activities of various electrocatalysts were investigated in an H-type cell. As revealed by linear sweep voltammetry (LSV) curves in Fig. 3a, the current densities of various catalysts under a CO2 atmosphere are significantly higher than those of catalysts under an Ar atmosphere, proving their preference towards the CO2RR. Compared with N–C (1.25 mA cm−2) and Ni–C (1.47 mA cm−2), Ni/N–C-900 exhibits higher current density (8.37 mA cm−2) (Fig. S7a†), demonstrating that the ultrathin carbon nanosheets can promote the performance of the CO2RR.42 To determine the composition of the resulting syngas, the H2/CO ratios of various samples are calculated at different potentials (Fig. 3b). Obviously, the controllable ratios of H2/CO vary from 1/2 to 2/1, providing an opportunity for tunable syngas production. Notably, most of the H2/CO ratios of each sample at different potentials are distributed in the optimal region (0.5–2), and the syngas contents in this interval are the key feedstocks for preparing multiple chemical products.24,43–47 The H2/CO ratios of N–C and Ni–C (Fig. S7b†) show that most of the ratios deviated from the optimal syngas region, further demonstrating that the presence of Ni–Nx active sites can improve the selectivity for CO and thereby modulate the H2/CO ratios.
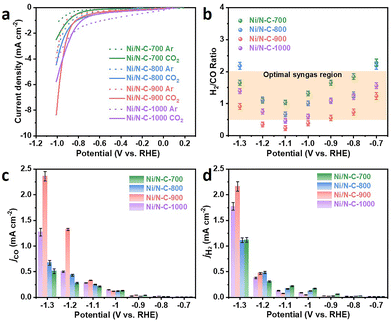 |
| Fig. 3 (a) Linear sweep voltammetric (LSV) curves of various samples in CO2 and Ar-saturated 0.1 M KHCO3 solution. (b) Dependence of the H2/CO ratio on the applied potentials of Ni/N–C-700, 800, 900 and 1000. Partial current densities of CO (c) and H2 (d). | |
The CO partial current densities (jCO) of various samples are 0.51 mA cm−2 (Ni/N–C-700), 0.68 mA cm−2 (Ni/N–C-800), 2.36 mA cm−2 (Ni/N–C-900), and 1.27 mA cm−2 (Ni/N–C-1000) (Fig. 3c). The jCO of Ni/N–C catalysts is higher than that of N–C (0.24 mA cm−2) and Ni–C (0.44 mA cm−2) (Fig. S7†), suggesting that the Ni–Nx coordination structure plays a vital role in the CO2RR. Meanwhile, the H2 partial current densities (jH2) (Fig. 3d) and jCO increase with the applied voltage; however, there are some differences in the magnitude of jH2 and jCO growth, indicating that the H2/CO ratios of various Ni/N–C catalysts can also be modulated by changing the applied potential. The syngas production rate is also a key parameter for estimating the catalytic performance.
The production rates of CO and H2 can vary with applied potentials (Fig. 4a and b). As predicted, the generation rates of CO and H2 gradually increase in a wide potential window (−0.7 to −1.1 V vs. RHE). Ni/N–C-900 displays the maximum CO production rate (19.96 mmol g−1 h−1) compared with those of Ni/N–C-700 (6.68 mmol g−1 h−1), Ni/N–C-800 (9.83 mmol g−1 h−1) and Ni/N–C-1000 (12.55 mmol g−1 h−1), demonstrating that the production rates of CO are consistent with the number of Ni–Nx active sites. From 700 to 900 °C, the number of Ni–Nx active sites in the catalysts gradually increases, the reactions are mainly based on the CO2RR, and the HER is inhibited, resulting in a gradual decrease of production rates for H2. As the temperature continued to rise to 1000 °C, the decrease in the number of Ni–Nx active sites caused a gradually enhanced HER. To further reveal the reaction kinetics of the catalysts, the Tafel slopes for CO production over Ni/N–C catalysts are presented in Fig. 4c. The Tafel slope of