DOI:
10.1039/D2QI01694J
(Research Article)
Inorg. Chem. Front., 2022,
9, 5820-5827
Interesting dimensional transition through changing cations as the trigger in multinary thioarsenates displaying variable photocurrent response and optical anisotropy†
Received
4th August 2022
, Accepted 19th September 2022
First published on 20th September 2022
Abstract
Due to the intriguing component variability and structure–property flexibility, lone-pair cation-based chalcogenides have garnered substantial interest in recent years. Herein, two new multinary thioarsenates, Cs2ZnAs4S8 and [(NH4)Cs]CdAs4S8, were successfully discovered via a surfactant–thermal reaction. Both of them possess identical stoichiometry 2–1–4–8, but they exhibit surprisingly different structural features. Cs2ZnAs4S8 demonstrates a three-dimensional (3D) [ZnAs4S8]2− framework made from the corner-sharing [ZnS4] tetrahedra and one-dimensional (1D) [As4S8]4− chains, whereas [(NH4)Cs]CdAs4S8 exhibits a two-dimensional (2D) [CdAs4S8]2− layer constructed from the corner-sharing [CdS4] tetrahedra and tetranuclear [As4S8] clusters. Photoelectric measurements display that Cs2ZnAs4S8 has higher photogenerated electron–hole pair separation efficiency than [(NH4)Cs]CdAs4S8 under visible light irradiation. Moreover, both of them show large optical anisotropy (Δn > 0.17 at 1064 and 2050 nm), while the low dimensional structure is more conducive to enhancing the optical anisotropy based on the theoretical calculations. These findings will provide inspiration for the exploration of multifunctional chalcogenides.
Introduction
Over the past few decades, inorganic chalcogenides containing [MIIIQn] (MIII = As, Sb, Bi) polyhedra have attracted increasing attention because of their intriguing structural and compositional diversity.1–15 These MIII cations with stereochemically active lone-pair electrons are favorable to form different asymmetric coordination modes, which will further influence the electronic structures and physical properties of the resultant chalcogenides. For example, M2As2Q5 (M = Ba, Pb; Q = S, Se) exhibit excellent overall infrared nonlinear optical (IR-NLO) performance thanks to their zero-dimensional (0D) discrete arsenate anions.12 Pentanary thioantimonate Rb2Ba3Cu2Sb2S10 adopts a one-dimensional (1D) chain structure and displays a wide-bandgap and an intriguing photocurrent response.9 The narrow gap semiconductor RbBi11/3Te6, which consists of a two-dimensional (2D) infinite Bi2Te3-like layer, exhibits a sharp superconducting transition at ∼3.2 K.4 The three-dimensional (3D) frameworks A3Mn2Sb3S8 (A = K and Rb) not only display IR-NLO performances but also possess temperature-dependent paramagnetism and photocurrent responses.13 On the other hand, transition-metal-based chalcogenides with d10 electronic configurations (e.g., Zn2+, Cd2+, Hg2+) have also been extensively investigated as these cations tend to show flexible coordination geometries, and hence engender an efficient route to design functional materials.16 For instance, Na6Zn3M2Q9 (M = Ga, In; Q = S, Se), 2D layered chalcogenides made of unprecedented T3-supertetrahedra, exhibit desirable photoluminescence performances.17 KCd3Ga5S11 adopts a diamond-like framework structure and achieves a strong second-harmonic-generation intensity (1.7 × AgGaS2).18 Ternary IR-NLO BaHgSe2 shows large susceptibility and physicochemical stability activated by the trigonal planar [HgSe3]4− motif.19
A tremendous amount of multinary lone-pair cation-based chalcogenides have been discovered so far, however, the systematic investigation of the A/TM/As/Q system (A = alkali metals; TM = group 12 metals; Q = chalcogen) is rarely reported. To the best of our knowledge, only 3 compounds in this system are known, namely NaCdAsS3,20 Rb4CdAs2S9,21 and CsHgAsS3.22 The first two are obtained by the solid-state method, while the last one is prepared by solvothermal synthesis. Recently, a surfactant–thermal reaction has been proved to be a facile method for exploring novel chalcogenides.23–31 In this study, our continuous explorations based on the surfactant–thermal method have led to the discovery of two new thioarsenates, Cs2ZnAs4S8 and [(NH4)Cs]CdAs4S8. Although these two compounds have identical stoichiometry 2–1–4–8, they are not isostructural and undergo an interesting dimensional transition from a 3D framework to a 2D layered structure. Herein, the crystal structure, physical properties, and corresponding theoretical studies are systematically investigated.
