DOI:
10.1039/D1TC04893G
(Paper)
J. Mater. Chem. C, 2022,
10, 4805-4812
Aromatic-imide-based TADF enantiomers for efficient circularly polarized electroluminescence†
Received
13th October 2021
, Accepted 1st January 2022
First published on 4th January 2022
Abstract
Recently, much attention has been paid to circularly polarized organic light-emitting diodes (CP-OLEDs) based on thermally activated delayed fluorescence (TADF) enantiomers. In this paper, we designed and synthesized a pair of aromatic-imide-based TADF enantiomers, namely R/S-OBN-AICz, using chiral perturbation strategy. The chiral emitters concurrently demonstrated efficient TADF and aggregation-induced emission (AIE) properties, together with a small singlet–triplet energy gap (ΔEST) of 0.08 eV and a high photoluminescence quantum yield (PLQY) of 81%. Moreover, the TADF enantiomers exhibited clear circularly polarized luminescence (CPL) activities with dissymmetry factor (|gPL|) values of up to 2.6 × 10−3 in toluene solution. Finally, the TADF enantiomers were utilized as emitters to fabricate CP-OLEDs, which achieved clear circularly polarized electroluminescence (CPEL) signals, a high maximum external quantum efficiency (EQEmax) of 19.0% and a high maximum luminance (Lmax) of 24
790 cd m−2.
Introduction
Recently, circularly polarized luminescence (CPL) materials have attracted more and more attention for their extensive application prospects in the fields of anti-glare displays, 3D displays, encrypted transmission, optical communications, optical recognition sensing, quantum computing, and so on.1 So far, CP photoluminescence (CPPL) has been extensively studied, but CP electroluminescence (CPEL), a more valuable property for optoelectronic technology in the future, is still in its infancy.2 The organic light-emitting diode (OLED), as one of the most successful electroluminescent devices, has many advantages such as high resolution, high flexibility, high electroluminescence efficiency and low power consumption.3 Therefore, circularly polarized OLEDs (CP-OLEDs) fabricated with a chiral emitter are considered to be a promising strategy for realizing CPEL.4 Since the first case of CP-OLEDs was reported in 1997,5 a variety of chiral emitters suitable for CP-OLEDs have been developed, including chiral fluorescent emitters,6 chiral phosphorescent emitters,7 and chiral thermally activated delayed fluorescent (TADF) emitters.8 Compared with the first two kinds of chiral emitter, chiral TADF emitters have been more widely used to fabricate efficient CP-OLEDs in recent years9 owing to their purely organic molecular structures and theoretical 100% internal quantum efficiencies.10 Thus, the development of new chiral TADF molecules is the key issue for obtaining highly efficient CPEL.
The chiral perturbation strategy, which directly combines chiral units and TADF skeletons, is the main method used to design chiral TADF emitters. Thus, it is important to develop such proper chiral moieties and efficient TADF moieties. For chiral moieties, three common moieties, including cyclohexanediamine,11 binaphthol12 and octahydro-binaphthol (OBN), have been used.13 Among these, OBN is an excellent chiral unit due to its good solubility and chiral stability. Zheng's group13a,b developed two pairs of TADF enantiomers by merging chiral OBN moieties with effective cyan acceptor-based TADF skeletons and achieved CPEL and high efficiencies in both doped and non-doped CP-OLEDs. Later, Li et al.13c introduced OBN units into the multiple resonance TADF units to achieve CPEL as well as narrowband emission. More recently, Tang and co-workers13d fabricated a series of red CP-OLEDs using chiral OBN-based TADF emitters. Therefore, it is feasible and convenient to construct chiral TADF molecules via the chiral perturbation strategy using OBN as a chiral moiety.
In recent years, we have devoted attention to expanding the chiral aromatic-imide family and have reported some successful attempts.14 In this work, (R/S)-OBN chiral units were introduced into aromatic-imide-based TADF skeletons to develop TADF enantiomers R/S-OBN-AICz. The obtained chiral aromatic-imide displayed a highly twisted conformation and a large torsion angle between the donor and acceptor, leading to a small singlet–triplet energy gap (ΔEST) of 0.08 eV and efficient TADF properties. Meanwhile, the TADF enantiomers showed intense CPL signals with dissymmetry factor (|gPL|) values of 2.6 × 10−3, a high photoluminescence quantum yield (PLQY) of 81%, as well as aggregation-induced emission (AIE) properties. Moreover, using the R/S-OBN-AICz enantiomers as emitters, the resulting CP-OLEDs exhibited an excellent device performance with maximum external quantum efficiency (EQEmax), current efficiency (CEmax), power efficiency (PEmax), and maximum luminance (Lmax) values of 19.0%, 55.9 cd A−1, 47.5 lm W−1, and 24790 cd m−2, respectively. Besides, the devices showed noticeable CPEL signals.
