DOI:
10.1039/C6RA27012C
(Review Article)
RSC Adv., 2017,
7, 2281-2287
A mini review of the synthesis of poly-1,2,3-triazole-based functional materials
Received
18th November 2016
, Accepted 5th December 2016
First published on 12th January 2017
Abstract
As a class of nitrogenous heterocyclic compounds, poly-1,2,3-triazole-based functional materials have recently attracted growing attention across many important scientific areas such as the synthesis of drugs and functional materials. There is therefore an urgent need to perform a review study of such an important class of materials for summarizing the state-of-the-art contributions and for clarifying the direction of the most recent advances (mostly in 2016). Herein, from the perspective of raw material selection and synthetic processes, we conduct a mini review study of the most recent new findings, including technologies and strategies, on the synthesis of macromolecular 1,2,3-triazole-based functional materials, such as various state-of-the-art approaches based on click chemistry and optimization of synthetic conditions like selection of raw materials, control of their concentrations, and selective utilization of different active groups. This mini review also briefly introduces the progress of using poly-1,2,3-triazole-based materials for a broad range of applications including molecular recognition, chemical sensing, drug chemistry, bio-chemistry, and conducting materials, etc.
1. Introduction
Over the past few decades, 1,2,3-triazoles have been widely employed as a linker and a functional moiety, primarily because of their appealing “Click” properties, orthogonality enhancement, and processing simplicity.1 As a consequence, their wide-ranging applications have also been explored, e.g. bactericides and conditioning agents for plant growth. Due to the extensive application prospects of 1,2,3-triazoles, considerable attention has been recently paid to the synthesis of functional macromolecules containing building units of 1,2,3-triazoles.2
As early as 2002, Demko and Sharpless reported the first synthesis of poly-1,2,3-triazole that showed powerful utility in further preparation of new functional materials.3 Indeed, the presence of poly-1,2,3-triazole units in macromolecular structures may lead to the generation of cooperative linkers or units.4 A wide spectrum of potential applications was also expected for poly-1,2,3-triazole-based functional materials, such as supermolecules, fluorescence probes, and biological proteins.5
Comparing to conventional 1,2,3-triazoles-based organic functional molecules,6 poly-1,2,3-triazole-based functional materials have shown more widespread applications (owing to their superior properties), including DNA chemistry, self-assembly, surface modification, supramolecular chemistry, combination chemistry, and dendrimer chemistry, as well as functional macromolecules and biomedical applications.7 In this mini review, the synthesis of poly-1,2,3-triazole-based functional materials in most recent years (mostly 2016) are systematically summarized in terms of different synthetic processes and raw material classification.
2. Influence of the type of acetylenic compounds
2.1. Synthesis based on the reaction between polyazide and mono-alkyne
On the basis of widely-reported propargyl alcohol 1 shown in Scheme 1 (as a substrate)-based click reactions, many poly-1,2,3-triazole-based functional materials have been synthesized. For example, propargyl alcohol was selected as a raw material for the synthesis of a macromolecular poly-triazole.8 The productivity of synthesizing macromolecular triazole-based materials could be further improved by employing microwave (MW) technology and propargyl ester 2 (see Scheme 1) as a raw material, resulting in a short period of synthesis (from 2 h to 4 min) as well as a very high production yield.
