DOI:
10.1039/C5RA26849D
(Paper)
RSC Adv., 2016,
6, 17811-17817
A turn on fluorescent sensor based on lanthanide coordination polymer nanoparticles for the detection of mercury(II) in biological fluids†
Received
15th December 2015
, Accepted 27th January 2016
First published on 1st February 2016
Abstract
Coordination polymers have recently emerged as very fascinating materials due to their tunable nature. In this work, we develop a lanthanide coordination polymer (CPNP)-based turn on sensor for Hg2+ detection by employing the strategy of inner filter effect (IFE). This kind of CPNP is composed of europium ions (Eu3+) as metal centers and isophthalic acid (IPA) as bridging ligands that can sensitize the fluorescence of Eu3+. Because the excitation spectrum of Eu/IPA CPNPs greatly overlaps with the absorbance band of imidazole-4,5-dicarboxylic acid (Im), the presence of Im can significantly quench the fluorescence of Eu/IPA CPNPs through a process of IFE. Upon the addition of Hg2+, however, the Im-quenched fluorescence of Eu/IPA CPNPs can be recovered due to the suppression of the IFE of Im through the formation of a Hg/Im complex. As a fluorescent sensor for Hg2+ detection, Eu/IPA CPNPs not only show high sensitivity up to a detection limit of 2 nM and excellent selectivity, but also possess the advantages of fast response, simple preparation procedure and flexible sensing performance. More importantly, interference from the background fluorescence of biological fluids can be efficiently eliminated via a time-resolved detection mode. The presence of the sensing strategy would be beneficial to the design of other lanthanide coordination polymer-based fluorescent sensors.
Introduction
Mercury(II) ion is one of the most toxic pollutants, which is widely distributed in the air, water and soil through natural and human activities. Mercury(II) can accumulate in the human body through the food chain, leading to brain damage and other chronic diseases. So, sensitive detection of mercury(II) ions is a very important task to ensure human health. In the past few decades, many methods have been developed to detect Hg2+, such as atomic absorption spectrometry,1,2 inductively coupled plasma atomic emission spectroscopy (ICP-AES),3 liquid chromatography4 and so on. Among these methods, fluorescence detection would be a more desirable method because of its simplicity, high sensitivity and rapid response time. To date, various fluorescent sensors based on organic dyes and inorganic nanocrystals have been reported for the detection of Hg2+.5–7 These fluorescent sensors have made great contributions toward an Hg2+ assay, but the drawbacks of poor water solubility, complicated synthesis procedures, toxic agents, and high cost limit their further applications, especially in biological samples. Moreover, owing to the quenching nature of Hg2+, these fluorescent sensors mostly work in turn off mode that often shows less sensitivity and selectivity compared with turn on sensors.8–10 Therefore, it is highly desirable to develop a turn on fluorescent sensor for the detection of Hg2+ in biological samples.
In recent years, coordination polymers have emerged as a very fascinating material due to their adjustable properties and potential applications in gas storage, catalysis, separation, drug delivery, and chemical sensing.11 Particularly, lanthanide coordination polymers have received considerable attention because of their unique optical features including large Stokes shifts, high quantum yields, and long lifetimes.12,13 Moreover, compared with molecular lanthanide compounds or inorganic nanocrystals, lanthanide coordination polymers can offer the advantages of superior mechanical properties, better processability, and thermal stability.14 Such properties make them ideal for the construction of optical devices and tunable fluorescent sensors as well as probes for biological and chemical species. In fact, some lanthanide coordination polymers have recently been successfully employed for the sensing of metal ions,15,16 anions17,18 and small molecules.19,20 In spite of this, these sensing reactions are mainly conducted in organic solution and usually lack sufficient chemical selectivity.20,21 Thus, great challenges still remain for the rational design and development of lanthanide coordination polymer-based fluorescent sensors with high selectivity.
Inspired by the above-mentioned facts, here we attempt to develop a lanthanide coordination polymer-based turn on sensor by employing the strategy of inner filter effect (IFE). The IFE refers to the absorption of the excitation and/or emission light of a fluorophore by an absorber in the detection system, which has been considered as an efficient strategy in developing fluorescent sensors.22 Unlike fluorescent sensors based on the strategies of fluorescence resonance energy transfer, intramolecular charge transfer and photoinduced electron transfer, IFE does not require a complicated chemical linkage between the absorber and fluorophore for signal communication. This feature will endow IFE-based fluorescent sensors with considerable flexibility and simplicity.23,24 Nevertheless, to the best of our knowledge, there have been no reports to date on the exploration of lanthanide coordination polymers in the construction of IFE-based turn on sensors.