Ni/N–C-900 is 243.08 mV dec−1, which is lower than those of Ni/N–C-700 (315.23 mV dec−1), Ni/N–C-800 (258.51 mV dec−1) and Ni/N–C-1000 (257.05 mV dec−1), implying that the increased number of Ni–Nx active sites accelerates the reaction kinetics.48 Meanwhile, the Tafel slopes of Ni/N–C catalysts are significantly lower than those of N–C (322.85 mV dec−1) and Ni–C (319.59 mV dec−1) (Fig. S8†), further proving that the Ni–Nx active sites can accelerate the reaction kinetics of Ni/N–C catalysts in the CO2RR.49,50
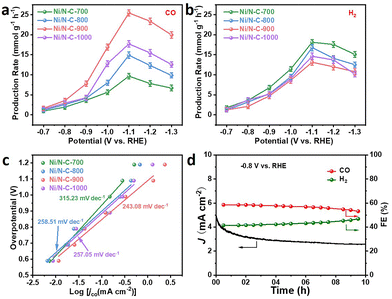 |
| Fig. 4 (a) CO and (b) H2 production rates of Ni/N–C catalysts at different applied potentials. (c) Tafel plots based on jCO of the Ni/N–C catalysts in CO2-saturated 0.1 M KHCO3 solution. (d) Long-term stability test of Ni/N–C-900 at −0.8 V vs. RHE for 10 h. | |
In addition, Fig. S9 and S10† show the electrochemically active surface areas (ECSAs) and the double-layer capacitances (Cdl) of all samples. The Cdl of Ni/N–C-900 (1.08 mF cm−2) is obviously larger than the Cdl of Ni/N–C-700 (0.613 mF cm−2), Ni/N–C-800 (0.842 mF cm−2), Ni/N–C-1000 (0.863 mF cm−2), N–C (0.365 mF cm−2) and Ni–C (0.426 mF cm−2). The results show that the ESCA of Ni/N–C-900 is the largest among the above samples, which is conducive to increasing the contact area between active sites and CO2. The jCO of different catalysts is normalized to the ECSA to fairly compare the catalytic activity of Ni/N–C-900 with other samples. As shown in Fig. S11,† Ni/N–C-900 displays a significantly larger ESCA-normalized CO current density than the N–C and Ni–C samples. Thus, the higher intrinsic activity of Ni/N–C-900 is attributed to its unique Ni–Nx sites. An isotope tracer measurement for the electroreduction of 13CO2 was carried out to trace the origin of the products, where 12CO2 was used as a reference. As shown in Fig. S12,† the dominant peak of 13CO (m/z = 29) was observed at m/z = 29, which was assigned to 13CO produced during the MS measurement. The results verify that CO detected upon the Ni/N–C materials comes from the electroreduction of the CO2 source instead of any organic impurities from the catalyst. Furthermore, Ni/N–C-900 exhibits significant electrochemical stability in a long-term electrolysis test. When the reaction continues for 10 h at −0.8 V vs. RHE, the H2/CO ratio remains basically stable and the current density decreases only slightly at the beginning (Fig. 4d). At the beginning of the reaction, sufficient CO2 is adsorbed on the electrode and a large amount of CO2 is consumed instantaneously after applying the potential. However, as the reaction proceeds, the insufficient supply of CO2 leads to a decrease in the current density. To verify the reason for the decrease of FEco after 10 h of electrolysis, we investigated the chemical states of N species. The relative contents of various N dopants show no obvious differences compared with the fresh Ni/N–C-900 sample. However, the atomic concentration of total N decreases from 3.85 to 3.02 at% (Fig. S13†), which may be the cause of deactivation.
In order to highlight the advantages of the stable and controlled syngas production within a wide potential window over the Ni–N co-doped ultrathin carbon nanosheet electrodes, a comparison between H2/CO ratios in this work and those in recent representative literature reports is presented in Fig. 5.