Results and discussion
Single-crystal X-ray diffraction (SXRD) test results show that Cs2ZnAs4S8 and [(NH4)Cs]CdAs4S8 belong to different space groups, i.e., tetragonal I41/a (no. 88) for Cs2ZnAs4S8 with cell parameters of a = b = 11.0828(5), c = 13.2303(8) Å, and Z = 4 and tetragonal P4/n (no. 85) for [(NH4)Cs]CdAs4S8 with cell parameters of a = b = 10.3879(2), c = 7.7691(2) Å, and Z = 2. The detailed crystallographic information is listed in Table 1. There are 1 unique Cs atom (Wyckoff site: 8e), 1 unique As atom (Wyckoff site: 16f), 1 unique Zn atom (Wyckoff site: 4a), and 2 unique S atoms (Wyckoff sites: 16f and 16f) in its asymmetric unit (Table 2). As illustrated in Fig. 1a, the three-dimensional (3D) crystal structure of Cs2ZnAs4S8 is constructed from charge-balanced Cs+ cations, regular [ZnS4] tetrahedra, (Fig. 1b) and one-dimensional (1D) anionic chains [As4S8]4− made of the corner-sharing [As4S9] groups (Fig. 1c). The important bond distances of Cs2ZnAs4S8 are listed in Table S1.† The As atom adopts a common coordination with 3 S atoms in the bond distance range of 2.2143(5)–2.3267(5) Å to build a [AsS3]3− triangular pyramid. The Zn–S bond distance in the [ZnS4] tetrahedron is 2.3309(5) Å. The S–As–S angles in Cs2ZnAs4S8 range from 98.07(2)° to 103.28(2)°, while the S–Zn–S angles vary from 105.79(2)° to 117.12(2)°. These bond distances and angles in Cs2ZnAs4S8 are normal and can also be comparable to the reported Cs-based chalcogenides.32–34
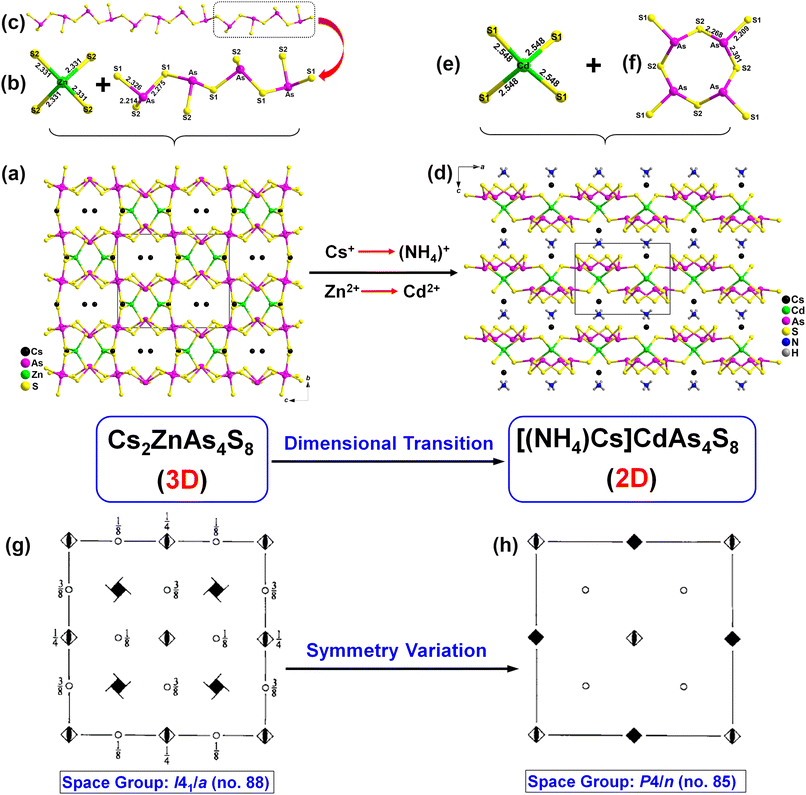 |
| Fig. 1 Dimensional transition from 3D Cs2ZnAs4S8 to 2D [(NH4)Cs]CdAs4S8 based on the isovalent cation substitution. (a) Crystal structure of 3D Cs2ZnAs4S8 viewed down the a-direction; (b) 1D [As4S8] chain with the coordination environment of the [As4S9] unit marked; (c) the coordination environment of the tetrahedral [ZnS4] unit; (d) crystal structure of 2D [(NH4)Cs]CdAs4S8 viewed down the a-direction; (e, f) the coordination environment of the tetrahedral [ZnS4] unit and tetra-nuclear [As4S8] cluster; (g, h) spatial symmetry operation from I41/a (no. 88) to P4/n (no. 85). | |
Table 1 Crystallographic data and refinement details of Cs2ZnAs4S8 and [(NH4)Cs]CdAs4S8
R1 = ∑||Fo| − |Fc||/∑|Fo|, wR2 = [∑w(Fo2 − Fc2)2/∑w(Fo2)2]1/2.
|
Formula |
Cs2ZnAs4S8 |
[(NH4)Cs]CdAs4S8 |
FW |
887.35 |
819.51 |
Crystal system |
Tetragonal |
Tetragonal |
Temperature (K) |
Yellow |
Orange |
Crystal color |
I41/a (no. 88) |
P4/n (no. 85) |
Space group |
11.0828(5) |
10.3879(2) |
a (Å) |
11.0828(5) |
10.3879(2) |
b (Å) |
13.2303(8) |
7.7691(2) |
c (Å) |
90 |
90 |
α (°) |
90 |
90 |
β (°) |
90 |
90 |
γ (°) |
1625.06(18) |
838.35(4) |
V (Å3) |
4 |
2 |
Z
|
3.627 |
3.246 |
D
c (g cm−3) |
15.004 |
12.243 |
μ (mm−1) |
1.039 |
1.071 |
GOOF on F2 |
0.0265, 0.0603 |
0.0152, 0.0300 |
R
1, wR2 (I > 2σ(I))a |
0.0369, 0.0645 |
0.0189, 0.0316 |
R
1, wR2 (all data) |
1.826/−1.271 |
0.818/−0.552 |
Largest diff. peak/hole (e Å−3) |
887.35 |
819.51 |
Table 2 Atomic coordinates and equivalent isotropic displacement parameters of Cs2ZnAs4S8 and [(NH4)Cs]CdAs4S8
Atom |
Wyckoff |
x
|
y
|
z
|
U
(eq) a |
Occu. |
U
(eq) is defined as one-third of the trace of the orthogonalized Uij tensor.