Results and discussion
Synthesis, theoretical calculations, single-crystal analysis and electrochemical properties
The synthetic routes to a pair of enantiomerically pure TADF emitters R/S-OBN-AICz are shown in Scheme 1. AI-4F was prepared according to the method reported by our group.15 Then, the R/S-OBN-AI2F intermediates were synthesized simultaneously through the nucleophilic substitution reaction of (R/S)-octahydro-binaphthol and AI-4F. The final products were obtained via nucleophilic substitution from carbazoles and the R/S-OBN-AI2F chiral intermediates. All the new molecules were characterized via their 1H NMR, 13C NMR and high-resolution mass spectra.
 |
| Scheme 1 Synthetic routes for the R/S-OBN-AICz enantiomers. | |
To gain insight into the electronic distributions and energy levels of OBN-AICz, density functional theory (DFT) calculations were performed using the Gaussian 09 package at the B3LYP/6-31G(d) level. As shown in Fig. 1, the lowest unoccupied molecular orbital (LUMO) occupied the aromatic-imide units, whereas the highest occupied molecular orbital (HOMO) was mainly localized on the electron-donor carbazole moieties. The clear separation of the HOMO and LUMO could be conductive to obtaining a small ΔEST, promoting the up-conversion process from the triplet (T1) to the singlet (S1) energy levels. Besides, the calculated HOMO and LUMO energy levels of OBN-AICz were −5.43 and −2.31 eV, respectively. The corresponding energy gap (Eg) between the HOMO and LUMO energy levels was calculated to be 3.12 eV. Although the chiral unit made no contribution to both the HOMOs and LUMOs, CD and CPL activities could be detected due to chiral perturbation.
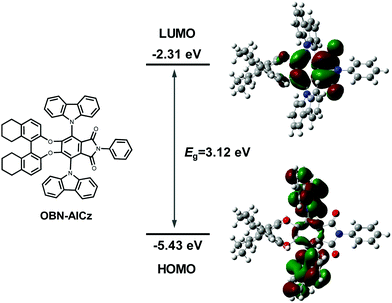 |
| Fig. 1 Chemical structures and calculated HOMO/LUMO distributions of OBN-AICz. | |
To further explore the structure of the obtained TADF enantiomers, the single-crystal structure of S-OBN-AICz was obtained successfully. As shown in Fig. 2 and Fig. S1 (ESI†), the absolute configurations, the intra- and intermolecular interactions, and the molecular packing modes of S-OBN-AICz were determined. As illustrated in Table S1 (ESI†), its single-crystal structure belongs to the monoclinic crystal system with the space group of P21. The crystal structure displayed a highly twisted conformation with dihedral angles between the two carbazoles and phenyl rings of 63.5° and 81.3°, respectively. Due to the asymmetry of the chiral moiety, the two carbazoles have different distances from their adjacent oxygen atoms on the OBN. The carbazole that is closer to the oxygen atom on OBN can form an intramolecular C–H⋯O hydrogen bond, while the other one that is farther away cannot form a hydrogen bond. The torsion angle of the carbazole can be limited by the C–H⋯O hydrogen bond between the oxygen atom on OBN and the C–H bond on the carbazole, leading to an obvious difference in dihedral angle. In addition, the large torsion angle between the donor and acceptor facilitates the effective spatial separation of the HOMO/LUMO, which is beneficial for achieving a small ΔEST value and efficient TADF properties.
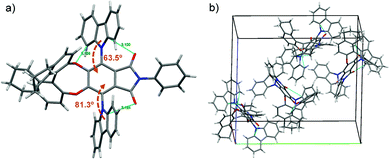 |
| Fig. 2 (a) Single-crystal structure and (b) molecular packing mode in the unit cell of S-OBN-AICz. | |
Then, the thermal stabilities of the enantiomers were studied using thermal gravimetric analysis (TGA) under a nitrogen atmosphere at a heating rate of 10 °C min−1 (Fig. S2, ESI†). The enantiomers displayed excellent thermal stability with a decomposition temperature (Td, corresponding to a 5% weight loss) of 419 °C for R-OBN-AICz. The glass transition temperature (Tg) is also one of the key material parameters of the emitter, which directly affects the process performance of the material. A high Tg can suppress crystallization or aggregation of the emitter during the deposition process, thereby forming a stable and uniform amorphous film. As shown in Fig. S3 (ESI†), the corresponding Tg was detected to be 220 °C using heating differential scanning calorimetry (DSC). The excellent thermal stabilities and morphological properties of the TADF enantiomers are necessary for fabricating OLED devices via vacuum evaporation.
The electrochemical properties of these TADF enantiomers were also studied using cyclic voltammetry (CV) in degassed anhydrous dichloromethane solvent. Owing to the almost identical properties of the enantiomers, R-OBN-AICz was chosen as the example (Fig. S4, ESI†). The HOMO energy levels were estimated to be −5.78 eV for R-OBN-AICz, from the onset of the oxidation spectrum with respect to that of ferrocene. Moreover, according to the absorption spectrum of the emitter in toluene, its corresponding Eg value was calculated to be 2.68 eV. Thus, the LUMO energy level of R-OBN-AICz was calculated to be −3.10 eV using the equation ELUMO = EHOMO + Eg. The suitable energy levels of this emitter in OLEDs are advantageous for charge injection (Table 1).
Table 1 Summary of photophysical, electrochemical and thermal properties of R-OBN-AICz
Compound |
λ
abs
[nm] |
λ
fl
[nm] |
Φ
PL
[%] |
τ
[μs] |
HOMO/LUMOe [eV] |
E
g
[eV] |
ΔEST [eV] |
T
d [°C] |
T
g [°C] |
Peaks of absorption spectra in toluene at room temperature.
Fluorescence spectra at room temperature measured in doped films.
Absolute PLQY measured in doped films using an integer sphere.
Delayed lifetimes were determined from the transient PL in doped films.