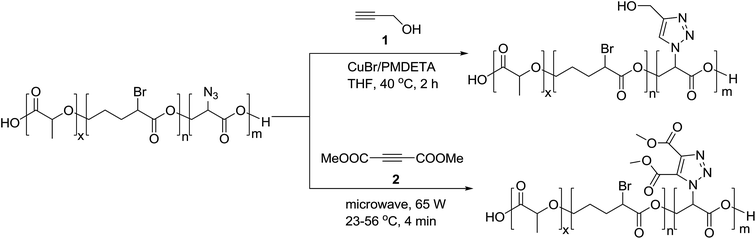 |
| Scheme 1 Synthesis of poly-1,2,3-triazole by a microwave method. | |
Most recently, Chen et al.9 modified guanosine triphosphate (GTP) derivatives based on glycosyl, producing a ribose-like mono-acetylene compound (see compound 3) that was further used to synthesize a series of block copolymers. Systematic characterizations have revealed that these products could be further manufactured into a supramolecular film for applications in high-performance storage devices. Meanwhile, Tao group10 introduced glucose units 4, as shown in Fig. 1, into a macromolecular triazole for enhancing the solubility of the target compound. The alkyl chain length of the thus-obtained polymer showed a significant impact on the antimicrobial activity, according to the antitumor activity measurement. Furthermore, the three components of the synthetic reaction could react with each other in a neutral aqueous solution through a one-pot tandem reaction, demonstrating a new synthetic route to macromolecular bis-triazoles for biomedical applications (such as those involving new anti-cancer drugs). On the other hand, Mindt et al.11 incorporated many O- and N-containing functional groups onto a peptide acetylene compound 5 through a click reaction, making full use of the triazole moiety as a stable bridging amide bond mimic. The final product with multiple triazole substitutions shows promising biological applications, and case-by-case investigation is expected to be done.
 |
| Fig. 1 Some alkynes involved in the synthesis of poly-1,2,3-triazoles. | |
A series of macromolecular triazoles were also synthesized using a mono-alkyne compound 6 (see Fig. 1), further forming nanoparticles by self-assembly with polyethylene glycol (PEG). The prepared nano-materials containing triazole units have potential applications for, e.g., controlled drug release and anti-cancer drug delivery applications. Such a design and synthesis protocol offers a general approach to produce nano-materials and many responsive materials, and also provides a research direction for intelligent delivery of drugs and for cancer therapy.12
Uyanik group13 took advantage of pyrene (that is a fluorescence group) to synthesize a triazole-based polymer using a pyrene-containing alkyne 7 (see Fig. 1) through a click reaction. Such a prepared polymer was further endowed with a liquid crystal property. Unexpectedly, the fluorescence intensity of the products decreases with increasing molecular weight, as it restricts the interaction between pyrene moieties incorporated into the synthesized products. The polymer is expected to possess drug delivery applications as biocompatible and degradable materials.
Apart from biomedical applications, macromolecular poly-1,2,3-triazoles also show promising battery applications. For example, in order to avoid electrolyte leakage and carbon dioxide poisoning, Li group14 constructed a triazole module (see Scheme 2) based on a click reaction, followed by attaching to an anion-exchange membrane. This triazole module-containing polymer membrane exhibited a markedly enhanced stability to an alkaline electrolyte, as compared with traditional membranes. This work affords a new strategy of design and utilization of anion-exchange membranes.
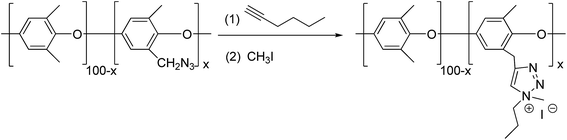 |
| Scheme 2 Synthesis of poly-1,2,3-triazole for anion-exchange membrane applications. | |
2.2. Synthesis based on the reaction between diazide and diyne
When diyne and diazide were adopted as raw materials in double click reactions, a macromolecular poly-triazole with different morphologies could be effectively prepared by controlling the reactant ratio, which was connected by triazole ring modules. For example, Xie group15 employed a diyne compound 8 (see Fig. 2) as a raw material and consequently synthesized hyperbranched poly-triazoles through a click reaction. After further alkylation, the synthesized poly-triazoles turned out to be anion-exchangeable, together with a significant increase of electrical conductivity, thereby presenting promising applications in new-type electrolyte materials.
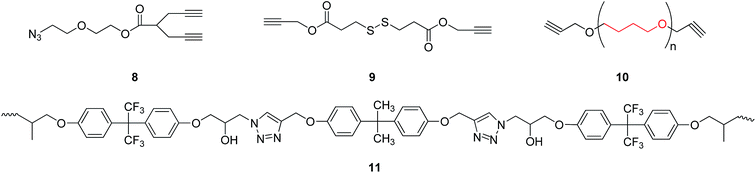 |
| Fig. 2 Some dialkynes and corresponding products correlated to the synthesis of poly-1,2,3-triazoles. | |
Likewise, Lim group16 prepared a polymer with poly-triazole units using a diyne compound 9 (see Fig. 2) as a substrate through a one-pot reaction of three components in water without involving alkaline environment, on the basis of click-coupling reactions. Such a polymer exhibited a pH-responsive property and could rapidly decompose disulfide groups under micelle action conditions.