|
Cs
2
ZnAs
4
S
8
|
Cs |
8e |
0 |
0.25 |
0.45521(2) |
0.02597(6) |
1.0 |
As |
16f |
0.16243(2) |
0.01798(2) |
0.00487(2) |
0.01245(5) |
1.0 |
Zn |
4a |
0 |
0.25 |
0.125 |
0.01320(8) |
1.0 |
S2 |
16f |
0.17589(5) |
0.21445(4) |
0.03310(3) |
0.01605(9) |
1.0 |
S1 |
16f |
0.31599(5) |
0.51563(5) |
0.10909(3) |
0.01892(10) |
1.0 |
[(NH
4
)Cs]CdAs
4
S
8
|
Cs |
2c |
0.25 |
0.25 |
0.68028(3) |
0.02210(7) |
1.0 |
Cd |
2a |
0.25 |
0.75 |
0 |
0.01361(7) |
1.0 |
As |
8g |
0.04860(2) |
0.15875(2) |
0.14479(2) |
0.01204(6) |
1.0 |
S2 |
8g |
0.16315(4) |
0.02562(4) |
0.31813(6) |
0.01556(10) |
1.0 |
S1 |
8g |
0.07233(4) |
0.64420(4) |
0.17643(6) |
0.01402(9) |
1.0 |
N |
2b |
0.25 |
0.75 |
0.5 |
0.0082(5) |
1.0 |
H |
8g |
0.2013(19) |
0.724(2) |
0.446(3) |
0.014(6) |
1.0 |
There are 7 unique crystallographic atoms in the asymmetric unit of the structure of [(NH4)Cs]CdAs4S8, including 1 Cs (Wyckoff site: 2c), 1 Cd (Wyckoff site: 2a), 1 As (Wyckoff site: 8g), 2 S (Wyckoff sites: 8g and 8g), 1 N (Wyckoff site: 2b) and 1 H (Wyckoff site: 8g) (Tables 1 and 2). As given in Fig. 1d, the crystal structure of [(NH4)Cs]CdAs4S8 is composed of regular [CdS4] tetrahedra with d(Cd–S) = 2.5481(5) Å and tetra-nuclear [As4S8] clusters with d(As–S) = 2.2676(5)–2.3008(5) Å, which interconnect with each other by sharing vertexes to form a two-dimensional (2D) [CdAs4S8]2− layer filling the dispersed (NH4)+ and Cs+ cations.
The interesting structural evolution between Cs2ZnAs4S8 and [(NH4)Cs]CdAs4S8 is illustrated in Fig. 1. Both of them possess an identical stoichiometry of 2–1–4–8 and belong to the same tetragonal system, but they are not isostructural and have some significantly different structural features: (i) pyramidal [AsS3] units in Cs2ZnAs4S8 forms a 1D infinite [As4S8]4− chain by vertex-sharing S1 atoms, while in [(NH4)Cs]CdAs4S8, the tetra-nuclear [As4S8] cluster is constructed from the same [AsS3] units; (ii) the coordination number (CN) of the crystallographically independent Cs atom in Cs2ZnAs4S8 is 10 and the Cs–S bond distances are in the range of 3.617 (5)–4.116 (6) Å, which are different from those of [(NH4)Cs]CdAs4S8 [CN = 8, d(Cs–S) = 3.696(5)–3.76(5) Å] (Fig. S1†). Such differences between them can be attributed to the different sizes of cations, i.e., the 3D [ZnAs4S8]2− framework in Cs2ZnAs4S8 can be viewed as [ZnS4] tetrahedra connected by 1D infinite [As4S8]4− chains by sharing S atoms and relatively large Cs+ cations occupied the space of the framework. However, when one of the “large” radius Cs+ cations was replaced by smaller radius (NH4)+ cations, the linkages between the [As4S8]4− chains in Cs2ZnAs4S8 are broken, and the 3D [ZnAs4S8]2− framework in Cs2ZnAs4S8 transforms into the 0D [As4S8] clusters in [(NH4)Cs]CdAs4S8. In addition, the above-mentioned structural evolution is ultimately reflected by their space groups, from I41/a (for Cs2ZnAs4S8) to P4/n (for [(NH4)Cs]CdAs4S8). The detailed symmetric operation change based on isovalent cation substitution is shown in Fig. 1g and h.