HOMO energy level measured from the oxidation potential in dichloromethane solution using cyclic voltammetry with ferrocene as the internal standard, and LUMO energy level calculated from EHOMO + Eg.
Estimated from the onset of the absorption spectrum.
|
R-OBN-AICz |
290, 318, 332, 397 |
509 |
81 |
4.0 |
−5.78/−3.10 |
2.68 |
0.08 |
419 |
220 |
Photophysical properties
The UV-vis absorption and steady-state photoluminescence (PL) spectra of the emitter were measured. Taking R-OBN-AICz as an example in various solvents (Fig. S5, ESI†), this enantiomer showed a broad absorption band in the range of 370–460 nm, which could be assigned to the intramolecular charge-transfer (ICT) process from the donor carbazole to the acceptor aromatic-imide. And the emitter also showed a strong absorption band near 332 nm, which was related to the π–π* transition of OBN and the carbazole units. Furthermore, with an increase of the solvent polarity, the absorption spectra of the compound exhibited a slight change, while the PL spectra were more sensitive to different solvents. The PL spectra of R-OBN-AICz displayed a remarkable red-shift from blue (467 nm) in hexane to yellow (550 nm) in acetonitrile (Fig. S6, ESI†), confirming its typical ICT character in the excited state. Besides, the PLQY of R-OBN-AICz in toluene solution was measured to be 57%.
Subsequently, the AIE and twisted ICT properties of R-OBN-AICz were studied in THF/H2O mixtures with various deionized water ratio fractions (fw). As shown in Fig. 3c, R-OBN-AICz displayed the relatively weak PL emission in pure THF solution. Then, as the proportion of water in the system was increased from 0% to 60%, the emission intensity decreased dramatically and the emission peaks were red-shifted. This phenomenon might be attributed to the strong twisted ICT process and increased ISC rate caused by the increased polarity of the solvent. When the water fraction continued to be increased from fw = 70% to fw = 90%, a significant PL emission enhancement and blue-shifted emission peaks were observed. As shown in Fig. 3d, the PL intensity of R-OBN-AICz showed increase of 2.1 times from pure THF to fw = 90% for the THF/H2O mixture. Owing to aggregate formation, the intramolecular rotational motion was sufficiently restricted and the local environment became less polar. Therefore, the non-radiative decay of the excited state energy was prevented and the radiative decay was promoted, leading to a strong PL emission. These results demonstrated the AIE properties of R-OBN-AICz.
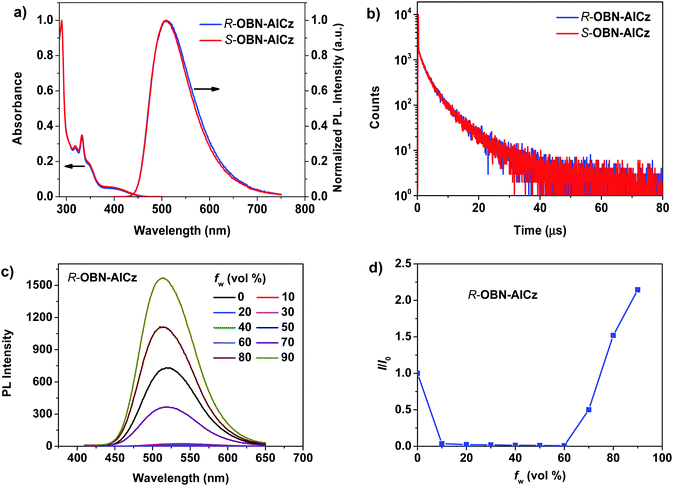 |
| Fig. 3 (a) UV–vis absorption in toluene and PL spectra of R/S-OBN-AICz in doped films. (b) Temperature-dependent transient decay curves of R/S-OBN-AICz in doped films. (c) PL spectra of R-OBN-AICz in THF/H2O mixtures with different water fraction (fw) values. (d) Plots of the relative PL peak intensity (I/I0) of the THF/H2O mixture of R-OBN-AICz. | |
The PL spectra of R-OBN-AICz in a neat film were then studied at 77 K. As illustrated in Fig. S7 (ESI†), the fluorescence and phosphorescence spectra of the emitter showed an emission band centered at 529 and 539 nm, respectively. According to the onset wavelengths of its fluorescence and phosphorescence spectra at 77 K, the ΔEST value of R-OBN-AICz was calculated to be 0.08 eV, which could facilitate the RISC process from T1 to S1, resulting in efficient TADF. In order to simulate the emissive behaviour in OLEDs, the basic photophysical properties of R-OBN-AICz in a 3,3-di(9H-carbazol-9-yl)biphenyl (mCBP) doped film were further measured. As illustrated in Fig. 3a, the 13 wt% R-OBN-AICz doped film exhibited a fluorescence peak centered at 509 nm, which has an obvious blue-shift compared with the pure film. And the doped film of R-OBN-AICz a high PLQY of 81% was demonstrated. Meanwhile, the transient PL decay curves of this doped film were also recorded at room temperature (Fig. 3b). It was found that the transient decay curve of R-OBN-AICz presented clear two exponential decay. The delayed lifetime of R-OBN-AICz was estimated to be 4.0 μs, which was related to the delayed fluorescence through RISC processes, illustrating its TADF properties. Moreover, the photophysical properties of S-OBN-AICz were also studied (Fig. 3a, b and Fig. S8, ESI†). It was found that the UV-vis absorption, PL and transient PL decay spectra of S-OBN-AICz were similar to those of R-OBN-AICz. These results demonstrate that chirality does not affect the molecular photophysical properties.