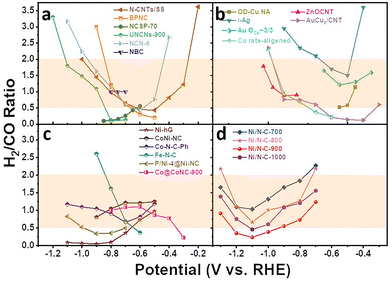 |
| Fig. 5 Recently reported H2/CO ratios of various catalysts used for syngas production at different applied potentials. (a) Heteroatom-doped carbon catalysts (N-CNTs,42 BPNC,14 NCSP-70,51 UNCNs-900,52 NCN-6,53 and NBC54), (b) metal catalysts (OD-Cu NA,55 ZnOCNT,56 I-Ag,57 AuCu2/CNT,58Au ΘCu ∼ 3/3,59 and Co rate-aligened60), (c) M–N–C single-atom catalysts (Ni-hG,23 CoNi-NC,24 Co–N–C–Ph,61 Fe–N–C,33 P/Ni-4@Ni-NC,62 and Co@CoNC-900 63) and (d) Ni–N co-doped ultrathin carbon nanosheet electrodes. The shaded regions are the areas with the optimal syngas ratios. | |
The H2/CO ratios over heteroatom-doped carbon catalysts and metal catalysts could be regulated in the range of 0–3.5, but it is far from the optimal range. Meanwhile, the weak intrinsic activity of heteroatom-doped carbon and the scarcity or cost disadvantages of metal catalysts still limit their large-scale application. Compared with the M–N–C catalysts in the literature, our Ni/N–C catalysts showed obvious advantages in the regulatory range, that is, it covered all the optimal syngas regions. Furthermore, the ratio of syngas on our catalysts can be controlled simultaneously by adjusting the active site content and applied potential. The unique and convenient regulation strategy of this work enables the H2/CO ratio to be adjusted between 1/2 and 2/1 within a wide voltage window, highlighting excellent intrinsic activity and economy.
Conclusions
In summary, this work presented Ni–N co-doped carbon nanosheets as promising catalysts for the electrochemical conversion of CO2 to tunable syngas. The number of Ni–N active sites in the catalysts is varied by adjusting the carbonization temperature of the synthesis process, enabling the effective regulation of H2/CO ratios to the optimal range (1/2–2/1) over a wide potential window (from −0.7 to −1.3 V vs. RHE). The scheme of modulating the H2/CO ratios by changing the number of Ni–Nx active sites shows great potential and may provide a valuable reference for future syngas production.
Author contributions
Kaining Gan: investigation, methodology, data curation, writing – original draft, review and editing. Hongqiang Li: supervision, conceptualization, methodology, writing – review and editing, and funding acquisition. Ran Li: investigation and validation. Jiabao Niu: investigation and methodology. Jun He: validation. Dedong Jia: review and editing, and funding acquisition. Xiaojun He: supervision and funding acquisition.
Conflicts of interest
There are no conflicts of interest to declare.
Acknowledgements
This work was partly supported by the National Natural Science Foundation of China [no. 22108003, 52072002, 51872005, 52002194], the Natural Science Foundation of Anhui Provincial Education Department [no. KJ2020A0254], the Research Fund for Young Teachers of Anhui University of Technology [no. QZ202007], and the Wanjiang Scholar Program. The authors would also like to acknowledge the financial support from the Anhui International Research Center of Energy Materials Green Manufacturing and Biotechnology.
References
- J. M. Spurgeon and B. Kumar, A comparative technoeconomic analysis of pathways for commercial electrochemical CO2 reduction to liquid products, Energy Environ. Sci., 2018, 11, 1536–1551 RSC.
- J. Hao, Z. Zhuang, J. Hao, K. Cao, Y. Hu, W. Wu, S. Lu, C. Wang, N. Zhang, D. Wang, M. Du and H. Zhu, Strain Relaxation in Metal Alloy Catalysts Steers the Product Selectivity of Electrocatalytic CO2 Reduction, ACS Nano, 2022, 16, 3251–3263 CrossRef CAS PubMed.
- F. Naseem, P. Lu, J. Zeng, Z. Lu, Y. H. Ng, H. Zhao, Y. Du and Z. Yin, Solid Nanoporosity Governs Catalytic CO2 and N2 Reduction, ACS Nano, 2020, 14, 7734–7759 CrossRef CAS PubMed.
- Z. Yin, J. Yu, Z. Xie, S.-W. Yu, L. Zhang, T. Akauola, J. G. Chen, W. Huang, L. Qi and S. Zhang, Hybrid Catalyst Coupling Single-Atom Ni and Nanoscale Cu for Efficient CO2 Electroreduction to Ethylene, J. Am. Chem. Soc., 2022, 144, 20931–20938 CrossRef CAS PubMed.