Hydroxyl-type chelating agents, based on poly-triazoles, have also been widely applied in solid fuel cells. For example, Reshmi et al.17 selected some O-containing diyne compounds (see the compound 10 shown in Fig. 2) for the reaction with polyazide through a click reaction, leading to the generation of a polymer with a network structure. Comparing to conventional polyurethane-based chelating agents, this polymer with the network structure showed a superior manufacturability, especially for use as binders in solid rocket fuels. Furthermore, Kumar et al.18 fabricated a temperature-sensitive shape-memory material (polymer 11, as shown in Fig. 2) by a simple method without the protection of inert atmosphere. The measurements of mechanical properties revealed that the shape recovery ratio of the shape-memory linear polymer could reach 95%, with a shape retention ratio being as high as 99%.
3. Influence of the type of azide compounds
3.1. Synthesis based on the reaction between poly-alkyne and mono-azide
Concerning some mono-azides, the macromolecular poly-triazoles can be easily synthesized using an excess amount of mono-azides through click reactions, due to the structural differences in the environment, as induced by alkyne ends. For instance, Hein et al.19 realized a highly efficient click reaction in a dimethylsulfoxide (DMSO) aqueous solution by selecting a mono-azide compound 12 (see Fig. 3) as a substrate, forming macromolecular poly-triazoles, with a high production yield. Based on a series of reactions and measurements, DNA destruction could be surprisingly inhibited by the addition of DMSO; in the meanwhile, the hydrophilicity of the poly-triazoles was improved, which could have many bio-friendly applications in both the in vivo and in vitro manners.
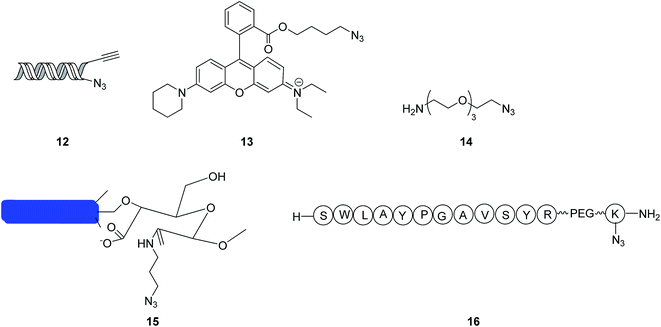 |
| Fig. 3 Some azides used for the synthesis of poly-1,2,3-triazoles. | |
Recently, Sacchetti group20 synthesized a macromolecular poly-triazole using a rhodamine derivative 13 (as shown in Fig. 3) as a substrate through a click reaction. To enhance the water solubility, the backbone of the polymer was incorporated with more nitrogen atoms. The polymer thus-obtained was effectively applied to drug and gene deliveries. The gelling test further verified that these integrated macromolecular poly-triazoles possessed cell recognition functions, thus promising the biomolecular labelling applications.
In the absence of any ligands, the click reaction between an alkyne compound (containing curcumin) and a mono-azide compound 14 shown in Fig. 3 (containing multiple CH2CH2O chain units) was also reported to produce a triazole-based linear polymer with the production yield of 70–80%. Because of its excellent biocompatibility and strong complexation interactions with heavy metal ions such as Cd2+, Cu2+ and Pb2+, the synthesized polymer shows promising applications in processing wastewater.21 On the other hand, a macromolecular poly-triazole was synthesized using a mono-azide compound 15 as presented in Fig. 3 (showing a rod-like cellulose structure) as a substrate through a click reaction.22 The effective synthesis of the stable polymer was based on the dicarboxylic cellulose and self-assembly chemistries. The atomic force microscopy (AFM) measurement showed a crosslinked nanofiber structure of the polymer, with an average width of 6 nm and length of 1 μm.