In this work, an IFE-based turn on sensor was constructed through selecting europium (Eu3+)-based coordination polymer nanoparticles (CPNPs) as the fluorophore and imidazole-4,5-dicarboxylic acid (Im) as the absorber. The CPNPs were built from the self-assembly of Eu3+ and isophthalic acid (IPA), denoted as Eu/IPA CPNPs. Because of the sensitizing effect of IPA, Eu/IPA CPNPs can emit strong red fluorescence.25 Upon the addition of Im, however, a significant decrease in the fluorescence of Eu/IPA CPNPs was observed. This is due to Im having a strong absorption band that greatly overlaps with the excitation spectrum of Eu/IPA CPNPs, which can filter out the excitation energy of Eu/IPA CPNPs through a process of IFE. On the other hand, it has been reported that Im can react with Hg2+ to form a stable Hg/Im complex due to its high affinity to Hg2+.26 Based on this fact, when Hg2+ was further added to the above system, Im could be released from the surface of Eu/IPA CPNPs to form a Hg/Im complex, leading to the suppression of the IFE of Im. In the presence of Hg2+, therefore, a recovered fluorescence of Eu/IPA CPNPs through the formation of a Hg/Im complex would be observed (Scheme 1).
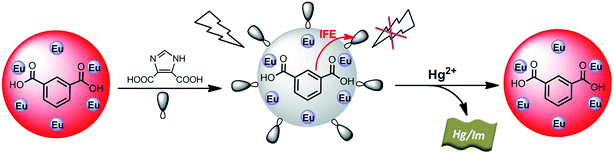 |
| Scheme 1 Schematic illustration of the changes in the fluorescence of Eu/IPA CPNPs induced by Im and Hg2+. | |
Experimental section
Chemicals and solutions
Europium nitrate hexahydrate (Eu(NO3)3·6H2O, 99.99%) were purchased from Ruike Rare Earth Metallurgy and Functional Materials Co., Ltd. (Baotou, China). Metal salts (HgCl2, AgNO3, MgCl2, KCl, NaCl, Pb(NO3)2, Zn(NO3)2, Ce(NO3)3, CdCl2, FeCl3, CaCl2, NiCl2, CoCl2, MnCl2, CrCl3 and AlCl3), amino acids (tryptophan (Trp), aspartic acid (Asp), alanine (Ala), leucine (Leu), glutamic acid (Glu), cysteine (Cys), phenylalanine (Phe) and methionine (Mel)), glucose, and ascorbic acid were purchased from Sinopharm Chemical Reagent Company (Shanghai, China). Imidazole-4,5-dicarboxylic acid (Im) and isophthalic acid (IPA) were obtained from Aladdin Chemical Company (Shanghai, China). Distilled and ultrapure water (18 MΩ cm−1) was used for the preparation of all aqueous solutions. Unless otherwise stated, all chemicals are of analytical reagent grade and used as received.
Instruments
The morphology of Eu/IPA CPNPs was examined using S-3400 scanning electron microscopy (SEM, Hitachi, Japan) equipped with an energy dispersive spectroscopy (EDS) detector and transmission electron microscopy (TEM, JEM-2100, Japan). The fluorescence spectra of Eu/IPA CPNPs were recorded by using a PerkinElmer LS55 spectrofluorometer (PerkinElmer, UK). A Lambda 35 spectrophotometer (PerkinElmer, UK) was employed to record the UV-visible absorption spectra. An Avatar 360 FTIR spectrometer (Nicolet, USA) was used to record the Fourier transform infrared (FTIR) spectra with the KBr pellet technique. Analysis of the powder X-ray diffraction (XRD) spectrum was performed on a D8 Advance X-ray diffractometer (Bruker, Germany).
Preparation of Eu/IPA CPNPs
The Eu/IPA CPNPs were synthesized by using a solvothermal method. Typically, 2 mL of IPA aqueous solution (40 mM) was first added to 8 mL of N,N-dimethylformamide (DMF) and mixed well. Then, 2 mL of Eu(NO3)3 aqueous solution was added dropwise to the above mixture. After stirring for 30 min at room temperature, the mixture containing Eu(NO3)3 and IPA was transferred to a Teflon-lined stainless steel vessel (50 mL) and reacted for 8 h at 170 °C. The white products were collected through centrifugation (13
000 rpm, 10 min) after cooling to room temperature. After washing three times with absolute ethanol, the as-obtained precipitate (approximately 0.0106 g, dry) was finally dispersed in 4 mL of absolute ethanol to form a Eu/IPA CPNPs suspension.