- H. Li, R. Li, J. Niu, K. Gan and X. He, Defect chemistry of electrocatalysts for CO2 reduction, Front. Chem., 2022, 10, 1067327 CrossRef CAS PubMed.
- H. Li, N. Xiao, M. Hao, X. Song, Y. Wang, Y. Ji, C. Liu, C. Li, Z. Guo, F. Zhang and J. Qiu, Efficient CO2 electroreduction over pyridinic-N active sites highly exposed on wrinkled porous carbon nanosheets, Chem. Eng. J., 2018, 351, 613–621 CrossRef CAS.
- H. Li, X. He, T. Wu, B. Jin, L. Yang and J. Qiu, Synthesis, modification strategies and applications of coal-based carbon materials, Fuel Process. Technol., 2022, 230, 107203 CrossRef CAS.
- Y. Jiang, R. Long and Y. Xiong, Regulating C–C coupling in thermocatalytic and electrocatalytic COx conversion based on surface science, Chem. Sci., 2019, 10, 7310–7326 RSC.
- Y. Liu, D. Tian, A. N. Biswas, Z. Xie, S. Hwang, J. H. Lee, H. Meng and J. G. Chen, Transition Metal Nitrides as Promising Catalyst Supports for Tuning CO/H2 Syngas Production from Electrochemical CO2 Reduction, Angew. Chem., Int. Ed., 2020, 59, 11345–11348 CrossRef CAS PubMed.
- Y. E. Kim, B. Kim, W. Lee, Y. N. Ko, M. H. Youn, S. K. Jeong, K. T. Park and J. Oh, Highly tunable syngas production by electrocatalytic reduction of CO2 using Ag/TiO2 catalysts, Chem. Eng. J., 2021, 413, 127448 CrossRef CAS.
- H. He, D. Xia, X. Yu, J. Wu, Y. Wang, L. Wang, L. Wu, J. Huang, N. Zhao, L. Deng and Y.-N. Liu, Pd-SnO2 interface enables synthesis of syngas with controllable H2/CO ratios by electrocatalytic reduction of CO2, Appl. Catal. B-Environ., 2022, 312, 121392 CrossRef CAS.
- J. Y. Maeng, J. H. Yang, H. J. Jang, M. H. Joo, Y. J. Kim, C. K. Rhee and Y. Sohn, Electrocatalytic syngas and photocatalytic long-chain hydrocarbon productions by CO2 reduction over ZnO and Zn-based electrodes, Appl. Surf. Sci., 2023, 609, 155349 CrossRef.
- P. Chen, Y. Jiao, Y.-H. Zhu, S.-M. Chen, L. Song, M. Jaroniec, Y. Zheng and S.-Z. Qiao, Syngas production from electrocatalytic CO2 reduction with high energetic efficiency and current density, J. Mater. Chem. A, 2019, 7, 7675–7682 RSC.
- J. Han, X. Deng, K. Chen, S. Imhanria, Y. Sun and W. Wang, Electrochemical conversion of CO2 into tunable syngas on a B, P, N tri-doped carbon, Renewable Energy, 2021, 177, 636–642 CrossRef CAS.
- H. Li, N. Xiao, Y. Wang, C. Li, X. Ye, Z. Guo, X. Pan, C. Liu, J. Bai, J. Xiao, X. Zhang, S. Zhao and J. Qiu, Nitrogen-doped tubular carbon foam electrodes for efficient electroreduction of CO2 to syngas with potential-independent CO/H2 ratios, J. Mater. Chem. A, 2019, 7, 18852–18860 RSC.
- T. Wang, Q. Zhao, Y. Fu, C. Lei, B. Yang, Z. Li, L. Lei, G. Wu and Y. Hou, Carbon-Rich Nonprecious Metal Single Atom Electrocatalysts for CO2 Reduction and Hydrogen Evolution, Small Methods, 2019, 3, 1900210 CrossRef CAS.