When a click reaction proceeded with an amino acid-based azide compound 16 (see Fig. 3) as a substrate, the finally prepared macromolecular poly-triazole could be used for labeling proteins. Meanwhile, the byproduct generated from the click reaction, namely dehydroascorbic acid, was demonstrated to be an indictor for monitoring oxidation interactions with soft tissues which was ascertained to suffer from an initial saccharification.23
In addition to the above-mentioned click reactions based on the mono-azides, strain-promoted alkyne–azide cycloaddition (SPAAC) reactions have also attracted attention for the synthesis of macromolecular poly-triazoles without using additional reagents. This approach is particularly useful for the modification of proteins in living cells, labelling of oligonucleotides, and other biochemical applications.24 Sutliff et al.25 synthesized a macromolecular poly-triazole with multiple ether bonds and other hydrophilic groups (see Scheme 3) using an acetylide (containing a cyclooctyne structure) and a mono-azide compound 17 as raw materials through the SPAAC reaction. This would facilitate the development of drugs being capable of specific targeting to, e.g., Escherichia coli and its derivatives.
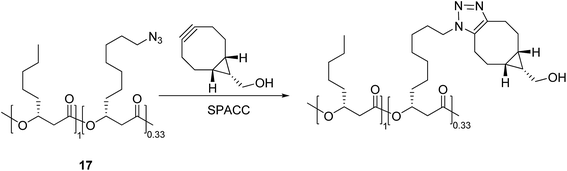 |
| Scheme 3 Synthesis of poly-1,2,3-triazole through the SPAAC reaction. | |
3.2. Synthesis based on the reaction between poly-alkyne and diazide
The nucleophilic substitution reaction between dihalide and sodium azide could first lead to the generation of an intermediate product 18 (see Fig. 4) of an organic diazide. The following reaction with alkyne could produce macromolecular poly-triazoles by a click reaction. For instance, a poly-triazole was successfully produced by a three-component reaction in an aqueous solution using monovalent copper as the catalyst and tripropargylamine as the crosslinking agent.26 The reaction product could be easily separated, without using reductants or oxygen protection during the process. It was also noted that the decrease of molecular weight of the polymer featured a one-step degradation. The thermal degradation properties of the polymer was found to be dependent on the length of the polyether chains, but not on the triazole linker. Additionally, for improving the mechanical properties and abrasive resistance of a polymer, a new-type, high-efficiency, fast, stable and safe crosslinking–coupling method was explored to insert a triazole module into the polymer.27 Using a diazide compound 19 (as shown in Fig. 4) as a substrate, the resulting water-soluble poly-triazole showed significantly improved mechanical strength, with the tensile strength increasing from 0.43 to 6.47 MPa, the Young's modulus raised to 40 MPa, and hardness reaching 73.1 MPa.
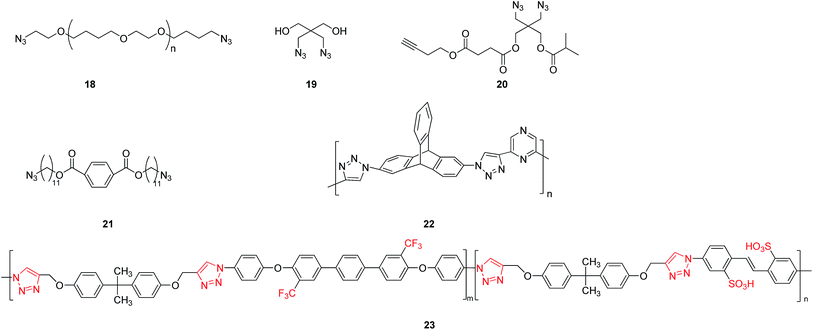 |
| Fig. 4 Some diazides and corresponding products involved in the synthesis of poly-1,2,3-triazoles. | |
On the other hand, the macromolecular poly-triazoles can be used as soft materials when prepared using a special diazide compound 20 having terminal alkynes (see Fig. 4) through a click reaction. Importantly, the polymer can be synthesized by a simple, efficient, fast one-pot reaction without any purification processes.28
Similarly, a chain-like diazide compound 21 (see Fig. 4) could also be used as a raw material for construction of triazole modules and then formation of dendrimer-like liquid crystal.29 Various characterizations including a polarizing microscopy, etc., indicated that the dendritic polymer exhibited a columnar nematic liquid crystal structure. This finding thus opens up a new avenue to liquid crustal materials.