Quenching effect of Im on the fluorescence of Eu/IPA CPNPs
The quenching effect of Im to the fluorescence of Eu/IPA CPNPs was studied at room temperature. Briefly, 1 μL of Eu/IPA CPNPs (2.65 mg mL−1) was first added to Tris–HCl buffer (25 mM, pH 7.0) and dispersed well using ultrasonic to obtain a Eu/IPA CPNPs suspension. Then, Im solutions with final concentrations from 0 to 1 mM were added to the above solution. The final reaction volumes were kept at 100 μL. The final concentration of Eu/IPA CPNPs was 26.5 μg mL−1. After reacting for 10 min, the fluorescence spectra of these reaction solutions were recorded.
Detection of Hg2+ based on the combination of Eu/IPA CPNPs with Im
To detect Hg2+ in aqueous solution, 3.2 μL of the Im solution (10 mM) was first reacted with 1 μL of the Eu/IPA CPNPs suspension in 50 μL of Tris–HCl buffer for 10 min. Then, Hg2+ aqueous solutions with final concentrations from 0 to 100 μM were added to the above solution, and Tris–HCl buffer was used to maintain the final reaction volume at 100 μL. After the reaction was carried out for 2 min at room temperature, the fluorescence spectra of these reaction solutions were measured. To evaluate the selectivity of Eu/IPA CPNPs to Hg2+, the Eu/IPA-Im complex was first prepared through the reaction of 3.2 μL of the Im solution (10 mM) with 1 μL of the Eu/IPA CPNPs suspension in 50 μL of Tris–HCl buffer at room temperature. Then, 1 μL volumes of 10 mM stock solutions of interference metal ions were individually added to the above solution. The total volume was increased to 100 μL by adding Tris–HCl buffer. The reaction was allowed to last 5 min before measuring the fluorescence intensities of these mixtures at 615 nm.
Determination of Hg2+ in biological fluids
The levels of Hg2+ in biological fluids (urine samples) were determined by using the standard addition method. The urine samples were obtained from healthy adult volunteers. All experiments were performed in compliance with relevant laws and institutional guidelines and with the approval of the Institutional Ethics Committee of Jiangxi Normal University, and informed consent was obtained from all participants. The original urine samples were diluted 10-fold for pretreatment. The spiked urine samples were prepared by adding a Hg2+ standard solution to diluted urine samples with final concentrations from 0 to 1 μM. The spiked urine samples were mixtures containing 3.2 μL of Im solution (10 mM) and 1 μL of the Eu/IPA CPNPs suspension, which were allowed to react for 2 min at room temperature and then had their fluorescence spectra recorded with an excitation wavelength of 250 nm.
Results and discussion
Characterization of Eu/IPA CPNPs
The SEM image of Eu/IPA CPNPs shows that the as-prepared Eu/IPA CPNPs are spherical structures (Fig. 1a). The average size of the Eu/IPA CPNPs is estimated to be approximately 405 ± 40 nm. The chemical composition of the Eu/IPA CPNPs was analyzed using EDS. As shown in Fig. 1b, the EDS peaks corresponding to C, O and Eu can be observed. The element ratios (atom %) of C, O and Eu were found to be 57.26, 38.12 and 4.62 %, respectively. The results reveal that IPA and Eu3+ are both involved in the formation of Eu/IPA CPNPs. The HRTEM image (Fig. S1†) indicates that no europium oxides were presented in the Eu/IPA CPNPs. To discern the chemical interaction of Eu3+ and IPA in Eu/IPA CPNPs, FTIR spectra of pure IPA and Eu/IPA CPNPs were analyzed. From Fig. 1c, it can be seen that the COO− stretching vibration of IPA was shifted from 1685 to 1604 cm−1 after the formation of Eu/IPA CPNPs.27 This indicates that the formation of Eu/IPA CPNPs is a result of the chemical coordination of Eu3+ with the carboxylate groups of IPA. Moreover, the XRD pattern of the Eu/IPA CPNPs reflects that they are structurally amorphous and not crystalline (Fig. 1d). So, the as-prepared Eu/IPA CPNPs are infinite coordination polymers (ICPs) rather than metal–organic frameworks (MOFs) with a crystalline structure,28 which is consistent with previously reported lanthanide CPNPs.29–31