- F. Pan, W. Deng, C. Justiniano and Y. Li, Identification of champion transition metals centers in metal and nitrogen-codoped carbon catalysts for CO2 reduction, Appl. Catal. B-Environ., 2018, 226, 463–472 CrossRef CAS.
- A. S. Varela, W. Ju and P. Strasser, Molecular Nitrogen–Carbon Catalysts, Solid Metal Organic Framework Catalysts, and Solid Metal/Nitrogen-Doped Carbon (MNC) Catalysts for the Electrochemical CO2 Reduction, Adv. Energy Mater., 2018, 8, 1703614 CrossRef.
- H. Tao, C. Choi, L.-X. Ding, Z. Jiang, Z. Han, M. Jia, Q. Fan, Y. Gao, H. Wang, A. W. Robertson, S. Hong, Y. Jung, S. Liu and Z. Sun, Nitrogen Fixation by Ru Single-Atom Electrocatalytic Reduction, Chem, 2019, 5, 204–214 CAS.
- J. Zhao, Y. Zhang, X. Kang and Y. Li, The preparation of NiO/Ni–N/C nanocomposites and its electrocatalytic performance for methanol oxidation reaction, New J. Chem., 2020, 44, 14970–14978 RSC.
- W. Bi, X. Li, R. You, M. Chen, R. Yuan, W. Huang, X. Wu, W. Chu, C. Wu and Y. Xie, Surface Immobilization of Transition Metal Ions on Nitrogen-Doped Graphene Realizing High-Efficient and Selective CO2 Reduction, Adv. Mater., 2018, 30, 1706617 CrossRef PubMed.
- W.-j. Wang, C. Cao, K. Wang and T. Zhou, Boosting CO2 electroreduction to CO with abundant nickel single atom active sites, Inorg. Chem. Front., 2021, 8, 2542–2548 RSC.
- J. Leverett, R. Daiyan, L. Gong, K. Iputera, Z. Tong, J. Qu, Z. Ma, Q. Zhang, S. Cheong, J. Cairney, R.-S. Liu, X. Lu, Z. Xia, L. Dai and R. Amal, Designing Undercoordinated Ni–Nx and Fe–Nx on Holey Graphene for Electrochemical CO2 Conversion to Syngas, ACS Nano, 2021, 15, 12006–12018 CrossRef CAS PubMed.
- Q. He, D. Liu, J. H. Lee, Y. Liu, Z. Xie, S. Hwang, S. Kattel, L. Song and J. G. Chen, Electrochemical Conversion of CO2 to Syngas with Controllable CO/H2 Ratios over Co and Ni Single-Atom Catalysts, Angew. Chem., Int. Ed., 2020, 59, 3033–3037 CrossRef CAS PubMed.
- C. Lu, J. Yang, S. Wei, S. Bi, Y. Xia, M. Chen, Y. Hou, M. Qiu, C. Yuan, Y. Su, F. Zhang, H. Liang and X. Zhuang, Atomic Ni Anchored Covalent Triazine Framework as High Efficient Electrocatalyst for Carbon Dioxide Conversion, Adv. Funct. Mater., 2019, 29, 1806884 CrossRef.
- Q. Wu, J. Gao, J. Feng, Q. Liu, Y. Zhou, S. Zhang, M. Nie, Y. Liu, J. Zhao, F. Liu, J. Zhong and Z. Kang, A CO2 adsorption dominated carbon defect-based electrocatalyst for efficient carbon dioxide reduction, J. Mater. Chem. A, 2020, 8, 1205–1211 RSC.
- C. Yan, H. Li, Y. Ye, H. Wu, F. Cai, R. Si, J. Xiao, S. Miao, S. Xie, F. Yang, Y. Li, G. Wang and X. Bao, Coordinatively unsaturated nickel–nitrogen sites towards selective and high-rate CO2 electroreduction, Energy Environ. Sci., 2018, 11, 1204–1210 RSC.
- C.-Z. Yuan, L.-Y. Zhan, S.-J. Liu, F. Chen, H. Lin, X.-L. Wu and J. Chen, Semi-sacrificial template synthesis of single-atom Ni sites supported on hollow carbon nanospheres for efficient and stable electrochemical CO2 reduction, Inorg. Chem. Front., 2020, 7, 1719–1725 RSC.