Chiroptical properties
We also investigated the chiroptical properties of R-OBN-AICz and S-OBN-AICz in the ground and excited states using electronic circular dichroism (ECD) and CPL spectra, respectively. As shown in Fig. 4a, the enantiomers exhibited a clear mirror-image ECD band in toluene. In the wavelength region below 325 nm, the strong Cotton effects could be attributed to the characteristic absorption of the chiral OBN moiety. Meanwhile, the weak Cotton effects in the long-wavelength region were assigned to the absorption of the ICT band from the carbazole donor to aromatic-imide moiety. These results revealed that the chirality was realized for these emitters through the chiral perturbation strategy. In addition, obvious mirror-image CPL spectra of R-OBN-AICz and S-OBN-AICz in toluene indicated their chiroptical properties in the excited state. As illustrated in Fig. 4b, R-OBN-AICz and S-OBN-AICz exhibited positive and negative CPL signals with gPL values of +2.6 × 10−3 and −2.4 × 10−3, respectively. These results indicate that the chiral perturbation strategy successfully imparts chirality to the TADF emitters. Moreover, the CPL signals of the enantiomers were also observed with gPL values of +2.6 × 10−3 for R-OBN-AICz and −2.4 × 10−3 for S-OBN-AICz in doped films (Fig. S11 and S12, ESI†).
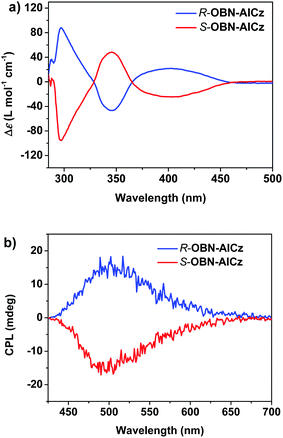 |
| Fig. 4 (a) ECD and (b) CPL spectra of R-OBN-AICz and S-OBN-AICz in toluene. | |
Electroluminescence properties
The electroluminescence (EL) properties of R-OBN-AICz and S-OBN-AICz were evaluated using mCBP as the host matrix of the emitting-layer (EML). First of all, four devices A–D were fabricated using 1,3,5-tri[(3-pyridyl)-phen-3-yl]benzene (TmPyPB), 1,3,5-tris(1-phenyl-1H-benzimidazol-2-yl)benzene (TPBi), 4,7-diphenyl-1,10-phenanthroline (Bphen) and 1,3-bis(3,5-dipyrid-3-ylphenyl)benzene (B3PYPB), respectively, as different electron-transporting layer (ETL) materials. These four series of OLED devices adopted the configurations of ITO/TAPC (40 nm)/mCBP (10 nm)/mCBP:13 wt% R-OBN-AICz (20 nm)/ETL (45 nm)/LiF (1 nm)/Al (100 nm). In these devices, TAPC (1,1-bis[(di-4-tolylamino)phenyl]cyclohexane) was used as the hole-transporting material, and mCBP was utilized as the exciton-blocking layer to confine the excitons within the EML. The results of their device performance are illustrated in Fig. S14–S18 (ESI†) and the relevant parameters are summarized in Table S2 (ESI†). Compared with the other three groups of devices, device A with TmPyPB as the ETL obtained the best overall performance with EQEmax = 17.5% and Lmax = 11
610 cd m−2.
We screened the thickness of each layer in the TmPyPB-based OLED devices to further optimize the device structures (Fig. S19–S23 and Table S3, ESI†). Finally, the best devices were obtained with the following device configurations: ITO/TAPC (40 nm)/mCBP (10 nm)/mCBP:13 wt% R/S-OBN-AICz (15 nm)/TmPyPB (50 nm)/LiF (1 nm)/Al (100 nm) (Fig. 5a). The device performances including the current density–voltage–luminance characteristics, EL spectra, and efficiency versus luminance of the OLED devices are illustrated in Fig. 5b–d and Fig. S24–S26 (ESI†), and the detailed device performance parameters are summarized in Table 2. As shown in Fig. 5c, the R-OBN-AICz-based OLEDs could emit EL emission with the peak centered at 514 nm and Commission Internationale de l’Éclairage (CIE) color coordinates of (0.27, 0.52). Furthermore, an EQEmax of 19.0%, a CEmax of 55.9 cd A−1, a PEmax of 47.5 lm W−1, and an Lmax of 24
790 cd m−2 were achieved in the R-OBN-AICz-based OLED devices. Similarly, the S-OBN-AICz-based OLED devices showed EQEmax, CEmax, PEmax, and Lmax values of 18.7%, 56.7 cd A−1, 45.7 lm W−1, and 21470 cd m−2, respectively. It was further found that the corresponding devices exhibited a low turn-on voltage of 3.7 V for the R-OBN-AICz-based OLED and 3.4 V for the S-OBN-AICz-based OLED at a luminance of 1 cd m−2, indicating efficient carrier injection and transport.