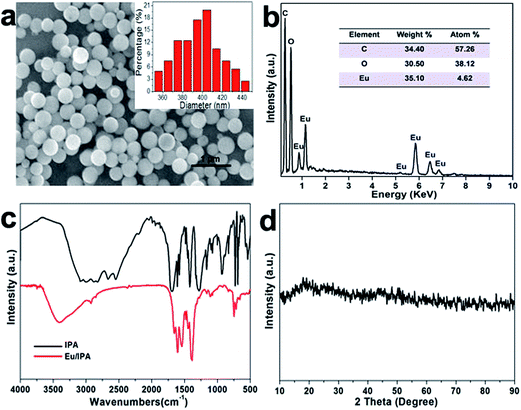 |
| Fig. 1 SEM image (a), EDS (b), FTIR spectrum (c) and XRD parttern (d) of Eu/IPA CPNPs. Inset: size distribution of Eu/IPA CPNPs. | |
Quenching effect of Im on the fluorescence of Eu/IPA CPNPs
Under an excitation wavelength of 250 nm, the Eu/IPA CPNPs exhibit a strong red fluorescence with four emission peaks at 593, 615, 648 and 698 nm (Fig. 2a). These emission peaks can be assigned to the Eu3+ electronic transitions of 5D0 to 7FJ (J = 1, 2, 3, 4).25 The results indicate that IPA can efficiently transfer its absorbed energy to Eu3+, sensitizing the Eu3+ fluorescence through an antenna effect. Upon the addition of Im, however, an obvious decrease in the fluorescence of Eu/IPA CPNPs was observed. Such quenching behavior can even be observed by the naked eye under a common UV lamp (Fig. 2b). Fig. 3a shows that Im has an absorption band in the range of 225 to 280 nm, which greatly overlaps the excitation spectrum of the Eu/IPA CPNPs from 220 to 320 nm. In particular, the strongest absorption peak of Im at 248 nm is very close to the maximum excitation wavelength of Eu/IPA CPNPs at 250 nm. Based on this finding, the presence of Im could filter out the excitation energy of the Eu/IPA CPNPs through a well known process of IFE, leading to the quenching of the fluorescence of the Eu/IPA CPNPs.32 Meanwhile, no spectral overlapping between the emission band of the Eu/IPA CPNPs and the absorption band of Im was observed, implying that the possibility of a fluorescence resonance energy transfer process from Eu/IPA CPNPs to Im can be excluded.33 The fluorescence behavior of Eu/IPA CPNPs in the presence of Im in the reaction media with different pH values (Fig. S2†) suggests that a photoinduced electron transfer process between Eu/IPA CPNPs and Im does not occur.34,35 Therefore, the Im-induced fluorescence quenching of Eu/IPA CPNPs originates from the IFE of Im.
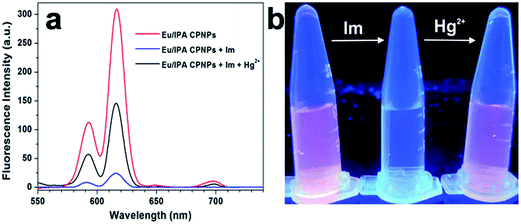 |
| Fig. 2 (a) Emission spectra of 26.5 μg mL−1 of Eu/IPA CPNPs in the absence and presence of 320 μM of Im with and without 100 μM Hg2+. The excitation wavelength is 250 nm. (b) The corresponding photograph of these three samples under a 365 nm UV lamp. | |
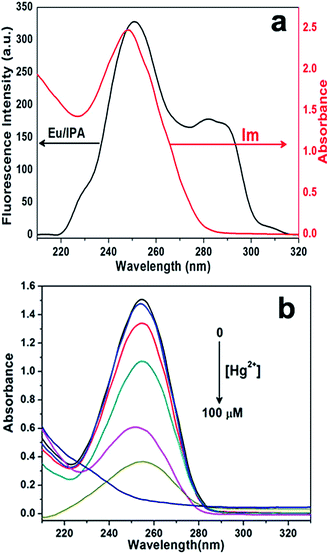 |
| Fig. 3 (a) Excitation spectrum of 26.5 μg mL−1 of Eu/IPA CPNPs (black line) and the absorption spectrum of 320 μM of pure Im (red line). The emission wavelength was fixed at 615 nm to record the excitation spectrum of Eu/IPA CPNPs. (b) Absorption spectra of the Eu/IPA-Im complex in the presence of Hg2+ with different concentrations (0, 0.5, 5, 10, 30, 50 and 100 μM). | |
As shown in Fig. S3,† there was an obvious decrease in the fluorescence of Eu/IPA CPNPs after the addition of 2 μM of Im. The fluorescence intensity of Eu/IPA CPNPs decreased with an increase in Im concentration, it was quenched more than 90% when the Im concentration reached 320 μM and was nearly quenched completely at more than 400 μM of Im. Although higher concentrations of Im can result in more complete fluorescence quenching, increased amounts of Hg2+ are required to bind Im for recovering the fluorescence of Eu/IPA CPNPs. Therefore, an Im concentration of 320 μM was used in the present study to obtain the best sensitivity.