- S. Li, X. Lu, S. Zhao, M. Ceccato, X.-M. Hu, A. Roldan, M. Liu and K. Daasbjerg, p-Block Indium Single-Atom Catalyst with Low-Coordinated In–N Motif for Enhanced Electrochemical CO2 Reduction, ACS Catal., 2022, 12, 7386–7395 CrossRef CAS.
- Y. Gang, F. Pan, Y. Fei, Z. Du, Y. H. Hu and Y. Li, Highly Efficient Nickel, Iron, and Nitrogen Codoped Carbon Catalysts Derived from Industrial Waste Petroleum Coke for Electrochemical CO2 Reduction, ACS Sustainable Chem. Eng., 2020, 8, 8840–8847 CrossRef CAS.
- N. Zhang, T. Zhou, M. Chen, H. Feng, R. Yuan, C. a. Zhong, W. Yan, Y. Tian, X. Wu, W. Chu, C. Wu and Y. Xie, High-purity pyrrole-type FeN4 sites as a superior oxygen reduction electrocatalyst, Energy Environ. Sci., 2020, 13, 111–118 RSC.
- E. Lepre, J. Heske, M. Nowakowski, E. Scoppola, I. Zizak, T. Heil, T. D. Kühne, M. Antonietti, N. López-Salas and J. Albero, Ni-based electrocatalysts for unconventional CO2 reduction reaction to formic acid, Nano Energy, 2022, 97, 107191 CrossRef CAS.
- J. Zhao, J. Deng, J. Han, S. Imhanria, K. Chen and W. Wang, Effective tunable syngas generation via CO2 reduction reaction by non-precious Fe-N-C electrocatalyst, Chem. Eng. J., 2020, 389, 124323 CrossRef CAS.
- F. Chang, Y. Ma, P. Su and J. Liu, Synthesis of a graphitized hierarchical porous carbon material supported with a transition metal for electrochemical conversion, Inorg. Chem. Front., 2022, 9, 1794–1801 RSC.
- Q. Lai, L. Zheng, Y. Liang, J. He, J. Zhao and J. Chen, Metal–Organic-Framework-Derived Fe-N/C Electrocatalyst with Five-Coordinated Fe-Nx Sites for Advanced Oxygen Reduction in Acid Media, ACS Catal., 2017, 7, 1655–1663 CrossRef CAS.
- Z. Ma, X. Zhang, D. Wu, X. Han, L. Zhang, H. Wang, F. Xu, Z. Gao and K. Jiang, Ni and nitrogen-codoped ultrathin carbon nanosheets with strong bonding sites for efficient CO2 electrochemical reduction, J. Colloid Interface Sci., 2020, 570, 31–40 CrossRef CAS PubMed.
- M. Jia, C. Choi, T.-S. Wu, C. Ma, P. Kang, H. Tao, Q. Fan, S. Hong, S. Liu, Y.-L. Soo, Y. Jung, J. Qiu and Z. Sun, Carbon-supported Ni nanoparticles for efficient CO2 electroreduction, Chem. Sci., 2018, 9, 8775–8780 RSC.
- X. Tan, C. Yu, S. Cui, L. Ni, W. Guo, Z. Wang, J. Chang, Y. Ren, J. Yu, H. Huang and J. Qiu, Activity descriptor of Ni,N-Codoped carbon electrocatalyst in CO2 electroreduction reaction, Chem. Eng. J., 2022, 433, 131965 CrossRef CAS.
- F. Pan, B. Li, E. Sarnello, Y. Fei, X. Feng, Y. Gang, X. Xiang, L. Fang, T. Li, Y. H. Hu, G. Wang and Y. Li, Pore-Edge Tailoring of Single-Atom Iron–Nitrogen Sites on Graphene for Enhanced CO2 Reduction, ACS Catal., 2020, 10, 10803–10811 CrossRef CAS.
- Y.-N. Gong, L. Jiao, Y. Qian, C.-Y. Pan, L. Zheng, X. Cai, B. Liu, S.-H. Yu and H.-L. Jiang, Regulating the Coordination Environment of MOF-Templated Single-Atom Nickel Electrocatalysts for Boosting CO2 Reduction, Angew. Chem., Int. Ed., 2020, 59, 2705–2709 CrossRef CAS PubMed.