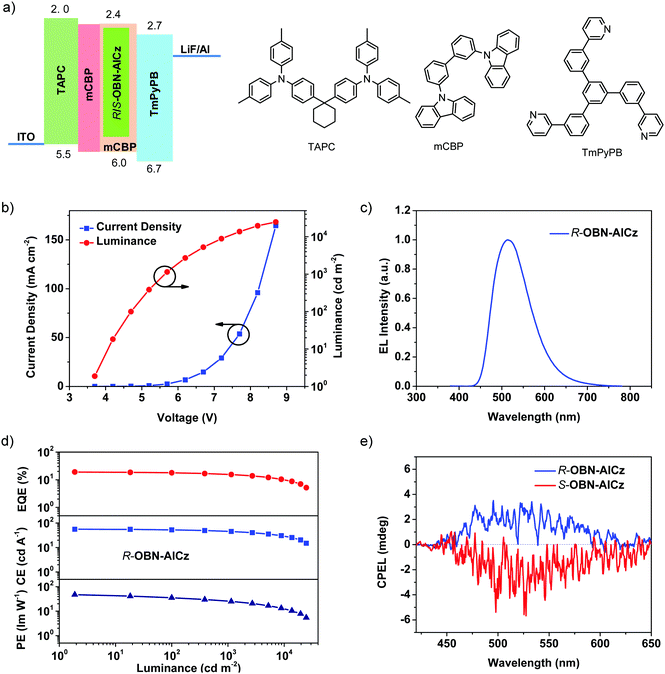 |
| Fig. 5 (a) Device structure, energy level diagram and molecular structures of the materials employed in the optimized devices. (b) Current density–voltage–luminance (J–V–L) characteristics, (c) EL spectra, and (d) efficiency–luminance characteristics of the optimized R/S-OBN-AICz-based OLEDs. (e) CPEL spectra of the CP-OLEDs based on R-OBN-AICz and S-OBN-AICz. | |
Table 2 EL performances of the optimized R/S-OBN-AICz-based OLEDs
Emitter |
V
turn-on
[V] |
λ
EL
[nm] |
EQEmaxc [%] |
CEmaxd [cd A−1] |
PEmaxe [lm W−1] |
L
max
[cd m−2] |
Turn-on voltage at 1 cd m−2.
EL peak wavelength.
Maximum external quantum efficiency (EQE).
Maximum current efficiency (CE).
Maximum power efficiency (PE).
Maximum luminance.
|
R-OBN-AICz |
3.7 |
514 |
19.0 |
55.9 |
47.5 |
24 790 |
S-OBN-AICz |
3.4 |
514 |
18.7 |
56.7 |
45.7 |
21 470 |
Considering the CPL properties of the enantiomers, the CP-OLEDs were fabricated using R-OBN-AICz and S-OBN-AICz as emitters to investigate the CPEL characteristics. As illustrated in Fig. 5e, the CP-OLEDs demonstrated clear mirror-image CPEL activities, keeping the direction consistent with the CPL signals. And these devices displayed opposing electroluminescence dissymmetry factor (gEL) values of +4.7 × 10−4 and −6.5 × 10−4 for the R-OBN-AICz- and S-OBN-AICz-based devices, respectively. The above results confirmed that the direct combination of chiral moieties and efficient TADF moieties could make the emitters produce CP signals in both photoluminescence and electroluminescence processes.
Conclusions
In summary, we have successfully developed a pair of aromatic-imide-based TADF enantiomers, namely R-OBN-AICz and S-OBN-AICz, by directly combining chiral units with TADF skeletons. These enantiomers displayed excellent TADF properties with a small ΔEST value of low to 0.08 eV, a high PLQY of up to 81%, as well as obvious AIE properties. Moreover, the TADF enantiomers exhibited clear mirror-image CD and CPL activities with |gPL| values of up to 2.6 × 10−3. Finally, high-efficiency CP-OLEDs were fabricated by adopting R-OBN-AICz and S-OBN-AICz as emitters. The fabricated CP-OLEDs showed a high EQEmax of 19.0% and an Lmax of 24
790 cd m−2. Notably, CPEL activities with opposing gEL signals were detected in the CP-OLEDs based on R/S-OBN-AICz. These results can provide a convenient method to develop aromatic-imide-based TADF enantiomers, which will be helpful for the further development of high-efficiency CPEL materials.
Experimental section
The synthetic routes to the pair of TADF enantiomers R/S-OBN-AICz are outlined in Scheme 1. All experiments were performed under an argon atmosphere. All reagents were purchased from commercial sources and used without further purification. Compound AI4F was synthesized according to the literature.15
Preparation procedure for R-OBN-AI2F
A solution of AI-4F (3.0 g, 10.2 mmol), R-5,5′,6,6′,7,7′,8,8′-octahydro-1,1′-2-naphthol (3.0 g, 10.2 mmol) and potassium carbonate (2.9 g, 21.0 mmol) in dry DMF (40.0 mL) was stirred for 12 h at 60 °C. After cooling to room temperature, the mixture was extracted using ethyl acetate and washed with saturated brine. The combined organic layer was dried with anhydrous MgSO4 and filtered, then concentrated to obtain the crude compound. Further purification was carried out via column chromatography on silica gel using CH2Cl2/petroleum ether as the eluent to afford a white powder with a yield of 64%. 1H NMR (500 MHz, CDCl3): δ 7.49 (t, J = 7.7 Hz, 2H), 7.43–7.36 (m, 3H), 7.14 (d, J = 8.3 Hz, 2H), 6.99 (d, J = 8.2 Hz, 2H), 2.89–2.78 (m, 4H), 2.72–2.61 (m, 2H), 2.43–2.39 (m, 2H), 1.84–1.81 (m, 6H), 1.68–1.64 (m, 2H). 13C NMR (126 MHz, CDCl3): δ 162.7, 149.6, 148.3, 146.2, 145.88, 145.85, 137.3, 136.4, 131.0, 130.6, 129.4, 129.2, 128.4, 126.5, 118.3, 112.0, 29.3, 27.6, 22.6, 22.5. HRMS m/z calcd for C34H26O4NF2 [M + H]: 550.1824, found: 550.1814.