Polycrystalline samples of Cs2ZnAs4S8 and [(NH4)Cs]CdAs4S8 were prepared by a facile surfactant–thermal method at 413 K for 7 days with CsOH·H2O, Zn (or Cd), As2S3, S, oleic acid, hydrazine monohydrate (98%) and PEG-400 as staring materials, in a yield of approximately 80–90% based on Zn (or Cd) (further experimental details see the ESI†). As displayed in Fig. S2 and S3,† semi-quantitative energy-dispersive X-ray (EDX) elemental analysis provides the average atomic ratios of 2.0/1.1(2)/3.9(8)/8.2(1) and 1.0/1.1(4)/4.0(5)/7.9(7) for Cs2ZnAs4S8 and [(NH4)Cs]CdAs4S8, respectively. In addition, the purity of the polycrystalline samples was confirmed by powder X-ray diffraction (XRD) analysis (Fig. S4 and S5†). Moreover, both of them exhibit desirable thermal stability (up to 700 K) under N2 conditions, as shown in Fig. S6 and S7.† Based on the different calculated formulas for direct semiconductors ((αhv)2vs. energy) or indirect semiconductors ((αhv)1/2vs. energy),35 the experimental energy gaps (Eg) of Cs2ZnAs4S8 and [(NH4)Cs]CdAs4S8 are about 2.24 and 2.36 eV, respectively (Fig. 2). These values are compared to those of other reported quaternary thioarsenates, such as RbCu4AsS4 (Eg = 2.15 eV),36 Cs3CuAs4S8 (Eg = 2.26 eV),30 Rb8Cu6As8S19 (Eg = 2.29 eV),37 and CsCu2AsS3 (Eg = 2.30 eV).27
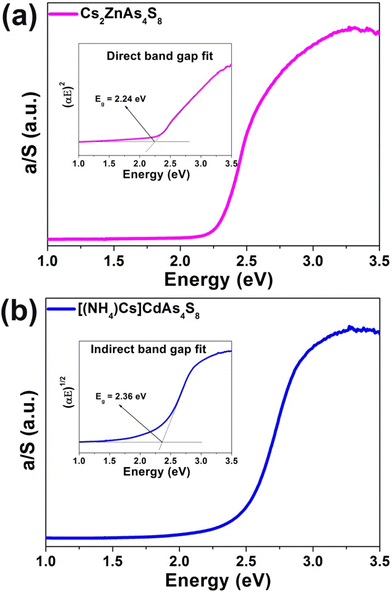 |
| Fig. 2 UV-vis-NIR diffuse reflectance spectra of (a) Cs2ZnAs4S8 and (b) [(NH4)Cs]CdAs4S8 (inset: direct or indirect band gap fit based on the DFT results). | |
In addition, inspired by recent reports that most of the lone-pair-based chalcogenides display intriguing photocatalytic properties,38–43 the photoelectrochemical experiment was performed through a standard three-electrode system using simulated solar light illumination to study the photoelectric properties of Cs2ZnAs4S8 and [(NH4)Cs]CdAs4S8. As illustrated in Fig. 3, the photocurrent–time curves exhibit a rapid and consistent photocurrent response in a multiple 20 s switching period. Clearly, Cs2ZnAs4S8 shows a remarkable transient photocurrent response, which is about 4 times that of [(NH4)Cs]CdAs4S8, that is, Cs2ZnAs4S8 possesses higher photogenerated electron–hole pair separation efficiency than [(NH4)Cs]CdAs4S8 under visible light irradiation. Meanwhile, these repeatable anodic photocurrent responses suggest that Cs2ZnAs4S8 and [(NH4)Cs]CdAs4S8 belong to n-type semiconductors. It is worth mentioning that these values of photocurrent densities (ca. 3.0 and 0.75 μA cm−2 for Cs2ZnAs4S8 and [(NH4)Cs]CdAs4S8, respectively) are much higher than those of most recently reported chalcogenides, such as Rb2Ba3Cu2Sb2S10 (ca. 6 nA cm−2),9 BaCuSbSe3 (ca. 30 nA cm−2),38 and BaCuSbS3 (ca. 55 nA cm−2).38
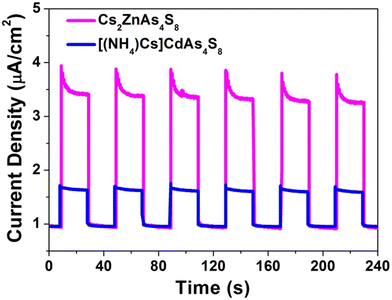 |
| Fig. 3 Photocurrent response curves of Cs2ZnAs4S8 and [(NH4)Cs]CdAs4S8. | |
To well understand the origin of difference of electronic structures and structure–activity relationships, theoretical calculations of Cs2ZnAs4S8 and [(NH4)Cs]CdAs4S8 have been systematically carried out based on DFT methods. As shown in Fig. 4a and b, the valence band maximum (VBM) and the conduction band minimum (CBM) located at the same high-symmetry points (i.e., Z|R) indicate that Cs2ZnAs4S8 is a direct-band-gap (Eg = 1.92 eV) semiconductor, while [(NH4)Cs]CdAs4S8 is an indirect-band-gap (Eg = 2.13 eV) semiconductor since the VBM and the CBM are located at different high-symmetry points (i.e., Z|R and V, respectively). These calculated values are slightly smaller than the experimental observations (2.24 eV for Cs2ZnAs4S8 and 2.36 eV for [(NH4)Cs]CdAs4S8, as given in Fig. 2), which is a well-known phenomenon for the local approximations to DFT.44 It is widely known that the photocatalytic activity mainly depends on the separation and diffusion rate of the photogenerated charge carriers, which can be judged by calculating the relative effective masses of electrons and holes based upon the electronic structures of the VBM and CBM in the title compounds.38 Through comparison, we can clearly see that the electronic bands of Cs2ZnAs4S8 are steeper than those of [(NH4)Cs]CdAs4S8. Namely, Cs2ZnAs4S8 is more conducive to improving the efficiency of photocatalysis, which is basically consistent with the experimental observation (Fig. 3). In addition, the projected density of states (PDOSs) with major contributions of title compounds is illustrated in Fig. 4c and d. Cs2ZnAs4S8 and [(NH4)Cs]CdAs4S8 exhibit similar results: the band from −5 eV to the Fermi level (EF), S-3p as well as As-4p and Zn-3d (or Cd-4d) states makes the main contribution, while the bottom of the CB is mainly composed of electronic hybridization of S-3p, As-4p and As-4s states. Consequently, the optical Eg values of Cs2ZnAs4S8 and [(NH4)Cs]CdAs4S8 mainly come from the charge transfer of Zn(or Cd)–S and As–S units, in which charge-balancing cations (e.g., Cs+, (NH4)+) show negligible contributions for the DOS. On the basis of their electron structures, we also calculated the birefringence (Δn) of Cs2ZnAs4S8 and [(NH4)Cs]CdAs4S8, respectively. As displayed in Fig. 5, their Δn values in both the important wavelengths can be calculated as 0.17@1064 nm and 0.18@2050 nm for Cs2ZnAs4S8 and 0.29@1064 nm and 0.31@2050 nm for [(NH4)Cs]CdAs4S8, which are comparable with some recently reported chalcogenides, indicating that they have potential as UV-vis or IR birefringent crystals. As known, the Δn value will mainly depend on the anisotropy of the anionic substructure, whereas the contribution of charge-balancing cations can be neglected.45–50 In other words, compared with the 3D anionic [CdAs4S8]2− framework in [(NH4)Cs]CdAs4S8, the anisotropy of the 2D anionic [ZnAs4S8]2− layer in Cs2ZnAs4S8 is more obvious, which is more beneficial for producing larger Δn values.