By considering that triptycene is a fluorescence group, and that nitrogen heterocycle of pyrazine can function as the polymer backbone and offers multiple sites, Das group30 fabricated a new-type poly-triazole-based fluorecnence sensor (polymer 22 shown in Fig. 4) using a triptycene-based diazide compound and a pyrazine-based acetylide compound as raw materials by a click reaction. Such a fluorescence sensor contained abundant nitrogen atoms and was rather sensitive to some aromatic compounds, even reaching a ppm level. This work provides a new method for ultra-sensitive fluorescence detection.
When a substrate containing trifluoromethyl groups is employed, the finally synthesized poly-triazole can then be suitable for applications in proton-exchange areas. For example, using a click reaction to build a triazole bridge, a diazide was first incorporated with multiple trifluoromethyl groups, followed by the final production of a poly-triazole membrane (polymer 23 shown in Fig. 4).31 This study also demonstrated that the oxidation resistance of the prepared polymer membrane was enhanced by incorporation of sulfonic acid, triazole and trifluoromethyl groups. Comparing to the conventional Nafion 117 membrane, it also exhibited superior proton-exchange properties, with a proton conductivity as high as 12–76 ms cm−1.
3.3. Synthesis based on the reaction between mono-alkyne and poly-azide
To avoid troubles caused by protection treatment, the organic synthesis of poly-triazoles can also be realized using a poly-azide compound as a raw material. Apparently, when the adopted poly-azide compound involves glycosyl modification, the resultant poly-triazole turns out to be water-soluble, regardless of the starting alkyne materials used. For instance, a poly-azide compound 24 (as presented in Fig. 5) as a raw material was employed to the synthesis of poly-triazole by a click reaction.32 According to the biological activity test, the synthesized poly-triazole showed an antimicrobial activity towards Escherichia coli and Staphylococcus aureus. Likewise, using a poly-azide compound 25 (shown in Fig. 5) as a raw material, a hyperbranched poly-triazole could be finally produced with the assistance of MW, which had potential practical applications as a new-type fluorescence molecular probe.33 Such a probe was water-soluble and sensitive to the heavy metal and transition metal ions. Under pH 7.0 solution conditions, it showed a high selectivity to Zn2+ in terms of fluorescence enhancement among many other bio-related metal ions. In addition, it could also be used to detect different organic solvents under visible light irradiation, such as acetonitrile and others. Furthermore, a soybean oil-like poly-azide compound 26 (shown in Fig. 5) was also employed as a substrate to incorporate triazole, ester, and other functional groups by a catalyst-free click reaction.34 The mechanical properties of the as-synthesized polymer could be improved dramatically. Besides, the antibacterial activity test revealed that the triazole groups of the obtained polymer had a great impact on the antibacterial activity.
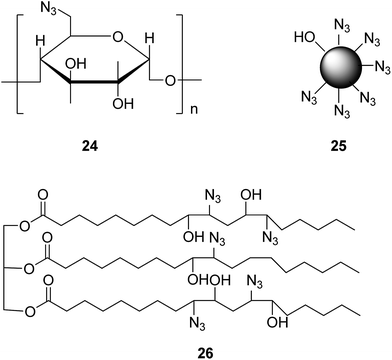 |
| Fig. 5 Some multi-azides used for the synthesis of poly-1,2,3-triazoles. | |
4. Conclusion & perspectives
All in all, with the help of click reactions, various macromolecular poly-triazoles have been synthesized by combination of different groups, of which the structure and function can be varied. The present review has presented versatile poly-1,2,3-triazole-based functional materials which have attracted growing attention all over the world and are believed to be a hot topic in the coming years, especially in exploration and development of synthetic routes.35 However, to further expand the applications of poly-1,2,3-triazoles in the areas of drug synthesis, ion recognition, chemicobiology, supramolecular chemistry and others, much work still remains.11,36 The mini review presented here can hopefully serve as a guide to better design and fabrication of functional poly-1,2,3-triazoles for addressing the problems existing in scientific communities and real-world industries.
