Combating pathogens with Cs2.5H0.5PW12O40 nanoparticles: a new proton-regulated antimicrobial agent†
Received
20th October 2017
, Accepted 1st December 2017
First published on 1st December 2017
Abstract
The transfer of pathogens from contaminated surfaces to patients is one of the main causes of health care-associated infections (HCAIs). Cases of HCAIs due to multidrug-resistant organisms have been growing worldwide, whereas inorganic nano-antimicrobials are valuable today for the prevention and control of HCAIs. Here, we present a cesium salt of phosphotungstic heteropolyacid (Cs2.5H0.5PW12O40) as a promising nanomaterial for use in antimicrobial product technologies. This water-insoluble Keggin salt exhibits a broad biocide spectrum against Gram-positive and Gram-negative bacteria, yeasts, and filamentous fungi even under dark conditions. The Cs2.5H0.5PW12O40 nanoparticles (NPs) act as a proton-regulated antimicrobial whose activity is mediated on the release of hydronium ions (H3O+), yielding an in situ acidic pH several units below those tolerable by most of the fungal and bacterial nosocomial pathogens.
Introduction
Nosocomial or health care-associated infections (HCAIs) are infections that patients acquire while receiving treatment at a health care facility that were not present at the time of admission.1 HCAIs are associated with increased health care costs – an additional cost between US$ 7453 and 15
155 per infected patient – and an average of 8 days of extra-length of stay per patient in hospitals.2 HCAIs not only delay patient recovery but also significantly contribute to patient mortality.1 Nowadays, the risks of HCAIs have become greater due to the fact that the available drugs to combat pathogens are losing their efficacy caused by the emergence of antimicrobial resistance.3 The incidence of HCAIs caused by multidrug-resistant organisms is increasing worldwide and finding a feasible drug against non-treatable HCAIs has become a challenge.4 It is estimated that the cumulative premature deaths caused by resistant microorganisms will reach 300 million by 2050 at a financial cost of up to $100 trillion to the global economy.5 The World Health Organization (WHO) and several researchers foresee the emergence of resistant microorganisms as one of the biggest public health threats of the 21st century,6,7 a century entitled as the Antibiotic-Resistance Era.8
It is well known that there are pathogens able to survive for months on surfaces at health care facilities.9 Due to this survival capability, a large number of HCAIs are eventually caused by the transference of pathogens from contaminated surfaces to patients.10 To address this issue, there are a huge number of studies looking for antimicrobial surfaces and devices containing nanosized inorganic antimicrobials.11,12 However, the approaches based on nano-antimicrobials to prevent HCAIs present some drawbacks: (i) the action of some inorganic antimicrobials is mediated by the release of heavy metal ions or nanoparticles (NPs), which are known to be potentially hazardous to humans and non-target cells,13 (ii) nanomaterials with antimicrobial activity based on the generation of reactive oxygen species (ROS) exhibit reduced efficacy under dark conditions,14 and (iii) some nanomaterials exhibit antimicrobial activity against a limited spectrum of pathogens.15 Indeed, the last concern is one important limitation for antimicrobial surfaces due to the high diversity of pathogens that occur at health care facilities.
Some of the above issues about the current inorganic antimicrobials have been addressed with an innovative concept based on acidic surfaces idealized by Guggenbichler, Zollfrank, and co-workers.16–18 The fundamental basis of this concept is the fact that several clinically relevant human pathogens are not able to grow in acidic environments. From a simplistic point of view, acidic surfaces work by lowering the interfacial pH between the functionalized surface and the microorganism, thus creating an inhospitable acidic environment for pathogens. This concept mimics the natural acidic nature of the human skin. A healthy human skin is an acidic environment,19 with pH ranging from 4 to 6,20 which is believed to be an evolutionary mechanism of defense against invading microorganisms.21 It also introduces an extremely relevant characteristic of acidic surfaces, that is; their intrinsic broad-spectrum antimicrobial activity. There are several clinically relevant human pathogens that are not able to grow in environments with pH less than 3.5.18 To build up acidic surfaces, an inorganic compound which has a large number of acidic sites able to release hydronium ions (H3O+) is necessary. Until now, molybdenum oxide-based compounds have been the unique reported materials suitable for this technology.22,23
This work presents a cesium salt of phosphotungstic heteropolyacid (Cs2.5H0.5PW12O40) as a new nano-antimicrobial with its action regulated by H3O+ release for the fabrication of antimicrobial acidic surfaces and devices. The Cs2.5H0.5PW12O40 NPs are a water-insoluble polyoxometalate (POM)24 with a Keggin structure that contains maximized density of surface acid sites,25 high surface area,26 and nanosized particles.27 Besides, its chemical structural features allow expressing high rates of H3O+ generation and proton mobility,28 being, as will be discussed here, a potential nanomaterial against HCAIs and overcoming the multidrug-resistant mechanisms.
Experimental
Synthesis of Cs2.5H0.5PW12O40 NPs
Cs2.5H0.5PW12O40 NPs were synthesized by precipitation according to the procedure of Ito et al.,29 with some modifications. 12-Phosphotungstic acid hydrate (H3PW12O40·xH2O, reagent grade – Sigma) and cesium chloride (CsCl, 99.9% – Sigma) were used as precursors without further purification. First, H3PW12O40·xH2O was dissolved in 55 mL of water to give an aqueous solution of 0.025 mol L−1, and, the resultant solution was cooled to 2 °C in an ice bath. In another flask, CsCl was dissolved in 63.1 mL of water to give an aqueous solution of 0.055 mol L−1, which was added dropwise at a rate of 0.7 mL min−1 to the first solution under stirring. The whole synthesis procedure lasted for 120 min, consisting of 90 min for complete dropwise addition of the cesium chloride solution and, further 30 min of final stirring at 2 °C. The white precipitates were separated by centrifugation, dried at 60 °C and then ground in an agate mortar.
Determination of the crystal structure
X-ray diffraction (XRD) patterns were obtained using a Siemens D5005 diffractometer in the Bragg–Brentano geometry with Cu Kα radiation (λ = 0.15418 nm) operating at a voltage of 40 kV and a current of 30 mA. X-ray diffractograms were collected at 2θ intervals between 5 and 60° with a step size of 0.03° and a scan time of 2 s per step. Raman spectra were recorded using a CRAIC 20/30 PV micro-spectrometer from 190 to 1900 cm−1 at 25 °C, under excitation at 830 nm and 49.8 mW.
Chemical composition
Structural water molecules (·nH2O) in Cs2.5H0.5PW12O40 were determined by thermogravimetric analysis (TG) using a TA Instruments SDTQ 600 thermal analyzer. Mass loss associated with the water molecules was measured after heating 15 mg of the as-synthesized powder from 25 to 300 °C at a heating rate of 10 °C min−1 and a nitrogen gas flow rate of 100 mL min−1. The real concentration of the Cs atoms in the Cs2.5H0.5PW12O40 Keggin structure was determined by standardless X-ray fluorescence using a Shimadzu EDX-720 spectrometer.