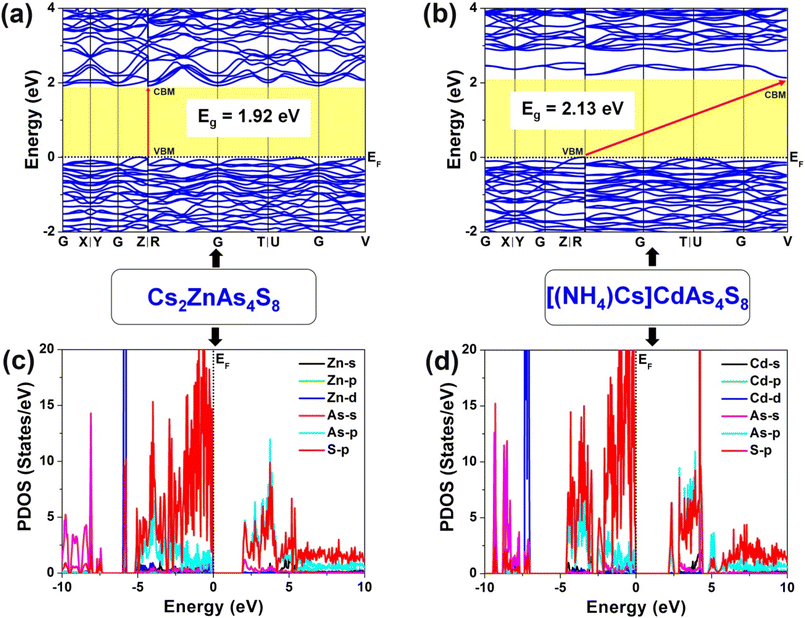 |
| Fig. 4 Theoretical calculated results of Cs2ZnAs4S8 and [(NH4)Cs]CdAs4S8: (a, b) electronic band structures; (c, d) PDOSs ((states with fewer contributions are omitted for a better view). The Fermi level EF is set at 0.0 eV. | |
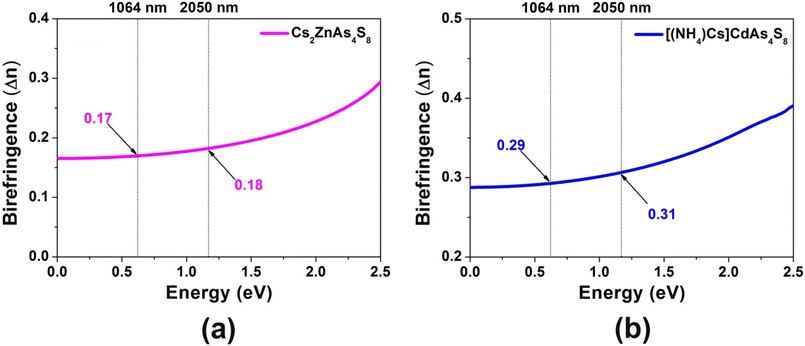 |
| Fig. 5 Curves of the calculated birefringence (Δn) as a function of energy (eV) for (a) Cs2ZnAs4S8 and (b) [(NH4)Cs]CdAs4S8. | |
Conclusions
In conclusion, two new members of the multinary X-TM-As-S family, Cs2ZnAs4S8 and [(NH4)Cs]CdAs4S8, have been prepared by a simple surfactant–thermal method. Although they have similar chemical stoichiometry 2–1–4–8, they undergo an intriguing dimensional transition from a 3D framework to a 2D layered structure. The optical absorption spectra and photoelectric results confirm that both the thioarsenates are wide-bandgap semiconductors and Cs2ZnAs4S8 exhibits a better photocurrent response than [(NH4)Cs]CdAs4S8 under the same test conditions. Meanwhile they also show large birefringence. In particular for [(NH4)Cs]CdAs4S8, the calculated birefringence values are 0.29@1064 nm and 0.31@2050 nm, respectively, suggesting its potential for application as a dual-waveband birefringent crystal. The analysis results of the structure–activity relationship show that the low dimensional structure of this family will be favorable for the generation of large optical anisotropy, that is, to obtain high birefringence. These results provide new insights into the exploration of novel functional chalcogenides and further research on the other physical properties of the title compounds is ongoing.
Author contributions
C. Zhang prepared the samples, and designed and carried out the experiments. S. H. Zhou carried out the theoretical calculations. Y. Xiao measured the optical properties. H. Lin and Y. Liu conceived the experiments, analyzed the results and wrote and edited the manuscript. All the authors have approved the final version of the manuscript.
Conflicts of interest
There are no conflicts to declare.
Acknowledgements
This work was supported by the National Natural Science Foundation of China (No. 52171277, 22175175 and 21771179), the Natural Science Foundation of Fujian Province (No. 2019J01133), the Fujian Science & Technology Innovation Laboratory for Optoelectronic Information of China (2021ZR118) and the Shanxi-Zheda Institute of Advanced Materials and Chemical Engineering (2022SZ-TD006).