Due to the existence of carboxylate groups, the added Im would be attached on the surface of Eu/IPA CPNPs by coordinating with Eu3+ to form a Eu/IPA-Im complex rather than being present free in the Eu/IPA CPNPs suspension. This is evidenced by the shifted FTIR spectrum of Im (Fig. S4†) and enhanced emission lifetime of the Eu/IPA-Im complex (Fig. S5†). In spite of this, the binding of Im with Eu/IPA CPNPs has almost no effect on the structural properties including the morphology, size and XRD pattern of the Eu/IPA CPNPs (Fig. S6†).
Recovery of the Im-quenched fluorescence of Eu/IPA CPNPs induced by Hg2+
When Hg2+ was further added to the Eu/IPA-Im complex suspension, the fluorescence of the Eu/IPA CPNPs can be observed again. This implies that Hg2+ is able to recover the Im-quenched fluorescence of the Eu/IPA CPNPs. Nevertheless, the presence of Hg2+ showed a very slight influence (<5%) on the fluorescence of the Eu/IPA CPNPs alone (Fig. S7†). So, we suppose that the Hg2+-recovered fluorescence of the Eu/IPA-Im complex might only arise from the interaction of Hg2+ with the Im on the surface of the Eu/IPA-Im complex. To confirm this hypothesis, the absorption spectra of the Eu/IPA-Im complex in the presence of Hg2+ were firstly measured. As shown in Fig. 3b, in the presence of Hg2+, an obvious decrease in the absorbance of Im in the range of 225 to 280 nm can be observed. With increased Hg2+ concentration, the absorbance of Im decreased gradually and finally completely disappeared when the Hg2+ concentration reached 100 μM. For Eu/IPA CPNPs, the decreased Im absorbance will allow more light to be available to produce fluorescence. Thus, the Im-quenched fluorescence of Eu/IPA CPNPs would be recovered upon the addition of Hg2+.
By examining the morphology of the reaction products of the Eu/IPA-Im complex with Hg2+ we found that some bulk structures were present in the reaction products in addition to the spherical Eu/IPA CPNPs (Fig. S8a†). Since the addition of pure Im or Hg2+ has almost no influence on the morphology of the Eu/IPA CPNPs alone (Fig. S6 and S9†), the bulk structures could be Hg/Im complexes that were formed from the reaction of Hg2+ with Im on the surface of the Eu/IPA CPNPs. Furthermore, obvious diffraction peaks from the products can be observed in the XRD pattern at 2θ = 16, 17, 18, 29 and 31° (Fig. S8b†). These peaks are new compared with the Eu/IPA CPNPs alone, but they are consistent with the XRD pattern of a pure Hg/Im complex. Apparently, the bulk structures in the reaction products of the Eu/IPA-Im complex with Hg2+ are Hg/Im complexes. Therefore, these results further support that the Hg2+-recovered fluorescence of Eu/IPA CPNPs results from the suppression of the IFE between Im and Eu/IPA CPNPs through the formation of a Hg/Im complex. It was noteworthy that the reaction of Hg2+ with the Eu/IPA-Im complex does not induce changes in the structure of the Eu/IPA CPNPs. This is different from previously reported coordination polymer-based fluorescent sensors.36,37
Detection sensitivity
The fluorescence response rate of the Eu/IPA-Im complex to Hg2+ is fast. After the addition of Hg2+, the fluorescence of the Eu/IPA-Im complex rapidly increased, and reached a constant value within 2 min (Fig. S10†). In addition, the maximum fluorescence of the Eu/IPA-Im complex in the presence of Hg2+ was observed when this reaction was performed in a solution of pH 7.0 (Fig. S11†). Thus, the reaction time of 2 min and a reaction media with pH 7.0 were used in the subsequent experiments to obtain the best sensitivity. Under the optimized conditions, the fluorescence changes of the Eu/IPA-Im complex in the presence of Hg2+ with different concentrations were then investigated. As shown in Fig. 4, the fluorescence of the Eu/IPA-Im complex was enhanced with gradually increasing Hg2+ concentration. The fluorescence intensity of the Eu/IPA-Im complex at 615 nm displayed a linear correlation to the Hg2+ concentration from 2 nM to 2 μM. The detection limit for Hg2+ is estimated to be about 2 nM (signal/noise > 3), which is lower than the maximum allowable Hg2+ level in drinking water (10 nM) permitted by the U.S. Environmental Protection Agency (EPA).38 Compared with most of the previous fluorescence methods for Hg2+ detection,7,39–42 the presented method not only showed a comparable detection limit and simple preparation of the sensing material, but also can efficiently eliminate the interference of background fluorescence via a time-resolved detection mode. Moreover, the proposed Eu/IPA CPNPs-based fluorescent sensor has the advantages of a turn on work mode with high sensitivity and selectivity and a faster response over other coordination polymer-based fluorescent sensors.