Concentration of surface acid sites
The density of surface acid sites of the Cs2.5H0.5PW12O40 NPs (μmol m−2) was estimated dividing the surface acidity (mmol g−1) by the surface area (m2 g−1). The surface acidity was determined by temperature-programmed desorption (TPD) of NH3 using a Micromeritics AutoChem II 2920 chemisorption analyzer. Initially, the samples were treated at 60 °C for 4 h under a stream of pure He. Subsequently, the temperature was cooled down to 50 °C and the samples were exposed to NH3 (10 vol% NH3 in He) for 30 min and immediately flushed with He (30 mL min−1) at the same temperature for 1 h to remove physically adsorbed NH3. Finally, the samples were heated up to 450 °C at a heating rate of 10 °C min−1 under a He stream (30 mL min−1). The specific surface area was determined by N2 physisorption at −196 °C via the Brunauer–Emmett–Teller method using a Micromeritics Model VII Gemini surface area analyzer.
Tested strains
The antimicrobial activity of Cs2.5H0.5PW12O40 NPs was evaluated against ten clinically relevant pathogens: Gram-negative bacteria Escherichia coli ATCC 25922 and Pseudomonas aeruginosa ATCC 14502, Gram-positive bacteria Staphylococcus aureus ATCC 25923 and multidrug-resistant Enterococcus faecium VRE16,30 filamentous fungus Aspergillus fumigatus akuBKU80,31 and yeasts Cryptococcus neoformans ATCC 90112, Cryptococcus gattii ATCC 32269, Candida albicans ATCC 64548, Candida glabrata ATCC 90030, and Candida parapsilosis ATCC 22019. All the antimicrobial tests with the Cs2.5H0.5PW12O40 NPs were carried out under dark conditions.
Electron microscopy
Scanning electron microscopy (SEM) images of the Cs2.5H0.5PW12O40 NPs were acquired using an FEI Magellan 400 L microscope at an accelerating voltage of 25 kV. Transmission electron microscopy (TEM) images of the Cs2.5H0.5PW12O40 NPs were obtained using a FEI Tecnai G2 F20 microscope operating at 200 kV. Experimental electron diffraction patterns were simulated using the JEMS software version 3.6907U2011.32 For the simulations, we used the crystallographic information file (CIF) of the parent Keggin salt, K3PMo12O40 (ICSD No. 150445), with structural parameters adjusted for the Cs2.5H0.5PW12O40 NPs after XRD analysis.
Electron microscopy images of C. parapsilosis were acquired with and without exposition to Cs2.5H0.5PW12O40 NPs. 50 mL of Sabouraud broth was inoculated with 200 μL of a preculture of C. parapsilosis (4 × 109 CFU mL−1) for previous growth at 37 °C in a reciprocal shaker (180 rpm) for 9 h. Subsequently, a flask containing the C. parapsilosis suspension was treated with ½ MIC of the Cs2.5H0.5PW12O40 NPs. Another flask without Cs2.5H0.5PW12O40 NPs was used as the control. Further, they were kept overnight at 37 °C in a reciprocal shaker (180 rpm). A pellet of C. parapsilosis cell suspension was collected by centrifugation at 3200g for 5 min and fixed at 4 °C for 24 h in 0.1 mol L−1 sodium phosphate buffer, pH 7.4, containing 2.5% glutaraldehyde and 2% paraformaldehyde. The fixed cells were stained with 1% OsO4. The final step of dehydration of the samples was performed using a series of increasing neat ethanol contents (25, 50, 75, 95, and 3 × 100%).33 Finally, the samples were dried under vacuum at 25 °C and observed using a FEI Magellan 400 L microscope at an acceleration voltage of 2 kV. Sample preparation for TEM images was performed as reported elsewhere,34 however using Spurr's resin. TEM images of C. parapsilosis cells were acquired using a FEI Tecnai G2 F20 microscope operating at 200 kV.
Minimum inhibitory/biocidal concentration
Minimum inhibitory concentration (MIC) of the Cs2.5H0.5PW12O40 NPs was determined by the broth microdilution method recommended by CLSI M38-A2,35 M27-A2,36 and M7-A6,37 respectively for fungi, yeasts, and bacteria. The experiments were performed in 96-well plates using Sabouraud broth for the filamentous fungus and yeasts, and Mueller Hinton broth for bacteria. Each well was filled with 175 μL of serial broth dilutions containing the Cs2.5H0.5PW12O40 NPs and inoculated with 5 μL of a fresh culture of each organism (105 CFU or conidia mL−1). 20 μL of alamarBlue (Thermo Scientific) was added into the above wells as a colorimetric indicator of metabolic activity. The plates were incubated for 24 h at 35 °C for bacteria and at 37 °C for the filamentous fungus and yeasts. The MIC corresponds to the lowest concentration of the compound that prevents the microbial growth. After the MIC evaluation, 100 μL of culture from the wells, in which no visible microbial growth was detected, were inoculated on agar plates under the same conditions as those of the MIC test. From these readings, the minimum biocidal concentration (MBC) was assumed to be the lowest concentration of the Cs2.5H0.5PW12O40 NPs that kills 100% of the initial population of microorganisms.
Microbial growth curves for yeasts and bacteria
The inhibitory effect of the Cs+ and [PW12O40]3− ions on the microbial growth was assessed by measuring optical density at 600 nm (OD600nm) using a Biochrom Libra S50 spectrophotometer. CsCl and H3PW12O40·xH2O were used as the sources of Cs+ and [PW12O40]3− ions, respectively. Initially, the flasks containing 50 mL of Sabouraud or Mueller Hinton broth were inoculated with 200 μL of a fresh suspension of each microorganism at 4 × 109 CFU mL−1. The growth inhibition by the Cs2.5H0.5PW12O40 NPs was done at the MIC value of each strain. The concentrations of Cs+ and [PW12O40]3− were evaluated based on the 6.4% water solubility reported for Cs2.5H0.5PW12O40.27 Then, the respective concentration of Cs+ and [PW12O40]3− was calculated considering the stoichiometric amount dissociated from the MIC of each strain. Experiments were performed at 35 °C for bacteria and 37 °C for yeasts in an orbital shaker (200 rpm). For OD600 readings, aliquots of the suspensions were taken hourly up to 14 h. The turbidity generated by the broth, Cs+, [PW12O40]3−, or Cs2.5H0.5PW12O40 was considered by taking blank readings. The tests were conducted in triplicate, and the average values are reported.
Phenotypic characterization of A. fumigatus
A. fumigatus conidia were aseptically collected from three day cultures in solid complete medium [YG; glucose 2% (w/w), 0.5% yeast extract (w/w), 1X trace elements, pH 6.5]. The composition of trace elements was described previously.38 Conidia were dispersed in autoclaved Milli-Q water and the concentration of the suspension was adjusted to 105 conidia mL−1 employing a Neubauer chamber. Photographs of radial growth were recorded after the inoculation of 5 μL of the conidia suspension in the center of Petri dishes containing solid YG for 72 h at 37 °C. Following the image acquisition, the same plates were used for conidia count. Conidia were counted after dispersion of agar fragments (0.8 cm2), taken from the radial zone, in an aqueous solution with 0.01% Tween 20. The reported values are the average of triplicate tests. The morphology of A. fumigatus was observed by optical microscopy using an Olympus Bx50 microscope. Prior to imaging, the fungi were harvested from the radial growth plates and stained with lactophenol blue solution (Sigma).