References
- T. K. Bera, J. I. Jang, J. B. Ketterson and M. G. Kanatzidis, Strong Second Harmonic Generation from the Tantalum Thioarsenates A3Ta2AsS11 (A = K and Rb), J. Am. Chem. Soc., 2009, 131, 75–77 CrossRef CAS PubMed.
- C. D. Malliakas, D. Y. Chung, H. Claus and M. G. Kanatzidis, Superconductivity in the Narrow-Gap Semiconductor CsBi4Te6, J. Am. Chem. Soc., 2013, 135, 14540–14543 CrossRef CAS PubMed.
- L. L. Long, A. Y. Zhang, Y. X. Huang, X. Zhang and H. Q. Yu, A robust cocatalyst Pd4S uniformly anchored onto Bi2S3 nanorods for enhanced visible light photocatalysis, J. Mater. Chem. A, 2015, 3, 4301–4306 RSC.
- C. D. Malliakas, D. Y. Chung, H. Claus and M. G. Kanatzidis, Superconductivity in the Narrow Gap Semiconductor RbBi11/3Te6, J. Am. Chem. Soc., 2016, 138, 14694–14698 CrossRef CAS PubMed.
- G. J. Tan, L. D. Zhao and M. G. Kanatzidis, Rationally Designing High-Performance Bulk Thermoelectric Materials, Chem. Rev., 2016, 116, 12123–12149 CrossRef CAS PubMed.
- N. Markus, F. Felix, K. Marcus and O. Oliver, Single crystal structure elucidation and thermoelectric properties of a long-periodically ordered germanium arsenic telluride, J. Alloys Compd., 2017, 694, 1160–1164 CrossRef.
- H. Lin, Y. Y. Li, M. Y. Li, Z. J. Ma, L. M. Wu, X. T. Wu and Q. L. Zhu, Centric-to-acentric structure transformation induced by a stereochemically active lone pair: a new insight for design of IR nonlinear optical materials, J. Mater. Chem. C, 2019, 7, 4638–4643 RSC.
- P. Levinsky, C. Candolfi, A. Dauscher, J. Tobola, J. Hejtmánek and B. Lenoir, Thermoelectric properties of the tetrahedrite–tennantite solid solutions Cu12Sb4−xAsxS13 and Cu10Co2Sb4−yAsyS13 (0 ≤ x, y ≤ 4), Phys. Chem. Chem. Phys., 2019, 21, 4547–4555 RSC.
- C. Liu, Y. Xiao, H. Wang, W. X. Chai, X. F. Liu, D. M. Yan, H. Lin and Y. Liu, One-Dimensional Chains in Pentanary Chalcogenides A2Ba3Cu2Sb2S10 (A = K, Rb, Cs) Displaying a Photocurrent Response, Inorg. Chem., 2020, 59, 1577–1581 CrossRef CAS PubMed.
- H. Lin, W. B. Wei, H. Chen, X. T. Wu and Q. L. Zhu, Rational design of infrared nonlinear optical chalcogenides by chemical substitution, Coord. Chem. Rev., 2020, 406, 213150 CrossRef CAS.
- M. Yan, H.-G. Xue and S.-P. Guo, Recent Achievements in Lone-Pair Cation-Based Infrared Second-Order Nonlinear Optical Materials, Cryst. Growth Des., 2021, 21, 698–720 CrossRef CAS.
- M.-M. Chen, Z. Ma, B.-X. Li, W.-B. Wei, X.-T. Wu, H. Lin and Q.-L. Zhu, M2As2Q5 (M = Ba, Pb; Q = S, Se): a source of infrared nonlinear optical materials with excellent overall performance activated by multiple discrete arsenate anions, J. Mater. Chem. C, 2021, 9, 1156–1163 RSC.
- Y. Xiao, M. M. Chen, Y. Y. Shen, P. F. Liu, H. Lin and Y. Liu, A3Mn2Sb3S8 (A = K and Rb): a new type of multifunctional infrared nonlinear optical material based on unique three-dimensional open frameworks, Inorg. Chem. Front., 2021, 8, 2835–2843 RSC.
- M.-M. Chen, S.-H. Zhou, W.-B. Wei, B.-X. Li, M.-Y. Ran, X.-T. Wu, H. Lin and Q.-L. Zhu, RbBiP2S6: A Promising IR Nonlinear Optical Material with a Giant Second-Harmonic Generation Response Designed by Aliovalent Substitution, ACS Mater. Lett., 2022, 4, 1264–1269 CrossRef CAS.
- H. Chen, M.-Y. Ran, W.-B. Wei, X.-T. Wu, H. Lin and Q.-L. Zhu, A comprehensive review on metal chalcogenides with three-dimensional frameworks for infrared nonlinear optical applications, Coord. Chem. Rev., 2022, 470, 214706 CrossRef CAS.
- H. Chen, W. B. Wei, H. Lin and X. T. Wu, Transition-metal-based chalcogenides: A rich source of infrared nonlinear optical materials, Coord. Chem. Rev., 2021, 448, 214154 CrossRef CAS.
- A. Abudurusuli, K. Wu, Y. Rouzhahong, Z. Yang and S. Pan, Na6Zn3MIII2Q9 (MIII = Ga, In; Q = S, Se): four new supertetrahedron-layered chalcogenides with unprecedented vertex-sharing T3-clusters and desirable photoluminescence performances, Inorg. Chem. Front., 2018, 5, 1415–1422 RSC.
- M.-M. Chen, S.-H. Zhou, W.-B. Wei, X.-T. Wu, H. Lin and Q.-L. Zhu, Phase Matchability Transformation in the Infrared Nonlinear Optical Materials with Diamond-Like Frameworks, Adv. Opt. Mater., 2022, 10, 2102123 CrossRef CAS.