Preparation procedure for R-OBN-AICz
A solution of carbazoles (1.5 g, 9.0 mmol) in dry DMF (20.0 mL) was stirred for 5 min at room temperature, and then sodium hydride (0.24 g, 10.0 mmol) was added to the solution. After another 30 min, R-OBN-AI2F (2.2 g, 4.0 mmol) was added to the mixture and stirred for 12 h at room temperature. Then the suspension mixture was poured into water, and the aqueous layer was extracted using ethyl acetate. The combined organic layers were washed with saturated brine and concentrated under vacuum. Further purification was carried out via column chromatography on silica gel using CH2Cl2/petroleum ether as the eluent to afford a green powder with a yield of 72%. 1H NMR (500 MHz, CDCl3): δ 8.19 (d, J = 7.5 Hz, 2H), 8.13 (d, J = 6.9 Hz, 2H), 7.50 (t, J = 7.1 Hz, 2H), 7.36 (t, J = 7.2 Hz, 2H), 7.32–7.26 (m, 5H), 7.24–7.19 (m, 3H), 7.16 (d, J = 7.7 Hz, 3H), 6.90 (d, J = 7.7 Hz, 4H), 6.32 (d, J = 8.1 Hz, 2H), 2.74–2.72 (m, 4H), 2.47–2.44 (m, 2H), 2.18–2.15 (m, 2H), 1.74–1.70 (m, 6H), 1.50–1.48 (m, 2H). 13C NMR (126 MHz, CDCl3): δ 162.9, 154.7, 149.8, 141.2, 141.0, 137.1, 135.8, 131.1, 130.1, 129.3, 128.6, 127.9, 127.7, 126.2, 126.0, 125.8, 124.4, 124.0, 120.8, 120.6, 120.43, 120.35, 117.9, 110.3, 109.6, 29.2, 27.4, 22.6, 22.4. HRMS m/z calcd for C58H42N3O4 [M + H]: 844.3170, found: 844.3157.
S-OBN-AICz was prepared using the above synthetic routes. 1H NMR (500 MHz, CDCl3): δ 8.19 (d, J = 7.7 Hz, 2H), 8.14 (d, J = 7.3 Hz, 2H), 7.50 (t, J = 7.5 Hz, 2H), 7.36 (t, J = 7.4 Hz, 2H), 7.32–7.26 (m, 5H), 7.24–7.20 (m, 3H), 7.17 (d, J = 8.0 Hz, 3H), 6.90 (d, J = 7.9 Hz, 4H), 6.33 (d, J = 8.2 Hz, 2H), 2.75–2.73 (m, 4H), 2.49–2.43 (m, 2H), 2.20–2.15 (m, 2H), 1.75–1.69 (m, 6H), 1.51–1.47 (m, 2H). 13C NMR (126 MHz, CDCl3): δ 162.9, 154.7, 149.8, 141.2, 141.0, 137.1, 135.8, 131.1, 130.1, 129.3, 128.6, 127.9, 127.7, 126.2, 126.0, 125.8, 124.4, 124.0, 120.8, 120.6, 120.42, 120.35, 117.9, 110.3, 109.6, 29.2, 27.4, 22.6, 22.4. HRMS m/z calcd for C58H42N3O4 [M + H]: 844.3170, found: 844.3154.
Author contributions
This manuscript was written through the contributions of all authors. All authors have given approval to the final version of the manuscript.
Conflicts of interest
There are no conflicts to declare.
Acknowledgements
We thank the National Natural Science Foundation of China (22122111, 91956119, and 92056109), the Beijing National Laboratory for Molecular Sciences (BNLMS-CXXM-202105) and the Youth Innovation Promotion Association CAS (2019034) for financial support.
References
-
(a) Y. Zhang and G. B. Schuster, J. Org. Chem., 1995, 60, 7192 CrossRef CAS;
(b) W. F. Jager, B. D. Lange and B. L. Feringa, Science, 1996, 273, 1686 CrossRef;
(c) J. Sherson, H. Krauter, R. Olsson, B. Julasgaard, K. Hammerer, I. Cirac and E. Polzik, Nature, 2006, 443, 557 CrossRef CAS PubMed;
(d) S. Relaix, C. Bourgerette and M. Mitov, Appl. Phys. Lett., 2006, 89, 251907 CrossRef;
(e) M. C. Heffern, L. M. Matosziuk and T. J. Meade, Chem. Rev., 2014, 114, 4496 CrossRef CAS PubMed;
(f) J. Kumar, T. Nakashima and T. Kawai, J. Phys. Chem. Lett., 2015, 6, 3445 CrossRef CAS PubMed;
(g) R. Clarke, K. Ho, A. Alsimaree, O. Woodford, P. Waddell, J. Bogaerts, W. Herrebout, J. Knight, R. Pal, T. Penfold and M. Hall, ChemPhotoChem, 2017, 1, 513 CrossRef CAS;
(h) J. Yan, F. Ota, B. A. San Jose and K. Akagi, Adv. Funct. Mater., 2017, 27, 1604529 CrossRef.