Concentration of H3O+
The concentration of H3O+ ions was assessed after dispersion of the Cs2.5H0.5PW12O40 NPs in distilled water. In the suspension, the powder was ultrasonically dispersed and the pH was measured using a PG 1800 (Gehaka) digital pH meter. The concentration of the hydronium ions was estimated using the following relation: [H3O+] = 10−pH.
Results
Nanostructured Cs2.5H0.5PW12O40
According to the XRD pattern shown in Fig. 1a, precipitation is a synthetic approach suitable for producing single-phase Cs2.5H0.5PW12O40 nanopowders. The cesium salt precipitates as cubic crystals with Pn
m symmetry and a measured lattice parameter of 1.3641 nm. It is known that the formation of three-dimensional Cs2.5H0.5PW12O40 crystals occurs when the primary§ Keggin heteropolyanion [PW12O40]3− has part of its structural proton sites substituted by the counter-cation Cs+.25,39 The Raman spectrum (Fig. 1b) confirms the formation of Cs2.5H0.5PW12O40 with [PW12O40]3− as its fundamental building blocks. The presence of intense bands at 1008 and 996 cm−1 matches with those of the Raman vibrational frequencies of the W–Od bond predicted at 1011 and 996 cm−1 for [PW12O40]3−.40
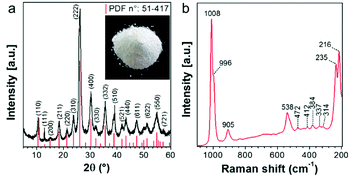 |
| Fig. 1 (a) XRD pattern and (b) Raman spectrum of Cs2.5H0.5PW12O40 NPs. The photograph of the synthesized nanopowder is the inset in (a). The vertical red lines show positions of Bragg peaks (powder diffraction file – PDF) for the crystal structure of the cesium salt of phosphotungstic heteropolyacid. | |
The powder characteristics of the Cs2.5H0.5PW12O40 NPs are summarized in Table 1. The cesium content of 2.4 mol indicates partial replacement of protons close to the expected quantity of 2.5 mol. This appropriate replacement of the protons by the large-sized Cs+ ensures the formation of a water-insoluble Keggin salt.40 Its insolubility in water is a key feature for the prospect of this compound into the group of inorganic antimicrobials of industrial interest. Moreover, the secondary structure occurs associated with eight structural water molecules, yielding the real chemical formula Cs2.5H0.5PW12O40·8H2O, although, for clarity, water molecules will be omitted in this paper. The morphological investigation by electron microscopy (Fig. 2) shows that the Cs2.5H0.5PW12O40 nanopowder contains primary nanoparticles with an average size of 10 nm. In addition, there is a self-arrangement of the nanosized primary particles to produce tertiary structures with a spherical morphology of 100 nm (Fig. 2a and b). The tendency of CsxH1−xPW12O40 compounds to form tertiary Keggin structures with a spherical morphology has also been reported by other research groups.25,41 Furthermore, the presence of microporosity among the primary particles in this tertiary spherical morphology is responsible for the relatively high surface area of 116 m2 g−1 measured for the Cs2.5H0.5PW12O40 nanopowder.42
Table 1 Morphological and chemical characteristics of the Cs2.5H0.5PW12O40 NPs
Cesium salt of phosphotungstic acid |
Tertiary morphology |
Spherical |
Size of tertiary structure |
100 nm |
Primary particle size |
10 nm |
Surface area |
116 m2 g−1 |
Atomic content of Cs+ |
2.4 |
·nH2O (mol) |
8 |
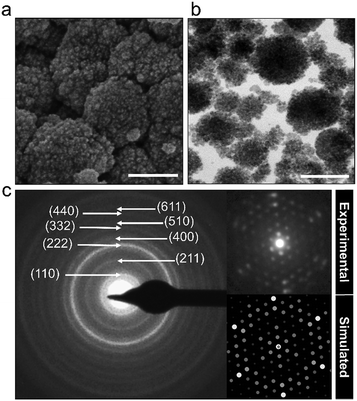 |
| Fig. 2 (a) SEM and (b) TEM images of Cs2.5H0.5PW12O40 NPs. Scale bars correspond to 100 nm. (c) The electron diffraction patterns from a polycrystalline region and a single particle oriented along the [111] zone axis reveals the cubic symmetry of the primary particles. | |
A broad spectrum of action
The Cs2.5H0.5PW12O40 NPs behave as a broad-spectrum antimicrobial agent according to the MIC values shown in Table 2. The table demonstrates the concentration where the growth is completely inhibited in MIC analysis. Interestingly, for most of the microorganisms tested, at this concentration the Cs2.5H0.5PW12O40 NPs also behave as a biocide, therefore leading to complete loss of viability of all the tested strains, except for the bacteria E. coli and P. aeruginosa. This result is evidence for the potential use of the Cs2.5H0.5PW12O40 NPs as a biocide against pathogens. Noteworthily, the Gram-negative bacteria also lost viability in the presence of the compound; however, for the two strains, the MBC was found to be higher than the MIC (3.00 mg mL−1). The low susceptibility of Gram-negative bacteria to Keggin salts was also observed previously.43 Comparing the antibacterial activity of Na7PMo11CuO40 against S. aureus and E. coli, the authors found the MBC to be two times higher for E. coli. Moreover, the resistance of Gram-negative bacteria is also common for other nano-antimicrobials, such as ZnO NPs.44 Importantly, the MBC of the Cs2.5H0.5PW12O40 NPs described herein is significantly lower than those reported for similar Keggin salts. For instance, Na3PW12O40 exhibits a MBC of 11.03 mg mL−1 against S. aureus, P. aeruginosa, and E. coli45 while neutral silver-based POMs exhibited a MBC higher than 20.00 mg mL−1 against S. aureus and E. coli.46 POMs can behave as photocatalysts under UV irradiation and produce reactive oxygen species (ROS),47 therefore, it is worth mentioning that our MIC/MBC experiments were performed under dark conditions, indicating that the Cs2.5H0.5PW12O40 NPs are an active nano-antimicrobial even in the dark.