- C. Li, W. Yin, P. Gong, X. Li, M. Zhou, A. Mar, Z. Lin, J. Yao, Y. Wu and C. Chen, Trigonal Planar [HgSe3]4− Unit: A New Kind of Basic Functional Group in IR Nonlinear Optical Materials with Large Susceptibility and Physicochemical Stability, J. Am. Chem. Soc., 2016, 138, 6135–6138 CrossRef CAS PubMed.
- Y. D. Wu and W. Bensch, Synthesis, crystal structures, and optical properties of NaCdPnS3 (Pn = As, Sb), J. Alloys Compd., 2012, 511, 35–40 CrossRef CAS.
- R. G. lyer and M. G. Kanatzidis, [Mn2(AsS4)4]8− and [Cd2(AsS4)2(AsS5)2]8−: Discrete Clusters with High Negative Charge from Alkali Metal Polythioarsenate Fluxes, Inorg. Chem., 2004, 43, 3656–3662 CrossRef.
- X. Y. Tian, C. X. Du, G. T. ZhaoRi, M. G. SheLe, Y. S. Bao and M. H. Baiyin, The solvothermal synthesis and characterization of quaternary arsenic chalcogenides CsTMAsQ3 (TM = Hg, Cd; Q = S, Se) using Cs+ as a structure directing agent: from 1D anionic chains to 2D anionic layers, RSC Adv., 2020, 10, 34903–34909 RSC.
- W. W. Xiong, E. U. Athresh, Y. T. Ng, J. Ding, T. Wu and Q. C. Zhang, Growing Crystalline Chalcogenidoarsenates in Surfactants: From Zero-Dimensional Cluster to Three-Dimensional Framework, J. Am. Chem. Soc., 2013, 135, 1256–1259 CrossRef CAS.
- W. W. Xiong, P. Z. Li, T. H. Zhou, A. Y. Tok, R. Xu, Y. Zhao and Q. C. Zhang, Kinetically Controlling Phase Transformations of Crystalline Mercury Selenidostannates through Surfactant Media, Inorg. Chem., 2013, 52, 4148–4150 CrossRef CAS PubMed.
- C. Liu, Y. Y. Shen, P. P. Hou, M. J. Zhi, C. M. Zhou, W. X. Chai, J. W. Cheng and Y. Liu, Hydrazine-Hydrothermal Synthesis and Characterization of the Two New Quaternary Thioantimonates(III) BaAgSbS3 and BaAgSbS3·H2O, Inorg. Chem., 2015, 54, 8931–8936 CrossRef CAS.
- W. W. Xiong and Q. C. Zhang, Surfactants as Promising Media for the Preparation of Crystalline Inorganic Materials, Angew. Chem., Int. Ed., 2015, 54, 11616–11623 CrossRef CAS.
- Y. Y. Shen, C. Liu, P. P. Hou, M. J. Zhi, C. M. Zhou, W. X. Chai, Q. C. Zhang and Y. Liu, Facile surfactant-thermal syntheses and characterization of quaternary copper thioantimonates(III) ACu2SbS3 (A = K, Rb, Cs), J. Alloys Compd., 2016, 660, 171–177 CrossRef CAS.
- D. M. Yan, P. P. Hou, C. Liu, W. X. Chai, X. R. Zheng, L. D. Zhang, M. J. Zhi, C. M. Zhou and Y. Liu, Effect of alkali cations on two-dimensional networks of two new quaternary thioarsenates (III) prepared by a facile surfactant-thermal method, J. Solid State Chem., 2016, 241, 47–53 CrossRef CAS.
- D. M. Yan, C. Liu, W. X. Chai, X. R. Zheng, L. D. Zhang, M. J. Zhi, C. M. Zhou, Q. C. Zhang and Y. Liu, Facile Hydrazine-Hydrothermal Syntheses and Characterizations of Two Quaternary Thioarsenates(III): Two-Dimensional SrAg4As2S6·2H2O and One-Dimensional BaAgAsS3, Chem. – Asian J., 2016, 11, 1842–1848 CrossRef CAS.
- D. M. Yan, Y. Xiao, C. Liu, P. P. Hou, W. X. Chai, H. Hosono, H. Lin and Y. Liu, Two new members in the quaternary Cs-Ag-As-S family with different arrangements of Ag–S and As–S asymmetric building units: syntheses, structures, and theoretical studies, Dalton Trans., 2020, 49, 9743–9750 RSC.
- R. Ye, B. W. Liu, X. M. Jiang, J. Lu, H. Y. Zeng and G. C. Guo, AMnAs3S6 (A = Cs, Rb): Phase-Matchable Infrared Nonlinear Optical Functional Motif [As3S6]3− Obtained via Surfactant–Thermal Method, ACS Appl. Mater. Interfaces, 2020, 12, 53950–53956 CrossRef CAS.
- H. Lin, L. J. Zhou and L. Chen, Sulfides with Strong Nonlinear Optical Activity and Thermochromism: ACd4Ga5S12 (A = K, Rb, Cs), Chem. Mater., 2012, 24, 3406–3414 CrossRef CAS.
- H. Lin, H. Chen, Y. J. Zheng, J. S. Yu, X. T. Wu and L. M. Wu, Coexistence of Strong Second Harmonic Generation Response and Wide Band Gap in AZn4Ga5S12 (A = K, Rb, Cs) with 3D Diamond-like Frameworks, Chem. – Eur. J., 2017, 23, 10407–10412 CrossRef CAS PubMed.