Although a significant advance has been achieved for synthesis and potential applications of poly-1,2,3-triazole-based functional materials in the past few decades, there still exist a number of challenges.37 On the one hand, how to precisely control the stoichiometric ratio of the monomers alkyl and azide during the synthesis, since this is highly important for obtaining poly-1,2,3-triazole-based functional materials with high performance, as well as with promising and useful applications. On the other hand, practical applications of these poly-1,2,3-triazole-based functional materials are still in its infancy and much work remains to realizing large-scale production and applications. Despite the fact that poly-1,2,3-triazole-based functional materials have found a wide spectrum of potential applications since their first effective synthesis; however only few practical applications have been developed so far. Finally, we also speculate that a breakthrough in synthetic methodologies is highly desirable to further promote the development of the poly-1,2,3-triazole-based functional materials for various practical applications on a large scale.
Acknowledgements
The authors greatly appreciate the National Natural Science Foundation of China (No. 21671038), the special funding project of the technical innovation of Foshan city (No.: 2014AG10009), the public welfare research and capacity building project of Guangdong Province (2015A030401106) and the self-innovation promotion project of the universities in Guangdong province (No.: 2015KQNCX178).
Notes and references
-
(a) Y. Bourne, K. B. Sharpless, P. Taylor and P. Marchot, J. Am. Chem. Soc., 2016, 138, 1611–1621 CrossRef CAS PubMed;
(b) W. Zhai, B. M. Chapin, A. Yoshizawa, H.-C. Wang, S. A. Hodge, T. D. James, E. V. Anslyn and J. S. Fossey, Org. Chem. Front., 2016, 3, 918–928 RSC;
(c) I. E. Valverde, S. Vomstein and T. L. Mindt, J. Med. Chem., 2016, 59, 3867–3877 CrossRef CAS PubMed;
(d) H. Hu, J. H. Xin, H. Hu, X. Wang, D. Miao and Y. Liu, J. Mater. Chem. A, 2015, 3, 11157–11182 RSC.
-
(a) D. Gangaprasad, J. P. Raj, T. Kiranmye, S. S. Sadik and J. Elangovan, RSC Adv., 2015, 5, 63473–63477 RSC;
(b) H. Naeimi, V. Nejadshafiee and S. Masoum, RSC Adv., 2015, 5, 15006–15016 RSC;
(c) A. B. Lopes, P. Wagner, R. O. M. A. de Souza, N. L. Germain, J. Uziel, J.-J. Bourguignon, M. Schmitt and L. S. M. Miranda, J. Org. Chem., 2016, 81, 4540–4549 CrossRef CAS PubMed;
(d) Z. Monasterio, A. Irastorza, J. I. Miranda and J. M. Aizpurua, Org. Lett., 2016, 18, 2511–2514 CrossRef CAS PubMed;
(e) J. Chen, M. A. van Dongen, R. L. Merzel, C. A. Dougherty, B. G. Orr, A. K. Kanduluru, P. S. Low, E. N. G. Marsh and M. M. Banaszak Holl, Biomacromolecules, 2016, 17, 922–927 CrossRef CAS PubMed;
(f) H. Hu, J. H. Xin, H. Hu, X. Wang and Y. Kong, Appl. Catal., A, 2015, 492, 1–9 CrossRef CAS;
(g) H. Hu, X. Wang, D. Miao, Y. Wang, C. Lai, Y. Guo, W. Wang, J. H. Xin and H. Hu, Chem. Commun., 2015, 51, 16699–16702 RSC;
(h) F. Jalilian, B. Yadollahi, S. Tangestaninejad and H. A. Rudbari, RSC Adv., 2016, 6, 13609–13613 RSC;
(i) H. Hu, J. H. Xin, H. Hu and X. Wang, Nano Res., 2015, 8, 3992–4006 CrossRef CAS.