Table 2 MIC and MBC values for the Cs2.5H0.5PW12O40 NPs against all tested strains
Microorganism |
MIC (mg mL−1) |
MBC (mg mL−1) |
Escherichia coli
|
1.75 |
3.00 |
Pseudomonas aeruginosa
|
2.00 |
3.00 |
Staphylococcus aureus
|
1.50 |
1.50 |
Enterococcus faecium
|
1.25 |
1.25 |
Aspergillus fumigatus
|
3.00 |
3.00 |
Cryptococcus neoformans
|
3.00 |
3.00 |
Cryptococcus gattii
|
3.00 |
3.00 |
Candida albicans
|
3.00 |
3.00 |
Candida glabrata
|
3.00 |
3.00 |
Candida parapsilosis
|
2.50 |
2.50 |
Another interesting finding in Table 2 is the small MIC and MBC value against the multidrug-resistant E. faecium. In this case, the strain with documented resistance to most of the current antibiotics depicted the highest sensitivity to the Cs2.5H0.5PW12O40 NPs. Other authors reported that the resistance to drugs does not attenuate the susceptibility of bacterial strains to Keggin-structural POMs.48,49 For instance, methicillin-resistant S. aureus (MRSA), multidrug resistant P. aeruginosa, and extended spectrum β-lactamase producing Klebsiella pneumonia exhibit a lower MIC value than the reference bacterial strains when exposed to Na3PW12O40.45
To gain further insight into the extension of the antimicrobial activity of the Cs2.5H0.5PW12O40 NPs, in the analysis of Table 2, we also included yeasts and a filamentous fungus with a notorious ability to infect immunocompromised patients with HCAIs.50 All the yeasts and filamentous fungus exhibited sensitivity to the Cs2.5H0.5PW12O40 NPs, with the MIC values equal to the MBC. For comparison, the MBC value of 3.00 mg mL−1 for the Cs2.5H0.5PW12O40 NPs in the fungal species is strikingly smaller than the values reported for the silver-based POMs, i.e. > 20.00 mg mL−1.46
A. fumigatus produces massive amounts of conidia of small size (2–3 μm) which can be highly dispersive in air. Conidiation is therefore one of the main virulence determinants of this pathogen. It is thought that, under normal conditions, every individual inhales about 100 conidia of this fungus per day due to its ubiquitous distribution worldwide coupled with its efficient conidiation process.51 Therefore, we further investigated whether the Cs2.5H0.5PW12O40 NPs could impair the ability of A. fumigatus to produce conidia. The phenotypic investigation revealed that the Cs2.5H0.5PW12O40 NPs induce the reduction of conidiation, which is noticed by the absence of the greenish color of the A. fumigatus colony and by the quantification of the number of conidia (Fig. 3a). More precisely, we found a reduction in conidiation on the order of 99% for A. fumigatus exposed to the Cs2.5H0.5PW12O40 NPs, which can be seen by comparing the optical microscopy images shown in Fig. 3b. Considering that invasive pulmonary aspergillosis is acquired after accidental inhalation of conidia by immunocompromised patients,52 this reduction of fungus conidiation by the Cs2.5H0.5PW12O40 NPs further emphasizes the relevant role of this compound in hospital environments to mitigate the dispersion of these infectious propagules. Altogether, these results reinforce the potential use of Cs2.5H0.5PW12O40 NPs as a biocide nano-antimicrobial against fungal and bacterial pathogens of high incidence in nosocomial infections worldwide.
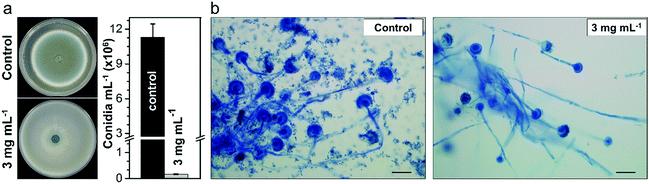 |
| Fig. 3 Morphological alterations in A. fumigatus induced by the Cs2.5H0.5PW12O40 NPs. (a) Photographs of radial growth with quantification of the level of conidiation. (b) Optical images of A. fumigatus without and with exposition to Cs2.5H0.5PW12O40 NPs. Scale bars correspond to 200 μm. | |
The mechanism of action of Cs2.5H0.5PW12O40 NPs is not regulated by the [PW12O40]3− and Cs+ ions
Metal ion release is one of the dominant antimicrobial mechanisms of metal oxide nanoparticles,53 therefore, we performed microbial growth assays to clarify the correlation between the [PW12O40]3− and Cs+ ions and the antimicrobial phenomenon of the Cs2.5H0.5PW12O40 NPs. The growth curves of some selected pathogens are shown in Fig. 4. For all the investigated strains, the presence of [PW12O40]3− in the liquid medium did not affect the growth curves, exhibiting the same growth profile as the controls. In the broth containing the Cs2.5H0.5PW12O40 NPs, S. aureus did not grow and the growth of P. aeruginosa corresponded to 67%, that of C. neoformans corresponded to 46%, and that of C. albicans corresponded to 51% surviving cells in comparison to the growth in the presence of [PW12O40]3−. Complete growth inhibition of P. aeruginosa, C. neoformans, and C. albicans in the presence of Cs2.5H0.5PW12O40 NPs occurs only at concentrations three times that of the MIC for each strain. Note that these concentrations differ from the MIC results due to the different inoculation and growth conditions used for the microbial growth curves. Therefore, there was a clear inhibition of the growth of microorganisms in the presence of the Cs2.5H0.5PW12O40 NPs. This suggests that no connection exists between the reduced growth rates of the strains in the presence of the Cs2.5H0.5PW12O40 NPs and the presence of the Keggin heteropolyanion in solution. This result is in agreement with the findings of Lu et al.54 and Wang et al.55 Both the authors observed that the antibacterial activity of silver-based POMs is not mediated by the release of [PW12O40]3−. Furthermore, according to Hu et al.,56 even a concentration of [PW12O40]3− as high as 1.06 mg mL−1 does not inhibit the growth of S. aureus and E. coli. Another front of studies, however, have suggested that the release of counter-cations is a regulatory pathway for the antimicrobial phenomenon in Keggin salts. These studies have associated the origin of the antimicrobial behaviour with the release of NH4+ in (NH4)3PMo12O40,57 Ag+ in silver-based POMs,46,55 and, recently, the tetraalkylammonium cations in exchanged silicotungstates.58 However, as for the [PW12O40]3−, our experimental growth curves did not indicate any appreciable inhibition effect by the counter-cation Cs+. Hence, the antimicrobial activity of the Cs2.5H0.5PW12O40 NPs does not seem to be a consequence of the ion release, which is expected due to the low tendency of this Keggin salt to release heteropoly species into water.27
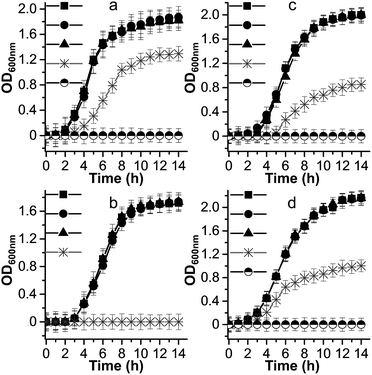 |
| Fig. 4 Microbial growth curves for: (a) P. aeruginosa, (b) S. aureus, (c) C. neoformans, and (d) C. albicans. Symbols represent the nutrient broth containing: (●) [PW12O40]3−, (▲) Cs+, ( ) Cs2.5H0.5PW12O40 NPs, and (■) control without compounds. The symbol ( ) indicates experiments carried out at concentrations of Cs2.5H0.5PW12O40 NPs equal to three times the MIC value for each strain. For simplicity, we consider the [PW12O40]3− as the stable heteropolyanion in the nutrient broth solution, although 31P and 183W NMR studies indicate that the heteropolyanion can exist as the lacunary [PW11O39]7− in the pH of the broth.79 | |