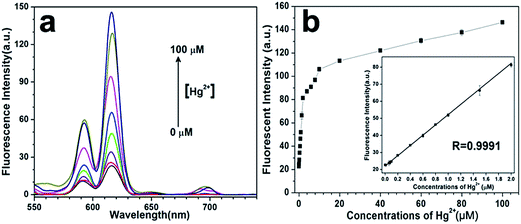 |
| Fig. 4 Emission spectra (a) and fluorescence intensities at 615 nm (b) of the Eu/IPA-Im complex in the presence of Hg2+. The reactions were performed in Tris–HCl buffer (25 mM, pH 7.0) with 26.5 μg mL−1 of Eu/IPA CPNPs, 320 μM of Im and Hg2+ with concentrations from 0 to 100 μM at room temperature, the total volume was100 μL. Inset: plot of the fluorescence intensity of the Eu/IPA-Im complex at 615 nm as a function of the Hg2+ concentration. The excitation wavelength is 250 nm. | |
Selectivity of the Eu/IPA-Im complex to Hg2+
To examine whether other metal ions can switch on the fluorescence of the Eu/IPA-Im complex, the fluorescence responses of the Eu/IPA-Im complex to various metal ions, including Ag+, K+, Na+, Mg2+, Pb2+, Zn2+, Cd2+, Ca2+, Ni2+, Co2+, Mn2+, Ce3+, Fe3+, Cr3+ and Al3+, were investigated under identical conditions. As shown in Fig. 5, only the addition of Hg2+ results in a significant enhancement in the fluorescence of the Eu/IPA-Im complex, and no marked fluorescence responses were found in the presence of other metal ions. Even though the concentrations of the other metal ions reached 1 mM, the changes in the fluorescence of the Eu/IPA-Im complex were still negligible. The results indicate that the fluorescence response of the Eu/IPA-Im complex to Hg2+ is highly specific. It is known that Im is a heterocyclic compound, which can coordinate with Hg2+ through its N atom of the imidazole ring and the O atom of the carboxylate groups. The binding constant of imidazole with Hg2+ is much higher than that of other metal ions, even including Eu3+.26,43,44 In addition, the presence of carboxylate groups has been reported to be able to increase the stability of imidazole complexes with metal ions.26 Therefore, the excellent selectivity of the Eu/IPA-Im complex to Hg2+ could be attributed to the higher affinity of Im to Hg2+ than other metal ions.
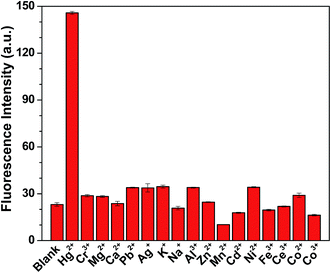 |
| Fig. 5 Fluorescence responses of the Eu/IPA-Im complex to different metal ions (each 100 μM). The reactions were performed in Tris–HCl buffer (25 mM, pH 7.0) with 26.5 μg mL−1 of the Eu/IPA CPNPs, 320 μM of Im and 100 μM of Hg2+ or other metal ions at room temperature, the total volume was 100 μL. The excitation wavelength is 250 nm. | |
Next, we investigated the effects of interfering substances that may coexist in urine on the fluorescence of the Eu/IPA-Im complex. Various amino acids, glucose and ascorbic acid were employed as interfering substances. As shown in Fig. S12,† only very little changes in the fluorescence of the Eu/IPA-Im complex were observed upon the addition of the above interfering substances (black bars). However, after the addition of Hg2+ to the Eu/IPA-Im complex solutions containing these interfering substances, the fluorescence of the Eu/IPA-Im complex increased immediately (gray bars). Compared with that in the presence of only Hg2+, no significant changes in the fluorescence of the Eu/IPA-Im complex were observed from the coexistence of these interfering substances and Hg2+. The results indicate that the Eu/IPA-Im complex showed high selectivity to Hg2+ against not only metal ions but also interfering substances. The negligible interference from these interfering substances suggests the potential utility of the Eu/IPA-Im complex as a fluorescent sensor for the detection of Hg2+ in biological samples.