- Z. P. Demko and K. B. Sharpless, Angew. Chem., Int. Ed., 2002, 41, 2113–2116 CrossRef CAS PubMed.
- J. Kalisiak, K. B. Sharpless and V. V. Fokin, Org. Lett., 2008, 10, 3171–3174 CrossRef CAS PubMed.
- P. Wu, M. Malkoch, J. N. Hunt, R. Vestberg, E. Kaltgrad, M. G. Finn, V. V. Fokin, K. B. Sharpless and C. J. Hawker, Chem. Commun., 2005, 5775–5777, 10.1039/b512021g.
- J.-P. Huo, J.-C. Luo, W. Wu, J.-F. Xiong, G.-Z. Mo and Z.-Y. Wang, Ind. Eng. Chem. Res., 2013, 52, 11850–11857 CrossRef CAS.
-
(a) X. Hou, C. Ke and J. Fraser Stoddart, Chem. Soc. Rev., 2016, 45, 3766–3780 RSC;
(b) Y. Shi, X. Cao and H. Gao, Nanoscale, 2016, 8, 4864–4881 RSC.
- C. Wright, A. Banerjee, X. Yan, W. K. Storms-Miller and C. Pugh, Macromolecules, 2016, 49, 2028–2038 CrossRef CAS.
- H.-S. Sun, Y. Chen, W.-Y. Lee, Y.-C. Chiu, T. Isono, T. Satoh, T. Kakuchi and W.-C. Chen, Polym. Chem., 2016, 7, 1249–1263 RSC.
- C. Fu, A. Bongers, K. Wang, B. Yang, Y. Zhao, H. Wu, Y. Wei, H. T. T. Duong, Z. Wang and L. Tao, Polym. Chem., 2016, 7, 546–552 RSC.
- A. Mascarin, I. E. Valverde and T. L. Mindt, Med. Chem. Commun., 2016, 7, 1640–1646 RSC.
- X. An, A. Zhu, H. Luo, H. Ke, H. Chen and Y. Zhao, ACS Nano, 2016, 10, 5947–5958 CrossRef CAS PubMed.
- S. Ilkar Erdagi, E. Doganci, C. Uyanik and F. Yilmaz, React. Funct. Polym., 2016, 99, 49–58 CrossRef CAS.
- L. Liu, S. He, S. Zhang, M. Zhang, M. D. Guiver and N. Li, ACS Appl. Mater. Interfaces, 2016, 8, 4651–4660 CAS.
- J. Wu, J. Chen, J. Wang, X. Liao, M. Xie and R. Sun, Polym. Chem., 2016, 7, 633–642 RSC.
- X. T. Cao, Y. H. Kim, J. M. Park and K. T. Lim, Eur. Polym. J., 2016, 78, 264–273 CrossRef CAS.
- S. Reshmi, H. Hemanth, S. Gayathri and C. P. Reghunadhan Nair, Polymer, 2016, 92, 201–209 CrossRef CAS.
- K. S. S. Kumar, B. Sreelakshmi and C. P. R. Nair, Mater. Lett., 2014, 137, 315–318 CrossRef CAS.
- G. R. Abel, Z. A. Calabrese, J. Ayco, J. E. Hein and T. Ye, Bioconjugate Chem., 2016, 27, 698–704 CrossRef CAS PubMed.
- E. Mauri, I. Moroni, L. Magagnin, M. Masi, A. Sacchetti and F. Rossi, React. Funct. Polym., 2016, 105, 35–44 CrossRef CAS.
- A. Averick, S. Dolai, A. Punia, K. Punia, S. R. Guariglia, W. L'Amoreaux, K.-l. Hong and K. Raja, React. Funct. Polym., 2016, 102, 47–52 CrossRef CAS.
- H. Yang and T. G. M. van de Ven, Biomacromolecules, 2016, 17, 2240–2247 CrossRef CAS PubMed.