Internalization of nanoparticles is not necessary for the action of the Cs2.5H0.5PW12O40 NPs
Another antimicrobial mechanism often associated with nanosized materials is the physical interaction between the nanoparticles and the cells.53 In particular in fungi and bacteria, this interaction is mediated by the cell wall which is a dynamic rigid, but permeable tridimensional structure composed mainly of carbohydrates that protects the cell against osmotic lysis and also provides cell shape.59 In order to determine whether the Cs2.5H0.5PW12O40 NPs act by cell wall damage or internalization of nanoparticles, we performed a careful electron microscopy investigation of C. parapsilosis cells. This yeast was chosen because it was the most sensitive fungal organism to our compound (Table 2). In addition, C. parapsilosis is the main non-albicans Candida species isolated from patients in Latin America.60 In a scenario of cell death induced by nanoparticles, visibly damaged cells with loss of the cell wall integrity are expected.61 We found that this scenario did not occur in C. parapsilosis cells exposed to the Cs2.5H0.5PW12O40 NPs (Fig. 5). Conversely, C. parapsilosis exposed to the nanoparticles did not show any morphological alteration in comparison to the control. Only an attachment of the tertiary structures of the Keggin salt to the cell wall was detected (Fig. 5e). Furthermore, we did not find internalization of the spherical tertiary structures or the 10 nm-sized primary particles. The TEM images in Fig. 5c–e demonstrate that the nanoparticles are not able to cross or weaken the cell wall structure of C. parapsilosis. This means that the Cs2.5H0.5PW12O40 NPs are unable to reach the cytosol, hence it cannot directly induce any internal physical damage to the cell. We propose that this result can be a common observation for all the fungal species that eventually interact with Cs2.5H0.5PW12O40 NPs. This hypothesis is further sustained by the susceptibility assays using A. fumigatus mutants for regulatory genes of the cell wall integrity (CWI) pathway (Fig. S1, ESI†). A common feature of these mutants is the defective maintenance of cell wall integrity, which ultimately makes the cells more susceptible to lysis or cell wall damaging agents.34,62 We observed similar levels of tolerance of the A. fumigatus wild-type and the CWI mutants towards the Cs2.5H0.5PW12O40 NPs supporting the previous finding in C. parapsilosis, i.e., the action of the Cs2.5H0.5PW12O40 NPs do not involve disruption of the cell wall integrity.
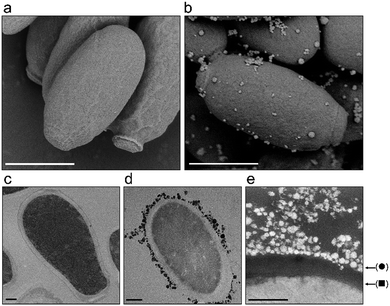 |
| Fig. 5 Electron microscopy images of C. parapsilosis cells. Secondary electron SEM image of the cells in (a) control and (b) exposed to Cs2.5H0.5PW12O40 NPs. Scale bars correspond to 2 μm. Bright-field TEM image of the cells in (c) control and (d) exposed to Cs2.5H0.5PW12O40 NPs, with magnification in the dark-field mode shown in (e). Scale bars correspond to 500 nm. Symbols: (●) Cs2.5H0.5PW12O40 NPs and (■) cell wall. | |
An antimicrobial mechanism regulated by H3O+
The high amount of acid sites in the Cs2.5H0.5PW12O40 NPs25,41 has the potential to be the main antimicrobial mechanism of this compound. To establish whether acid sites are important for the antimicrobial response of the Cs2.5H0.5PW12O40 NPs, the TPD-NH3 technique was employed to probe the relation between the surface acid sites and the antimicrobial response. First, we reduced the original concentration of the surface acid sites in the as-synthesized nanopowder through a heat treatment at 300 °C. This heat treatment decreases the number of acid sites by the loss of structural water molecules40 (Fig. S2, ESI†), and also restrains the mobility of the structural protons.63 The TPD-NH3 results (Fig. 6a and b) show that the total acid site concentration in the as-synthesized Cs2.5H0.5PW12O40 NPs is almost two times higher than that in the heat-treated powder. Moreover, a small concentration of strong acid sites (2.6 × 10−3 mmol g−1) disappear after the heat treatment. Both the results indicate that the as-synthesized Cs2.5H0.5PW12O40 NPs are more acidic than their heat-treated form. As a result, there is a considerable modification in the antimicrobial activity. As shown in Fig. 6c, the loss of surface acid sites is accompanied by a pronounced decrease in the antibacterial efficacy of the Cs2.5H0.5PW12O40 NPs. For all the bacterial strains, the MIC values increase between 0.75 and 1.00 mg mL−1 for the sample with a lowered concentration of surface acid sites. We can therefore assume that surface acidity is an important regulator for the antimicrobial effect in this Keggin salt.
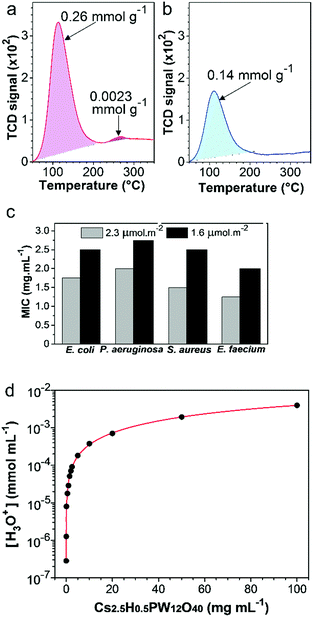 |
| Fig. 6 TPD-NH3 profiles of Cs2.5H0.5PW12O40 NPs, (a) as-synthesized and (b) heat treated at 300 °C for 2 h. (c) Dependence of the MIC values on the surface acid site concentration in Cs2.5H0.5PW12O40 NPs, where 2.3 and 1.6 μmol m−2 represent, respectively, the as-synthesized nanopowder and the powder heat treated at 300 °C for 2 h. (d) Concentration of H3O+ in aqueous suspensions containing several additions of the as-synthesized Cs2.5H0.5PW12O40 NPs. For this measurement, distilled water was selected as a standard medium, instead of the nutrient broth, because it is a more appropriate medium for accurately estimating the number of H3O+ released from Cs2.5H0.5PW12O40 NPs without interference of other organic compounds. Due to the buffering action, the acidification observed in aqueous suspension cannot be totally extrapolated to nutrient broths. | |
The apparent complex interrelationship between the surface acid sites and the antimicrobial response can be explained via the contribution of these acid sites to the generation and release of H3O+ ions into the media. As shown in Fig. 6d, we found an exponential growth of the H3O+ species in water as the concentration of the Cs2.5H0.5PW12O40 NPs increases. Obviously, a direct effect of the high protonation rate is the acidification of the environment in the presence of Cs2.5H0.5PW12O40 NPs. For instance, by simulating the MBC values of the Cs2.5H0.5PW12O40 NPs in aqueous suspensions, we found pH 3.8 for Gram-negative bacteria, 4.3–4.4 for Gram-positive bacteria, and 3.8–4.0 for yeasts. Surprisingly, these experimental values are close to the expected pH range for acid-induced death in the tested strains.16 In other words, the H3O+ ions released by the Cs2.5H0.5PW12O40 NPs can reduce the local pH to levels theoretically not supported for the tested strains and presumably for the majority of nosocomial pathogens.