- H. Cheng, X. Wu, X. Li, Y. Zhang, M. Feng, Z. Fan and G. He, Zeolitic imidazole framework-derived FeN5-doped carbon as superior CO2 electrocatalysts, J. Catal., 2021, 395, 63–69 CrossRef CAS.
- K.-H. Liu, H.-X. Zhong, X.-Y. Yang, D. Bao, F.-L. Meng, J.-M. Yan and X.-B. Zhang, Composition-tunable synthesis of “clean” syngas via a one-step synthesis of metal-free pyridinic-N-enriched self-supported CNTs: the synergy of electrocatalyst pyrolysis temperature and potential, Green Chem., 2017, 19, 4284–4288 RSC.
- A. Kamiyama, K. Kubota, T. Nakano, S. Fujimura, S. Shiraishi, H. Tsukada and S. Komaba, High-Capacity Hard Carbon Synthesized from Macroporous Phenolic Resin for Sodium-Ion and Potassium-Ion Battery, ACS Appl. Energy Mater., 2020, 3, 135–140 CrossRef CAS.
- L. Xiong, X. Zhang, L. Chen, Z. Deng, S. Han, Y. Chen, J. Zhong, H. Sun, Y. Lian, B. Yang, X. Yuan, H. Yu, Y. Liu, X. Yang, J. Guo, M. H. Rümmeli, Y. Jiao and Y. Peng, Geometric Modulation of Local CO Flux in Ag@Cu2O Nanoreactors for Steering the CO2RR Pathway toward High-Efficacy Methane Production, Adv. Mater., 2021, 33, 2101741 CrossRef CAS PubMed.
- B. Ren, Z. Zhang, G. Wen, X. Zhang, M. Xu, Y. Weng, Y. Nie, H. Dou, Y. Jiang, Y.-P. Deng, G. Sun, D. Luo, L. Shui, X. Wang, M. Feng, A. Yu and Z. Chen, Dual-Scale Integration Design of Sn–ZnO Catalyst toward Efficient and Stable CO2 Electroreduction, Adv. Mater., 2022, 34, 2204637 CrossRef CAS PubMed.
- J. Zhu, M. Xiao, D. Ren, R. Gao, X. Liu, Z. Zhang, D. Luo, W. Xing, D. Su, A. Yu and Z. Chen, Quasi-Covalently Coupled Ni–Cu Atomic Pair for Synergistic Electroreduction of CO2, J. Am. Chem. Soc., 2022, 144, 9661–9671 CrossRef CAS PubMed.
- W. Yang, J.-H. Zhang, R. Si, L.-M. Cao, D.-C. Zhong and T.-B. Lu, Efficient and steady production of 1
:
2 syngas (CO/H2) by simultaneous electrochemical reduction of CO2 and H2O, Inorg. Chem. Front., 2021, 8, 1695–1701 RSC.
- H. Li, K. Gan, R. Li, H. Huang, J. Niu, Z. Chen, J. Zhou, Y. Yu, J. Qiu and X. He, Highly Dispersed NiO Clusters Induced Electron Delocalization of Ni-N-C Catalysts for Enhanced CO2 Electroreduction, Adv. Funct. Mater., 2023, 33, 2208622 CrossRef CAS.
- P. Ding, H. Zhao, T. Li, Y. Luo, G. Fan, G. Chen, S. Gao, X. Shi, S. Lu and X. Sun, Metal-based electrocatalytic conversion of CO2 to formic acid/formate, J. Mater. Chem. A, 2020, 8, 21947–21960 RSC.
- X. Zhong, S. Liang, T. Yang, G. Zeng, Z. Zhong, H. Deng, L. Zhang and X. Sun, Sn Dopants with Synergistic Oxygen Vacancies Boost CO2 Electroreduction on CuO Nanosheets to CO at Low Overpotential, ACS Nano, 2022, 16, 19210–19219 CrossRef CAS PubMed.
- Y. Shang, Y. Ding, P. Zhang, M. Wang, Y. Jia, Y. Xu, Y. Li, K. Fan and L. Sun, Pyrrolic N or pyridinic N: The active center of N-doped carbon for CO2 reduction, Chin. J. Catal., 2022, 43, 2405–2413 CrossRef CAS.