Determination of Hg2+ in biological fluids
The levels of Hg2+ in urine are usually used as a indicator of recent exposure to mercury because urinary mercury is closest to the level of Hg2+ presented in the kidneys.45 Thus, the levels of Hg2+ in urine samples were determined to test the practical application of the Eu/IPA-Im complex as a turn on fluorescent sensor. From Table S1,† it can be seen that the recoveries of Hg2+ in urine samples are all more than 95% and the relative standard deviations (RSD, n = 3) are all less than 3.81%. The results indicate that spiked Hg2+ could be accurately measured with good recovery and precision. Therefore, the presented sensor can be used for the detection of Hg2+ in urine samples.
Conclusions
In summary, we have developed a turn on fluorescence sensor based on Eu/IPA CPNPs for the detection of Hg2+ by employing the strategy of IFE. The presence of Im can quench the fluorescence of Eu/IPA CPNPs through a process of IFE, whereas recovered fluorescence of Eu/IPA CPNPs can be observed upon the further addition of Hg2+ through the formation of a Hg/Im complex. As a turn on fluorescent sensor for Hg2+, the Eu/IPA CPNPs not only show high sensitivity up to a detection limit of 2 nM and excellent selectivity, but they also possess the features of a simple preparation procedure and flexible sensing performance. Different from previously reported coordination polymer-based fluorescent sensors, the Eu/IPA CPNP-based sensing reaction does not induce changes in the structure of the Eu/IPA CPNPs, and thus displays a fast response rate to targets. Furthermore, the proposed turn on fluorescent sensor was applied successfully in the determination of Hg2+ in biological fluids and showed satisfactory results. We believe that the proposed sensing strategy would provide a new insight into the design of other lanthanide coordination polymer-based fluorescent sensors, expanding their applications in biomedical fields.
Acknowledgements
This work was supported by the Natural Science Foundation of China (No. 21305054), Start-up Funding of Jiangxi Normal University, Specialized Research Funding for the Doctoral Program of Higher Education of China (No. 20133604120002), Scientific Research Foundation of Education Commission of Jiangxi Province (No. GJJ14258), and the Natural Science Foundation of Jiangxi Province (No. 20151BAB203020).
References
- M. Filippelli, Anal. Chem., 1987, 59, 116–118 CrossRef CAS PubMed.
- T. Labatzke and G. Schlemmer, Anal. Bioanal. Chem., 2004, 378, 1075–1082 CrossRef CAS PubMed.
- M. Wang, W. Feng, J. Shi, F. Zhang, B. Wang, M. Zhu, B. Li, Y. Zhao and Z. Chai, Talanta, 2007, 71, 2034–2039 CrossRef CAS PubMed.
- H. Chen, J. Chen, X. Jin and D. Wei, J. Hazard. Mater., 2009, 172, 1282–1287 CrossRef CAS PubMed.
- S. Yoon, E. W. Miller, Q. He, P. H. Do and C. J. Chang, Angew. Chem., Int. Ed., 2007, 46, 6658–6661 CrossRef CAS PubMed.
- S. Voutsadaki, G. K. Tsikalas, E. Klontzas, G. E. Froudakis and H. E. Katerinopoulos, Chem. Commun., 2010, 46, 3292–3294 RSC.
- J. Duan, L. Song and J. Zhan, Nano Res., 2009, 2, 61–68 CrossRef CAS.
- E. M. Nolan and S. J. Lippard, Chem. Rev., 2008, 108, 3443–3480 CrossRef CAS PubMed.
- L. Shang, L. Zhang and S. Dong, Analyst, 2009, 134, 107–113 RSC.
- S. Hussain, S. De and P. K. Iyer, ACS Appl. Mater. Interfaces, 2013, 5, 2234–2240 CAS.
- A. Carne, C. Carbonell, I. Imaz and D. Maspoch, Chem. Soc. Rev., 2011, 40, 291–305 RSC.
- J. Rocha, L. D. Carlos, F. A. A. Paz and D. Ananias, Chem. Soc. Rev., 2011, 40, 926–940 RSC.
- Y. Cui, B. Chen and G. Qian, Coord. Chem. Rev., 2014, 273–274, 76–86 CrossRef CAS.
- Y. Qiao, H. Chen, Y. Lin, Z. Yang, X. Cheng and J. Huang, J. Phys. Chem. C, 2011, 115, 7323–7330 CAS.
- W. Liu, T. Jiao, Y. Li, Q. Liu, M. Tan, H. Wang and L. Wang, J. Am. Chem. Soc., 2004, 126, 2280–2281 CrossRef CAS PubMed.
- W. Yang, J. Feng and H. Zhang, J. Mater. Chem., 2012, 22, 6819–6823 RSC.
- K. L. Wong, G. L. Law, Y. Y. Yang and W. T. Wong, Adv. Mater., 2006, 18, 1051–1054 CrossRef CAS.