Discussion
Our systematic description of the antimicrobial activity exerted by the Cs2.5H0.5PW12O40 NPs demonstrates that this compound offers some advantages over the current nano-antimicrobials. An exciting feature comes from its biocide effect with a broad spectrum of action against Gram-negative and Gram-positive bacteria, yeasts, and filamentous fungus. Although being a highly desired characteristic, a broad spectrum of action not always occurs in oxide-based nanomaterials. For instance, ZnO NPs are ineffective against Proteus vulgaris,15 in addition to the low efficacy of CuO NPs against Salmonella typhimurium, Aeromonas liquefaciens, P. aeruginosa, and Proteus spp.64–66 Another important feature of the Cs2.5H0.5PW12O40 NPs described here is the manifestation of biocide effect even with the susceptibility assays being strictly carried out under dark conditions. This suggests that the Cs2.5H0.5PW12O40 NPs do not require light or UV activation. Comparatively, the TiO2 NP-based antimicrobials regulated by ROS have been reported to be significantly less active under dark conditions.14 Also, the fact that the antimicrobial action of Cs2.5H0.5PW12O40 NPs has no dependence on the [PW12O40]3−, Cs+, and internalization of nanoparticles, is an important aspect that mitigates the toxicity of nano-antimicrobials towards mammalian cells and the environment.13,67 Nowadays, there are still some safety concerns regarding nano-antimicrobials with a mode of action mediated by the release of heavy metal ions or nanoparticles, such as, Ag NPs.68
One significant finding of our research is the relationship between the antimicrobial action of the Cs2.5H0.5PW12O40 NPs and the release of H3O+. Protonation in Keggin salts is a well-known intrinsic property induced by structural acid sites.69,70 Even a substantial replacement of the proton sites with Cs+ in the phosphotungstic heteropolyacid structure did not compromise the protonation ability of the resultant Cs2.5H0.5PW12O40 salt. On the contrary, the antimicrobial behaviour can take advantage of the marked increase in proton mobility when counter-cations are added to POMs.63 Furthermore, CsxH3−xPW12O40-based antimicrobials can be safely designed upon modulation of the extension of H3O+ release. The produced acidic environment (pH) can be adequately regulated to a desired level by controlling the replacement of the structural protons by the counter-cation Cs+,41 or in a more simple approach, by controlling the amount of the Keggin salt used (Fig. 6d).
Although not yet entirely described, the current mechanistic insight into the proton release by Keggin salts reveals that external oxygen atoms of the heteropolyanion are the active centers for H3O+ generation28,63,71 (see eqn (1)). Consequently, the acidic pH produced by the Cs2.5H0.5PW12O40 NPs has its origin in the interaction of the nanoparticle surface with water, and the subsequent release of H3O+ species to the environment.41,71 This release of protons is also facilitated by the small electrostatic attraction between the H3O+ and the big-sized heteropolyanion.24 Therefore, our observation of the superior antimicrobial activity of the Cs2.5H0.5PW12O40 NPs with a higher amount of surface acid sites supports a mode of action mediated by H3O+. Acid sites are fundamental structures for proton formation and motion.25,63 Thus, the physical meaning of the MBC values found in this work is an amount of Cs2.5H0.5PW12O40 NPs in which the release of H3O+ reaches an extent sufficient to decrease the local pH to levels not supported by the tested strains. Although the MBC values of the Cs2.5H0.5PW12O40 NPs are considerably higher than those reported for metal nanoparticles such as Ag (0.10 mg mL−1),64 the values shown in Table 2 are comparable to those found for metal oxide nanoparticles such as ZnO (2.50–5.00 mg mL−1)64 and TiO2 (>2.50 mg mL−1).72
| (–W–O–W–) + 3 H2O ⇆ 2 (–W–O)− + 2 H3O+ | (1) |
Since the majority of clinically relevant pathogens are neutralophiles, that is, pathogens with optimum growth responses only at near-neutral pH,73,74 the antimicrobial mechanism of Cs2.5H0.5PW12O40 NPs regulated by the release of H3O+ ions appears to be of great importance to combat HCAIs. As a rule, neutralophiles require a circumneutral cytoplasmic pH; however, they do not possess effective pH homeostasis mechanisms in extremely acidic environments.75 Targeting this specific weakness, the H3O+ ions released by the Cs2.5H0.5PW12O40 NPs can lead to an acid-induced death by lowering the cytoplasmic pH.76 Considering Fig. 5e, the Cs2.5H0.5PW12O40 NPs behave as a proton carrier anchored on the cell surface, releasing protons into the cytoplasm and other cell structures affecting the functionality of cell molecules. Thus, the antimicrobial susceptibility of the strains towards the Cs2.5H0.5PW12O40 NPs in the order: Gram-positive bacteria > Gram-negative bacteria > yeasts/filamentous fungus, seems to be associated with the effectiveness of the cell walls/cell membranes in pH homeostasis in each of these different species.73 For example, the superior resistance of yeasts to the action of Cs2.5H0.5PW12O40 NPs can be explained by the proton pump out mechanism.77 Yeasts can maintain the cytosolic pH neutral even when exposed to acidic environments due to a combined response of Pma1p and V-ATPase. Pma1p is a single 100 kDa polypeptide localized in the plasma membrane that pumps protons out of the cell. V-ATPase is responsible for pH homeostasis in organelles.78 However, the improbable adaptation of neutralophiles to an environment rich in H3O+ makes the Cs2.5H0.5PW12O40 NPs a low risk nano-antimicrobial agent of eliciting resistance. Even in the absence of aqueous medium, condensation of moisture present in the environment should be sufficient to create an aqueous interface for H3O+ diffusion from a surface or device containing Cs2.5H0.5PW12O40 NPs to the target pathogen.22 Furthermore, not only limited to the Cs2.5H0.5PW12O40 NPs, an antimicrobial mechanism regulated by H3O+ can be expected for other members of the vast family of water-insoluble POM compounds.
Conclusion
In summary, we demonstrated that Cs2.5H0.5PW12O40 NPs hold potential as a proton-regulated inorganic antimicrobial. The key phenomenon behind their antimicrobial performance is the formation of an inhospitable acid environment through the generation and release of H3O+. One important characteristic of the antimicrobial mechanism mediated by H3O+ is the biocide effect against neutralophiles. It supports the prospect of this nano-antimicrobial to combat several clinically relevant human pathogens, and, with potential for utilization against multidrug-resistant organisms. Overall, the Cs2.5H0.5PW12O40 NPs expand the possibilities for surface or medical device functionalization as an attempt to reduce the incidence of HCAIs.
Conflicts of interest
There are no conflicts to declare.
Acknowledgements
This work was supported by the São Paulo Research Foundation (FAPESP Grant no. 2016/16098-8 to R. H. P. and 2015/17541-0 to IM). R. H. P. thanks the postdoctoral program of the São Carlos Institute of Chemistry (IQSC) at the University of São Paulo (USP).