- Y. J. Zheng, Y. F. Shi, C. B. Tian, H. Lin, L. M. Wu, X. T. Wu and Q. L. Zhu, An Unprecedented Pentanary Chalcohalide with the Mn Atoms in Two Chemical Environments: Unique Bonding Characteristics and Magnetic Properties, Chem. Commun., 2019, 55, 79–82 RSC.
- J. Tauc, R. Grigorovici and A. Vancu, Optical Properties and Electronic Structure of Amorphous Germanium, Phys. Status Solidi, 1966, 15, 627 CrossRef CAS.
- Y. Takeuchi and N. Haga, On the crystal structures of seligmannite, PbCuAsS3, and related minerals, Z. Kristallogr. - Cryst. Mater., 1969, 130, 254–260 CrossRef CAS.
- H. G. Yao, M. Ji, S. H. Ji and Y. L. An, Synthesis, structure and characterization of two new copper(I)-thioarsenates (III) constructed by the [AsS3]3− and CuSx units, J. Solid State Chem., 2013, 198, 289–294 CrossRef CAS.
- C. Liu, P. P. Hou, W. X. Chai, J. W. Tian, X. R. Zheng, Y. Y. Shen, M. J. Zhi, C. M. Zhou and Y. Liu, Hydrazine-hydrothermal syntheses, characterizations and photoelectrochemical properties of two quaternary chalcogenidoantimonates (III) BaCuSbQ3 (Q = S, Se), J. Alloys Compd., 2016, 679, 420–425 CrossRef CAS.
- C. Li, Z. Lin, L. Kang, Z. Lin, H. Huang, J. Yao and Y. Wu, Sn2SiS4, synthesis, structure, optical and electronic properties, Opt. Mater., 2015, 47, 379–385 CrossRef CAS.
- L. N. Nie and Q. C. Zhang, Recent progress in crystalline metal chalcogenides as efficient photocatalysts for organic pollutant degradation, Inorg. Chem. Front., 2017, 4, 1953–1962 RSC.
- L. N. Nie, G. F. Liu, J. Xie, T. T. Lim, G. S. Armatas, R. Xu and Q. C. Zhang, Syntheses, crystal structures, and photocatalytic properties of two ammonium-directed Ag-Sb-S complexes, Inorg. Chem. Front., 2017, 4, 954–959 RSC.
- M.-Y. Ran, S.-H. Zhou, W.-B. Wei, B.-J. Song, Y.-F. Shi, X.-T. Wu, H. Lin and Q.-L. Zhu, Quaternary Chalcohalides CdSnSX2 (X = Cl or Br) with Neutral Layers: Syntheses, Structures, and Photocatalytic Properties, Inorg. Chem., 2021, 60, 3431–3438 CrossRef CAS.
- M.-Y. Li, X.-Y. Xie, X.-T. Wu, X.-F. Li and H. Lin, Quaternary Selenophosphate Cs2ZnP2Se6 Featuring Unique One-dimensional Chains and Exhibiting Remarkable Photo-electrochemical Response, Chin. J. Struct. Chem., 2021, 40, 246–255 CAS.
- K. Burke, Perspective on density functional theory, J. Chem. Phys., 2012, 136, 150901 CrossRef PubMed.
- M. Y. Ran, Z. J. Ma, H. Chen, B. X. Li, X. T. Wu, H. Lin and Q. L. Zhu, Partial Isovalent Anion Substitution to Access Remarkable Second-Harmonic Generation Response: A Generic and Effective Strategy for Design of Infrared Nonlinear Optical Materials, Chem. Mater., 2020, 32, 5890–5896 CrossRef CAS.
- M.-Y. Ran, Z. Ma, X.-T. Wu, H. Lin and Q.-L. Zhu, Ba2Ge2Te5: a ternary NLO-active telluride with unusual one-dimensional helical chains and giant second-harmonic-generation tensors, Inorg. Chem. Front., 2021, 8, 4838–4845 RSC.
- C. Liu, S.-H. Zhou, C. Zhang, Y.-Y. Shen, X.-Y. Liu, H. Lin and Y. Liu, CsCu3SbS4: rational design of a two-dimensional layered material with giant birefringence derived from Cu3SbS4, Inorg. Chem. Front., 2022, 9, 478–484 RSC.
- M.-Y. Ran, S.-H. Zhou, B.-X. Li, W.-B. Wei, X.-T. Wu, H. Lin and Q.-L. Zhu, Enhanced Second-Harmonic-Generation Efficiency and Birefringence in Melillite Oxychalcogenides Sr2MGe2OS6 (M = Mn, Zn, and Cd), Chem. Mater., 2022, 34, 3853–3861 CrossRef CAS.
- H.-D. Yang, M.-Y. Ran, S.-H. Zhou, X.-T. Wu, H. Lin and Q.-L. Zhu, Rational Design via Dual-Site Aliovalent Substitution Leads to an Outstanding IR Nonlinear Optical Material with Well-Balanced Comprehensive Properties, Chem. Sci., 2022, 13, 10725–10733 RSC.
- Y.-F. Shi, Z. Ma, B.-X. Li, X.-T. Wu, H. Lin and Q.-L. Zhu, Phase matching achieved by isomorphous substitution in IR nonlinear optical material Ba2SnSSi2O7 with an undiscovered [SnO4S] functional motif, Mater. Chem. Front., 2022 10.1039/D2QM00621A.
Footnote |
† Electronic supplementary information (ESI) available: Additional experimental and theory results, together with additional tables and figures. CCDC 2167064 and 2167065. For ESI and crystallographic data in CIF or other electronic format see DOI: https://doi.org/10.1039/d2qi01694j |
|
This journal is © the Partner Organisations 2022 |