- A. C. Conibear, K. Farbiarz, R. L. Mayer, M. Matveenko, H. Kählig and C. F. W. Becker, Org. Biomol. Chem., 2016, 14, 6205–6211 CAS.
-
(a) G. Mohammadi Ziarani, Z. Hassanzadeh, P. Gholamzadeh, S. Asadi and A. Badiei, RSC Adv., 2016, 6, 21979–22006 RSC;
(b) C. Mari, S. Mosberger, N. Llorente, S. Spreckelmeyer and G. Gasser, Inorg. Chem. Front., 2016, 3, 397–405 RSC;
(c) R. R. Ramsubhag and G. B. Dudley, Org. Biomol. Chem., 2016, 14, 5028–5031 RSC.
- A. Pinto, J. H. Ciesla, A. Palucci, B. P. Sutliff and C. T. Nomura, ACS Macro Lett., 2016, 5, 215–219 CrossRef CAS.
- J. Zhai, N. Zhang, X. Guo, J. He, D. Li and R. Yang, Eur. Polym. J., 2016, 78, 72–81 CrossRef CAS.
- J. Hu, K. Peng, J. Guo, D. Shan, G. B. Kim, Q. Li, E. Gerhard, L. Zhu, W. Tu, W. Lv, M. A. Hickner and J. Yang, ACS Appl. Mater. Interfaces, 2016, 8, 17499–17510 CAS.
- X. Cao, Y. Shi, X. Wang, R. W. Graff and H. Gao, Macromolecules, 2016, 49, 760–766 CrossRef CAS.
- S. Guerra, T. L. A. Nguyen, J. Furrer, J.-F. Nierengarten, J. Barberá and R. Deschenaux, Macromolecules, 2016, 49, 3222–3231 CrossRef CAS.
- S. Mondal and N. Das, Polymer, 2015, 75, 109–118 CrossRef CAS.
- A. Singh, R. Mukherjee, S. Banerjee, H. Komber and B. Voit, J. Membr. Sci., 2014, 469, 225–237 CrossRef CAS.
- W. Tan, Q. Li, H. Wang, Y. Liu, J. Zhang, F. Dong and Z. Guo, Carbohydr. Polym., 2016, 142, 1–7 CrossRef CAS PubMed.
- S. Medel, P. Bosch, I. Grabchev, M. C. de la Torre and P. Ramírez, Eur. Polym. J., 2016, 74, 241–255 CrossRef CAS.
- H. Gholami, H. Yeganeh, R. Gharibi, M. Jalilian and M. Sorayya, Polymer, 2015, 62, 94–108 CrossRef CAS.
-
(a) Z. Meng, J.-F. Xiang and C.-F. Chen, J. Am. Chem. Soc., 2016, 138, 5652–5658 CrossRef CAS PubMed;
(b) L. Mendecki, X. Chen, N. Callan, D. F. Thompson, B. Schazmann, S. Granados-Focil and A. Radu, Anal. Chem., 2016, 88, 4311–4317 CrossRef CAS PubMed;
(c) A. Zarnegaryan, M. Moghadam, S. Tangestaninejad, V. Mirkhani and I. Mohammadpoor-Baltork, Polyhedron, 2016, 115, 61–66 CrossRef CAS.
-
(a) S. Chassaing, V. Bénéteau and P. Pale, Catal. Sci. Technol., 2016, 6, 923–957 RSC;
(b) L. R. Yule, R. L. Bower, H. Kaur, R. Kowalczyk, D. L. Hay and M. A. Brimble, Org. Biomol. Chem., 2016, 14, 5238–5245 RSC.
-
(a) V. K. Tiwari, B. B. Mishra, K. B. Mishra, N. Mishra, A. S. Singh and X. Chen, Chem. Rev., 2016, 116, 3086–3240 CrossRef CAS PubMed;
(b) Y. Zheng, S. Li, Z. Weng and C. Gao, Chem. Soc. Rev., 2015, 44, 4091–4130 RSC.
|
This journal is © The Royal Society of Chemistry 2017 |
Click here to see how this site uses Cookies. View our privacy policy here.