Notes and references
- B. J. Hensley and J. R. T. Monson, Surgery, 2015, 33, 528–533 Search PubMed.
- H. Arefian, S. Hagel, S. Heublein, F. Rissner, A. Scherag, F. M. Brunkhorst, R. J. Baldessarini and M. Hartmann, Am. J. Infect. Control, 2016, 44, 160–166 CrossRef PubMed.
- R. Sugden, R. Kelly and S. Davies, Nat. Microbiol., 2016, 1, 16187 CrossRef CAS PubMed.
- J. I. Barrasa-Villar, C. Aibar-Remón, P. Prieto-Andrés, R. Mareca-Doñate and J. Moliner-Lahoz, Clin. Infect. Dis., 2017, 65, 644–652 CrossRef PubMed.
-
J. O’Neill, Antimicrobial resistance: tackling a crisis for the health and wealth of nations, 2014.
- S. Garneau-Tsodikova and G. D. Wright, Med. Chem. Commun., 2016, 7, 10 RSC.
- World Health Organization, Worldwide country situation analysis: response to antimicrobial resistance, 2015.
- E. D. Brown and G. D. Wright, Nature, 2016, 529, 336–343 CrossRef CAS PubMed.
- A. C. Abreu, R. R. Tavares, A. Borges, F. Mergulhao and M. Simoes, J. Antimicrob. Chemother., 2013, 68, 2718–2732 CrossRef CAS PubMed.
- B. Cohen, C. C. Cohen, B. Løyland and E. Larson, Clin. Epidemiol., 2017, 9, 297–310 CrossRef PubMed.
- M. C. Sportelli, R. A. Picca and N. Cioffi, TrAC, Trends Anal. Chem., 2016, 84, 131–138 CrossRef CAS.
- L. Wang, C. Hu and L. Shao, Int. J. Nanomed., 2017, 12, 1227–1249 CrossRef PubMed.
- O. Bondarenko, K. Juganson, A. Ivask, K. Kasemets, M. Mortimer and A. Kahru, Arch. Toxicol., 2013, 87, 1181–1200 CrossRef CAS PubMed.
- T. P. Dasari, K. Pathakoti and H. M. Hwang, J. Environ. Sci., 2013, 25, 882–888 CrossRef CAS.
- M. Ramani, S. Ponnusamy, C. Muthamizhchelvan, J. Cullen, S. Krishnamurthy and E. Marsili, Colloids Surf., B, 2013, 105, 24–30 CrossRef CAS PubMed.
- M. Lackner, S. Maninger and J.-P. Guggenbichler, Nachr. Chem., 2013, 61, 112–115 CrossRef CAS.
- S. Shafaei, M. Lackner, R. Voloshchuk, I. Voloshchuk, J. Guggenbichler and C. Zollfrank, Recent Pat. Mater. Sci., 2014, 7, 26–36 CrossRef.
- C. Zollfrank, K. Gutbrod, P. Wechsler and J. P. Guggenbichler, Mater. Sci. Eng., C, 2012, 32, 47–54 CrossRef CAS PubMed.
- H. Schade and A. Marchionini, Klin. Wochenschr., 1928, 7, 12–14 CrossRef CAS.
- A. Zlotogorski, Arch. Dermatol. Res., 1987, 279, 398–401 CrossRef CAS.
- S. Ali and G. Yosipovitch, Acta Derm.-Venereol., 2013, 93, 261–267 CrossRef PubMed.
- S. Shafaei, D. Van Opdenbosch, T. Fey, M. Koch, T. Kraus, J. P. Guggenbichler and C. Zollfrank, Mater. Sci. Eng., C, 2016, 58, 1064–1070 CrossRef CAS PubMed.
- S. Shafaei, M. Lackner, M. Meier, J. Plank, J. P. Guggenbichler and C. Zollfrank, Surf. Innovations, 2013, 1, 202–208 CrossRef CAS.
- T. Okuhara, Chem. Rev., 2002, 102, 3641–3666 CrossRef CAS PubMed.
- M. Misono, Chem. Commun., 2001, 1141–1152 RSC.
- A. Corma, A. Martínez and C. Martínez, J. Catal., 1996, 164, 422–432 CrossRef CAS.
- T. Nakato, M. Kimura, S. Nakata and T. Okuhara, Langmuir, 1998, 14, 319–325 CrossRef CAS.
- I. V. Kozhevnikov, Chem. Rev., 1998, 98, 171–198 CrossRef CAS PubMed.
- T. Ito, K. Inumaru and M. Misono, J. Phys. Chem. B, 1997, 101, 9958–9963 CrossRef CAS.
- S. S. de Mello, D. Van Tyne, A. N. G. Dabul, M. S. Gilmore and I. L. B. C. Camargo, Genome Announc., 2016, 4, e00992 Search PubMed.
- M. E. da Silva Ferreira, M. R. V. Z. Kress, M. Savoldi, M. H. S. Goldman, A. Hartl, T. Heinekamp, A. A. Brakhage and G. H. Goldman, Eukaryotic Cell, 2006, 5, 207–211 CrossRef PubMed.
- P. A. Stadelmann, Ultramicroscopy, 1987, 21, 131–145 CrossRef CAS.
- R. Wright, Microsc. Res. Tech., 2000, 51, 496–510 CrossRef CAS PubMed.
- M. C. Rocha, J. H. T. M. Fabri, K. Franco de Godoy, P. Alves de Castro, J. I. Hori, A. Ferreira da Cunha, M. Arentshorst, A. F. J. Ram, C. A. M. J. J. van den Hondel, G. H. Goldman and I. Malavazi, G3: Genes, Genomes, Genet., 2016, 6, 2983–3002 Search PubMed.
- NCCLS, M38-A2|Reference Method for Broth Dilution Antifungal Susceptibility Testing of Filamentous Fungi, 2008.
- NCCLS, M27-A2: Reference Method for Broth Dilution Antifungal Susceptibility Testing of Yeasts, 2002.
- NCCLS, M7-A6: Methods for Dilution Antimicrobial Susceptibility Tests for Bacteria That Grow Aerobically, 2003.
-
E. Käfer, Advances in Genetics, 1977, vol. 19, pp. 33–131 Search PubMed.
- M. Sun, J. Zhang, P. Putaj, V. Caps, F. Lefebvre, J. Pelletier and J.-M. Basset, Chem. Rev., 2014, 114, 981–1019 CrossRef CAS PubMed.
-
T. Okuhara, N. Mizuno and M. Misono, Advances in Catalysis, 1996, pp. 113–252 Search PubMed.
- L. Matachowski, A. Zięba, M. Zembala and A. Drelinkiewicz, Catal. Lett., 2009, 133, 49–62 CrossRef CAS.
- N. R. Shiju, H. M. Williams and D. R. Brown, Appl. Catal., B, 2009, 90, 451–457 CrossRef CAS.
- R. Xing, F. Wang, L. Dong, A.-P. Zheng, L. Wang, W.-J. Su and T. Lin, Food Chem., 2016, 197, 205–211 CrossRef CAS PubMed.