- B. Wei, J. Hao, B. Ge, W. Luo, Y. Chen, Y. Xiong, L. Li and W. Shi, Highly efficient electrochemical carbon dioxide reduction to syngas with tunable ratios over pyridinic-nitrogen rich ultrathin carbon nanosheets, J. Colloid Interface Sci., 2022, 608, 2650–2659 CrossRef CAS PubMed.
- J. Gui, K. Zhang, X. Zhan, Y. Yu, T. Huang, Y. Li, J. Xue, X. Jin, S. Gao and Y. Xie, Nitrogen-doped porous carbon nanosheets as a robust catalyst for tunable CO2 electroreduction to syngas, Sustain. Energy Fuels, 2022, 6, 1512–1518 RSC.
- X. Ma, L. Shi, L. Zhang, C. Hu and D. Liu, Tuning B–N pairs in porous carbon nanorods for electrochemical conversion of CO2 to syngas with controllable CO/H2 ratios, Sustain. Energy Fuels, 2023, 7, 661–670 RSC.
- Y. Wang, C. Niu, Y. Zhu, D. He and W. Huang, Tunable Syngas Formation from Electrochemical CO2 Reduction on Copper Nanowire Arrays, ACS Appl. Energy Mater., 2020, 3, 9841–9847 CrossRef CAS.
- I. Hjorth, Y. Wang, Y. Li, M. E. M. Buan, M. Nord, M. Rønning, J. Yang and D. Chen, Electrochemical syngas production from CO2 and water with CNT supported ZnO catalysts, Catal. Today, 2021, 364, 172–181 CrossRef CAS.
- H. Li, J. Gao, J. Shan, Q. Du, Y. Zhang, X. Guo, M. Xie, S. Wu and Z. Wang, Effect of halogen-modification on Ag catalyst for CO2 electrochemical reduction to syngas from NH4HCO3 electrolyte, J. Environ. Chem. Eng., 2021, 9, 106415 CrossRef CAS.
- H. Chen, Z. Li, Z. Zhang, K. Jie, J. Li, H. Li, S. Mao, D. Wang, X. Lu and J. Fu, Synthesis of Composition-Tunable Syngas from Efficiently Electrochemical Conversion of CO2 over AuCu/CNT Bimetallic Catalyst, Ind. Eng. Chem. Res., 2019, 58, 15425–15431 CrossRef CAS.
- M. B. Ross, C. T. Dinh, Y. Li, D. Kim, P. De Luna, E. H. Sargent and P. Yang, Tunable Cu Enrichment Enables Designer Syngas Electrosynthesis from CO2, J. Am. Chem. Soc., 2017, 139, 9359–9363 CrossRef CAS PubMed.
- M. B. Ross, Y. Li, P. De Luna, D. Kim, E. H. Sargent and P. Yang, Electrocatalytic Rate Alignment Enhances Syngas Generation, Joule, 2019, 3, 257–264 CrossRef CAS.
- Z. Guo, Q. Zhang, F. Shen, H. Liu, H. Zhang, Z. Guo, B. Jin and R. Peng, Boosting electron transport over controllable N ligand doping for electrochemical conversion of CO2 to syngas, Electrochim. Acta, 2021, 388, 138647 CrossRef CAS.
- C. Ye, X. Yu, W. Li, L. He, G. Hao and A. Lu, Engineering of Bifunctional Nickel Phosphide@Ni-N-C Catalysts for Selective Electroreduction of CO2-H2O to Syngas, Acta Phys.-Chim. Sin., 2022, 38, 2004054 Search PubMed.
- R. Daiyan, R. Chen, P. Kumar, N. M. Bedford, J. Qu, J. M. Cairney, X. Lu and R. Amal, Tunable Syngas Production through CO2 Electroreduction on Cobalt–Carbon Composite Electrocatalyst, ACS Appl. Mater. Interfaces, 2020, 12, 9307–9315 CrossRef CAS PubMed.
|
This journal is © the Partner Organisations 2023 |
Click here to see how this site uses Cookies. View our privacy policy here.