-
(a) R. Carr, N. H. Evans and D. Parker, Chem. Soc. Rev., 2012, 41, 7673 RSC;
(b) M. C. Heffern, L. M. Matosziuk and T. J. Meade, Chem. Rev., 2014, 114, 4496 CrossRef CAS PubMed;
(c) E. Yashima, N. Ousaka, D. Taura, K. Shimomura, T. Ikai and K. Maeda, Chem. Rev., 2016, 116, 13752 CrossRef CAS PubMed;
(d) M. Li, W.-B. Lin, L. Fang and C.-F. Chen, Acta Chim. Sin., 2017, 75, 1150 CrossRef.
-
(a) Z. Yang, Z. Mao, Z. Xie, Y. Zhang, S. Liu, J. Zhao, J. Xu, Z. Chi and M. P. Aldred, Chem. Soc. Rev., 2017, 46, 915 RSC;
(b) M. Y. Won and E. Zysman-Colman, Adv. Mater., 2017, 29, 1605444 CrossRef PubMed;
(c) Y. Liu, C. Li, Z. Ren, S. Yan and M. R. Bryce, Nat. Rev. Mater., 2018, 3, 18020 CrossRef CAS;
(d) S. K. Jeon, H. Lee, K. S. Yook and J. Y. Lee, Adv. Mater., 2019, 31, 1803524 CrossRef PubMed.
-
(a) J. R. Brandt, F. Salerno and M. J. Fuchter, Nat. Rev. Chem., 2017, 1, 0045 CrossRef CAS;
(b) D.-W. Zhang, M. Li and C.-F. Chen, Chem. Soc. Rev., 2020, 49, 1331 RSC;
(c) M. Li, M.-Y. Wang, Y.-F. Wang, L. Feng and C.-F. Chen, Angew. Chem., Int. Ed., 2021, 60, 20728 CrossRef CAS PubMed.
- E. Peeters, M. Christiaans, R. Janssen, H. Schoo, H. Dekkers and E. W. Meijer, J. Am. Chem. Soc., 1997, 119, 9909 CrossRef CAS.
-
(a) D.-M. Lee, J.-W. Song, Y.-J. Lee, C.-J. Yu and J.-H. Kim, Adv. Mater., 2017, 29, 1700907 CrossRef PubMed;
(b) J.-H. Jung, D.-M. Lee, J.-H. Kim and C.-J. Yu, J. Mater. Chem. C, 2018, 6, 726 RSC;
(c) L. Yang, Y. Zhang, X. Zhang, N. Li, Y. Quan and Y. Cheng, Chem. Commun., 2018, 54, 9663 RSC;
(d) Y. Zhang, X. Zhang, H. Zhang, Y. Xiao, Y. Quan, S. Ye and Y. Cheng, J. Phys. Chem. C, 2019, 123, 24746 CrossRef CAS;
(e) L. Wan, J. Wade, F. Salerno, O. Arteaga, B. Laidlaw, X. Wang, T. Penfold, M. J. Fuchter and A. J. Campbell, ACS Nano, 2019, 13, 8099 CrossRef CAS PubMed.
-
(a) T.-Y. Li, Y.-M. Jing, X. Liu, Y. Zhao, L. Shi, Z. Y. Tang, Y.-X. Zheng and J.-L. Zuo, Sci. Rep., 2015, 5, 14912 CrossRef CAS PubMed;
(b) F. Zinna, U. Giovanella and L. D. Bari, Adv. Mater., 2015, 27, 1791 CrossRef CAS PubMed;
(c) J. R. Brandt, X. Wang, Y. Yang, A. J. Campnell and M. J. Fuchter, J. Am. Chem. Soc., 2016, 138, 9743 CrossRef CAS PubMed;
(d) J. Han, S. Guo, J. Wang, L. Wei, Y. Zhuang, S. Liu, Q. Zhao, X. Zhang and W. Huang, Adv. Opt. Mater., 2017, 5, 1700359 CrossRef;
(e) Z.-P. Yan, K. Liao, H.-B. Han, J. Su, Y.-X. Zheng and J.-L. Zuo, Chem. Commun., 2019, 55, 8215 RSC;
(f) Z.-P. Yan, X.-F. Luo, W.-Q. Liu, Z.-G. Wu, X. Liang, K. Liao, Y. Wang, Y.-X. Zheng, L. Zhou, J.-L. Zuo, Y. Pan and H. Zhang, Chem. – Eur. J., 2019, 25, 5672 CrossRef CAS PubMed;
(g) G. Fu, Y. He, W. Li, B. Wang, X. Lü, H. He and W.-Y. Wong, J. Mater. Chem. C, 2019, 7, 13743 RSC;
(h) Y. Chen, X. Li, N. Li, Y. Quan, Y. Cheng and Y. Tang, Mater. Chem. Front., 2019, 3, 867 RSC.