- D. H. Piva, R. H. Piva, M. C. Rocha, J. A. Dias, O. R. K. Montedo, I. Malavazi and M. R. Morelli, Adv. Powder Technol., 2017, 28, 463–472 CrossRef CAS.
- L. Grama, A. Man, D.-L. Muntean, Şerban A. Gâz Florea, F. Boda and A. Curticăpean, Rom. Rev. Lab. Med., 2014, 22, 111–118 Search PubMed.
- T. Hu, Y.-H. Li, X.-F. Kuang and C.-Z. Lu, CrystEngComm, 2017, 19, 3445–3454 RSC.
- D. A. Friesen, L. Morello, J. V. Headley and C. H. Langford, J. Photochem. Photobiol., A, 2000, 133, 213–220 CrossRef CAS.
- T. Yamase, N. Fukuda and Y. Tajima, Biol. Pharm. Bull., 1996, 19, 459–465 CAS.
- S. Balici, M. Niculae, E. Pall, M. Rusu, D. Rusu and H. Matei, Rev. Chim., 2016, 67, 485–490 CAS.
- Editorial, Nat. Microbiol., 2017, 2, 17120 CrossRef PubMed.
- K. J. Kwon-Chung and J. A. Sugui, PLoS Pathog., 2013, 9, e1003743 Search PubMed.
- T. Heinekamp, H. Schmidt, K. Lapp, V. Pähtz, I. Shopova, N. Köster-Eiserfunke, T. Krüger, O. Kniemeyer and A. A. Brakhage, Semin. Immunopathol., 2015, 37, 141–152 CrossRef CAS PubMed.
- N. B. Saleh, D. J. Milliron, N. Aich, L. E. Katz, H. M. Liljestrand and M. J. Kirisits, Sci. Total Environ, 2016, 568, 926–932 CrossRef CAS PubMed.
- X.-X. Lu, Y.-H. Luo, C. Lu, X. Chen and H. Zhang, J. Solid State Chem., 2015, 232, 123–130 CrossRef CAS.
- L. Wang, W. Yang, W. Zhu, X. Guan, Z. Xie and Z. Sun, Inorg. Chem., 2014, 53, 11584–11588 CrossRef CAS PubMed.
- S. Hu, C. Ma, F. Zhan, Y. Cao, P. Hu and Q. Zhen, Chem. Pap., 2017, 71, 1323–1329 CrossRef CAS.
- J. He, H. Pang, W. Wang, Y. Zhang, B. Yan, X. Li, S. Li and J. Chen, Dalton Trans., 2013, 42, 15637 RSC.
- A.-L. Kubo, L. Kremer, S. Herrmann, S. G. Mitchell, O. M. Bondarenko, A. Kahru and C. Streb, ChemPlusChem, 2017, 82, 867–871 CrossRef CAS.
- I. Malavazi, G. H. Goldman and N. A. Brown, Briefings Funct. Genomics, 2014, 13, 456–470 CrossRef PubMed.
- A. C. R. Souza, B. B. Fuchs, H. M. S. Pinhati, R. A. Siqueira, F. Hagen, J. F. Meis, E. Mylonakis and A. L. Colombo, Antimicrob. Agents Chemother., 2015, 59, 6581–6587 CrossRef CAS PubMed.
- H. K. Daima, P. R. Selvakannan, A. E. Kandjani, R. Shukla, S. K. Bhargava and V. Bansal, Nanoscale, 2014, 6, 758–765 RSC.
- M. C. Rocha, K. F. de Godoy, P. A. de Castro, J. I. Hori, V. L. P. Bom, N. A. Brown, A. F. da Cunha, G. H. Goldman and I. Malavazi, PLoS One, 2015, 10, e0135195 Search PubMed.
- E. A. Ukshe, L. S. Leonova and A. I. Korosteleva, Solid State Ionics, 1989, 36, 219–223 CrossRef CAS.
- G. Ren, D. Hu, E. W. C. Cheng, M. a. Vargas-Reus, P. Reip and R. P. Allaker, Int. J. Antimicrob. Agents, 2009, 33, 587–590 CrossRef CAS PubMed.
- D. Das, B. C. Nath, P. Phukon and S. K. Dolui, Colloids Surf., B, 2013, 101, 430–433 CrossRef CAS PubMed.
- T. Pandiyarajan, R. Udayabhaskar, S. Vignesh, R. A. James and B. Karthikeyan, Mater. Sci. Eng., C, 2013, 33, 2020–2024 CrossRef CAS PubMed.
- M. Heinlaan, A. Ivask, I. Blinova, H. C. Dubourguier and A. Kahru, Chemosphere, 2008, 71, 1308–1316 CrossRef CAS PubMed.
- M. Moritz and M. Geszke-Moritz, Chem. Eng. J., 2013, 228, 596–613 CrossRef CAS.
- I. V. Kozhevnikov, J. Mol. Catal. A: Chem., 2007, 262, 86–92 CrossRef CAS.
- I. V. Kozhevnikov, J. Mol. Catal. A: Chem., 2009, 305, 104–111 CrossRef CAS.
- A. I. Chikin, A. V. Chernyak, Z. Jin, Y. S. Naumova, A. E. Ukshe, N. V. Smirnova, V. I. Volkov and Y. A. Dobrovolsky, J. Solid State Electrochem., 2012, 16, 2767–2775 CrossRef CAS.
- M. A. Vargas-Reus, K. Memarzadeh, J. Huang, G. G. Ren and R. P. Allaker, Int. J. Antimicrob. Agents, 2012, 40, 135–139 CrossRef CAS PubMed.
-
J. L. Slonczewski, M. Fujisawa, M. Dopson and T. A. Krulwich, in Advances in Microbial Physiology, Elsevier, 2009, vol. 55, pp. 1–317 Search PubMed.
- T. A. Krulwich, G. Sachs and E. Padan, Nat. Rev. Microbiol., 2011, 9, 330–343 CrossRef CAS PubMed.
- C. Baker-Austin and M. Dopson, Trends Microbiol., 2007, 15, 165–171 CrossRef CAS PubMed.
- J. W. Foster and H. K. Hall, J. Bacteriol., 1991, 173, 5129–5135 CrossRef CAS PubMed.
- R. Orij, S. Brul and G. J. Smits, Biochim. Biophys. Acta, Gen. Subj., 2011, 1810, 933–944 CrossRef CAS PubMed.
- U. Nehls and S. Dietz, Appl. Microbiol. Biotechnol., 2014, 98, 8835–8851 CrossRef CAS PubMed.
-
J. B. Moffat, Metal-Oxygen Clusters – The Surface and Catalytic Properties of Heteropoly Oxometalates, 1st edn, Springer, US, Boston, 2001 Search PubMed.
Footnotes |
† Electronic supplementary information (ESI) available. See DOI: 10.1039/c7tb02763j |
‡ These authors contributed equally to this work. |
§ In the solid state, POM compounds present hierarchical formation divided into primary, secondary, and tertiary structures. More information about this concept can be found in the paper of Misono.25 |
|
This journal is © The Royal Society of Chemistry 2018 |
Click here to see how this site uses Cookies. View our privacy policy here.