- B. Chen, L. Wang, F. Zapata, G. Qian and E. B. Lobkovsky, J. Am. Chem. Soc., 2008, 130, 6718–6719 CrossRef CAS PubMed.
- B. Chen, Y. Yang, F. Zapata, G. Lin, G. Qian and E. B. Lobkovsky, Adv. Mater., 2007, 19, 1693–1696 CrossRef CAS.
- H. Xu, F. Liu, Y. Cui, B. Chen and G. Qian, Chem. Commun., 2011, 47, 3153–3155 RSC.
- H. Xu, X. Rao, J. Gao, J. Yu, Z. Wang, Z. Dou, Y. Cui, Y. Yang, B. Chen and G. Qian, Chem. Commun., 2012, 48, 7377–7379 RSC.
- X. Yan, H. Li, Y. Li and X. Su, Anal. Chim. Acta, 2014, 852, 189–195 CrossRef CAS PubMed.
- S. Yang, C. Wang, C. Liu, Y. Wang, Y. Xiao, J. Li, Y. Li and R. Yang, Anal. Chem., 2014, 86, 7931–7938 CrossRef CAS PubMed.
- Y. Tang, Y. Liu and A. Cao, Anal. Chem., 2013, 85, 825–830 CrossRef CAS PubMed.
- W. Cho, H. J. Lee, S. Choi, Y. Kim and M. Oh, Sci. Rep., 2014, 4, 6518 CrossRef CAS PubMed.
- R. J. Sundberg and R. B. Martin, Chem. Rev., 1974, 74, 471–517 CrossRef CAS.
- J. Son, H. J. Lee and M. Oh, Chem.–Eur. J., 2013, 19, 6546–6550 CrossRef CAS PubMed.
- W. Lin, W. J. Rieter and K. M. L. Taylor, Angew. Chem., Int. Ed., 2009, 48, 650–658 CrossRef CAS PubMed.
- H. Tan, L. Zhang, C. Ma, Y. Song, F. Xu, S. Chen and L. Wang, ACS Appl. Mater. Interfaces, 2013, 5, 11791–11796 CAS.
- M. Yang, Z. Shen, T. Chen, H. Bi, B. Yang and W. Xu, Dalton Trans., 2013, 42, 1174–1179 RSC.
- Z. Shen, G. Zhang, H. Zhou, P. Sun, B. Li, D. Ding and T. Chen, Adv. Mater., 2008, 20, 984–988 CrossRef CAS.
- Y. Wang, L. Xiong, F. Geng, F. Zhang and M. Xu, Analyst, 2011, 136, 4809–4814 RSC.
- C. Yu, Y. Wu, F. Zeng and S. Wu, J. Mater. Chem. B, 2013, 1, 4152–4159 RSC.
- G. Zong and G. Lu, J. Phys. Chem. C, 2009, 113, 2541–2546 CAS.
- X. Guo, X. Qian and L. Jia, J. Am. Chem. Soc., 2004, 126, 2272–2273 CrossRef CAS PubMed.
- J. Deng, P. Yu, Y. Wang and L. Mao, Anal. Chem., 2015, 87, 3080–3086 CrossRef CAS PubMed.
- N. Lin, J. Li, Z. Lu, L. Bian, L. Zheng, Q. Cao and Z. Ding, Nanoscale, 2015, 7, 4971–4977 RSC.
- U. S. EPA, Drinking Water Criteria Document for Inorganic Mercury, Environmental Criteria and Assessment Office, Cincinnati, Ohio, 1988.
- L. Zhou, Y. Lin, Z. Huang, J. Ren and X. Qu, Chem. Commun., 2012, 48, 1147–1149 RSC.
- B. Liu, F. Zeng, Y. Liu and S. Wu, Analyst, 2012, 137, 1698–1705 RSC.
- H. Li and L. Wang, Analyst, 2013, 138, 1589–1595 RSC.
- J. M. Fang, P. F. Gao, X. L. Hu and Y. F. Li, RSC Adv., 2014, 4, 37349–37352 RSC.
- P. Brooks and N. Davidson, J. Am. Chem. Soc., 1960, 82, 2118–2123 CrossRef CAS.
- J. M. Thomas, R. Ting and D. M. Perrin, Org. Biomol. Chem., 2004, 2, 307–312 CAS.
- T. W. Clarkson, L. Friberg, G. F. Nordberg and P. R. Sager, Biological monitoring of toxic metals, Plenum Press, New York, NY, 1988 Search PubMed.
Footnote |
† Electronic supplementary information (ESI) available. See DOI: 10.1039/c5ra26849d |
|
This journal is © The Royal Society of Chemistry 2016 |