-
(a) Y. Wang, Y. Zhang, W. Hu, Y. Quan, Y. Li and Y. Cheng, ACS Appl. Mater. Interfaces, 2019, 11, 26165 CrossRef CAS PubMed;
(b) M. Li, Y.-F. Wang, D. Zhang, L. Duan and C.-F. Chen, Angew. Chem., Int. Ed., 2020, 59, 3500 CrossRef CAS PubMed;
(c) Z.-L. Tu, Z.-P. Yan, X. Liang, L. Chen, Z.-G. Wu, Y. Wang, Y.-X. Zheng, J.-L. Zuo and Y. Pan, Adv. Sci., 2020, 7, 2000804 CrossRef CAS PubMed;
(d) S.-Y. Yang, Y.-K. Wang, C.-C. Peng, Z.-G. Wu, S. Yuan, Y.-J. Yu, H. Li, T.-T. Wang, H.-C. Li, Y.-X. Zheng, Z.-Q. Jiang and L.-S. Liao, J. Am. Chem. Soc., 2020, 142, 17756 CrossRef CAS PubMed;
(e) F. Ni, C.-W. Huang, Y. Tang, Z. Chen, Y. Wu, S. Xia, X. Cao, J.-H. Hsu, W.-K. Lee, K. Zheng, Z. Huang, C.-C. Wu and C. Yang, Mater. Horiz., 2021, 8, 547 RSC;
(f) Y.-F. Wang, M. Li, J.-M. Teng, H.-Y. Zhou and C.-F. Chen, Adv. Funct. Mater., 2021, 31, 2106418 CrossRef CAS;
(g) Y.-F. Wang, M. Li, J.-M. Teng, H.-Y. Zhou, W.-L. Zhao and C.-F. Chen, Angew. Chem., Int. Ed., 2021, 60, 23619 CrossRef CAS PubMed.
- L. Frédéric, A. Desmarchelier, L. Favereau and G. Pieters, Adv. Funct. Mater., 2021, 31, 2010281 CrossRef.
-
(a) M. Li, Y. Liu, R. Duan, X. Wei, Y. Yi, Y. Wang and C.-F. Chen, Angew. Chem., Int. Ed., 2017, 56, 8818 CrossRef CAS PubMed;
(b) M. Li, Y.-F. Wang, D.-W. Zhang, D. Zhang, Z.-Q. Hu, L. Duan and C.-F. Chen, Sci. China Mater., 2021, 64, 899 CrossRef CAS;
(c) L. Zhang, Y.-F. Wang, M. Li, Q.-Y. Gao and C.-F. Chen, Chin. Chem. Lett., 2021, 32, 740 CrossRef CAS.
-
(a) M. Li, S.-H. Li, D. Zhang, M. Cai, L. Duan, M.-K. Fung and C.-F. Chen, Angew. Chem., Int. Ed., 2018, 57, 2889 CrossRef CAS PubMed;
(b) Y.-F. Wang, H.-Y. Lu, C. Chen, M. Li and C.-F. Chen, Org. Electron., 2019, 70, 71 CrossRef CAS.
-
(a) F. Song, Z. Xu, Q. Zhang, Z. Zhao, H. Zhang, W. Zhao, Z. Qiu, C. Qi, H. Zhang, H. H. Y. Sung, I. D. Williams, J. W. Y. Lam, Z. Zhao, A. Qin, D. Ma and B. Z. Tang, Adv. Funct. Mater., 2018, 28, 1800051 CrossRef;
(b) S. Sun, J. Wang, L. Chen, R. Chen, J. Jin, C. Chen, S. Chen, G. Xie, C. Zheng and W. Huang, J. Mater. Chem. C, 2019, 7, 14511 RSC;
(c) L. Frédéric, A. Desmarchelier, R. Plais, L. Lavnevich, G. Muller, C. Villafuerte, G. Clavier, E. Quesnel, B. Racine, S. Meunier-Della-Gatta, J.-P. Dognon, P. Thuéry, J. Crassous, L. Favereau and G. Pieters, Adv. Funct. Mater., 2020, 30, 2004838 CrossRef PubMed.
-
(a) Z.-G. Wu, H. B. Han, Z.-P. Yan, X.-F. Luo, Y. Wang, Y.-X. Zheng, J.-L. Zuo and Y. Pan, Adv. Mater., 2019, 31, 1900524 CrossRef PubMed;
(b) Z.-G. Wu, Z.-P. Yan, X.-F. Luo, L. Yuan, W.-Q. Liang, Y. Wang, Y.-X. Zheng, J.-L. Zuo and Y. Pan, J. Mater. Chem. C, 2019, 7, 7045 RSC;
(c) Y. Xu, Q. Wang, X. Cai, C. Li and Y. Wang, Adv. Mater., 2021, 33, 2100652 CrossRef CAS PubMed;
(d) F.-M. Xie, J.-X. Zhou, X.-Y. Zeng, Z.-D. An, Y.-Q. Li, D.-X. Han, P.-F. Duan, Z.-G. Wu, Y.-X. Zheng and J.-X. Tang, Adv. Opt. Mater., 2021, 9, 2100017 CrossRef CAS.
- Y.-F. Wang, C. Chen, L. Cui, J.-M. Teng, M. Li, H.-Y. Lu and C.-F. Chen, Org. Electron., 2021, 99, 106355 CrossRef CAS.
- X. Liu, Y.-F. Wang, M. Li, Y. Zhu and C.-F. Chen, Org. Electron., 2021, 88, 106017 CrossRef CAS.
Footnotes |
† Electronic supplementary information (ESI) available. CCDC 2107122. For ESI and crystallographic data in CIF or other electronic format see DOI: 10.1039/d1tc04893g |
‡ The authors contributed equally to the creation of this work. |
|
This journal is © The Royal Society of Chemistry 2022 |