DOI:
10.1039/C6RA04247C
(Paper)
RSC Adv., 2016,
6, 48479-48488
Fabrication of monodispersed Au@Ag bimetallic nanorod-loaded nanofibrous membrane with fast thermo-responsiveness and its use as a smart free-standing SERS substrate†
Received
16th February 2016
, Accepted 1st May 2016
First published on 3rd May 2016
Abstract
A novel type of metal nanoparticle-loaded smart nanofibrous membrane with fast thermo-responsiveness was fabricated by electrospinning an aqueous solution containing poly(N-isopropylacrylamide-co-N-hydroxymethylacrylamide) and monodispersed Au@Ag bimetallic nanorods with a core–shell structure, followed by heat treatment. The results obtained by electron microscopy show that the anisotropic nanoparticles are oriented along the axes of its constituent nanofibers. The membrane produced has high stability in aqueous media and remarkable thermo-responsiveness. It takes less than 10 seconds to reach its deswelling or swelling equilibrium state with the temperature alternating between 25 °C and 50 °C. The smart nanofibrous membrane with macroscopic mechanical strength can be used as a free-standing surface-enhanced Raman scattering (SERS) substrate with high Raman signal reproducibility for quantitative analysis, and its SERS efficiency can be readily elevated by raising the detection temperature across its phase transition temperature due to its fast thermo-response rate. Particularly, since the composite nanofibrous membrane possesses catalytic properties toward the reduction of 4-nitrothiophenol to 4-aminothiophenol by NaBH4, it has the ability to act as an in situ SERS monitor for the reaction, and it was deduced from the detected intermediate that the reaction proceeds via a condensation reaction route.
Introduction
In recent years, metal nanoparticle-loaded stimulus-responsive composite materials have attracted more and more attention due to their prominent characteristics, i.e., the properties of the loaded nanoparticles can be tuned by an external stimulus or the stimulus can be sensed by detecting their change in properties.1–4 Therefore, smart composite materials have fascinating potential applications in many fields, such as sensors, actuators, biomedicine, and catalysis.1,4–6 To date, the smart polymer materials used for loading metal nanoparticles have been stimulus-responsive bulky hydrogels and microgels (including nanogels) with nanometer to micrometer sizes.4,5,7 It is known that smart bulky hydrogels have much slower stimulus responses than smart microgels due to their response rates being inversely correlated to their size.8 Since a fast stimulus-response in smart materials is important to their applications, such as tunable catalysis, sensing or detection, actuation, and drug delivery,1,9 smart microgels are preferentially used for loading metal nanoparticles.4,5,10,11 For example, in our group, pH and/or temperature-responsive microgels have been utilized for loading Ag or Au nanoparticles, and the composite microgels produced were used as smart catalysts or surface-enhanced Raman scattering (SERS) substrates with temperature-controllable activity.12–15 However, smart microgels usually exist as colloidal particles in aqueous solution, so they suffer from poor colloidal stability and no macroscopic morphology or mechanical strength for use in devices such as sensors or actuators. Moreover, when used as catalysts, they are difficult to recycle. Hence, developing a new type of smart polymer material for loading metal nanoparticles, which has both macroscopic mechanical strength and a fast stimulus-response, is of important practical significance.
A stimulus-responsive nanofibrous membrane is a smart polymer material, which consists of orderly or randomly aligned nanofibers with diameters of tens to hundreds of nanometers.16 Compared to smart bulky hydrogels, these have much faster stimulus-responses owing to their constituent ultra thin fibers and porous structure, which is helpful for the transfer of the stimulus to the whole material. Their reported applications have demonstrated that such smart materials allow for convenient use in a device because of their macroscopic mechanical strength and flexible morphology.17,18 If used as a carrier for nanocatalysts, they can be readily recovered from a reaction system.19,20 A nanofibrous membrane is usually produced by an electrospinning technique, which features simplicity, flexible morphology tuning and a wide variety of usable polymers.19,21,22 Therefore, it could be expected that stimulus-responsive electrospun nanofibrous membranes can act as substitutes for smart bulky hydrogels and microgels for loading metal nanoparticles.
Within the field of metal nanoparticle-based nanoscience and nanotechnology, bimetallic nanoparticles with a core–shell structure have attracted increasing interest due to their superior physicochemical, optical and catalytic properties compared to their respective monometallic analogues.23,24 Among these, a bimetallic nanorod based on a gold nanorod (AuNR) core and a silver shell (Au@AgNR) is particularly attractive because of its potential applications in surface-enhanced Raman scattering (SERS) substrates and catalysts.23,25,26 Recently, in our group, monodispersed Au@AgNR prepared through a seed-mediated growth process by reduction of AgNO3, using AuNRs with a narrow size and shape distribution as seeds, was used as a SERS substrate with high Raman signal reproducibility for quantitative analysis.26 Its SERS efficiency is remarkably increased with its Ag shell thickness due to the enhancing surface electric field and the chemical enhancement associated with the electronic ligand effect. Furthermore, it has been reported that, compared to monometallic Ag nanoparticles, the bimetallic Au@Ag nanoparticles with a core–shell structure have much higher chemical stability owing to free electron transfer from the Au core to the Ag shell.27 This can avoid weakening of the Au@AgNRs'; SERS efficiency during usage and storage, otherwise their oxidized Ag surfaces would hinder the charge transfer to the analyte molecule, according to the chemical enhancement mechanism.28 In addition to SERS substrates, the nanorods can be used as nanocatalysts with high catalytic reactivity and selectivity for some chemical reactions owing to the synergistic catalytic effect of their constituent gold and silver components.29,30 For example, the results obtained by Monga et al. demonstrated that Au@AgNRs catalyze the selective formation of 3-nitroaniline in the reduction of 1,3-dinitrobenzene, compared to 1,3-phenylenediamine in the case of Au and Ag nanorods.31
Herein, we report the fabrication of a monodispersed Au@AgNR-loaded thermo-responsive composite nanofibrous membrane by electrospinning an aqueous solution containing poly(N-isopropylacrylamide-co-N-hydroxymethylacrylamide) and the nanorods, followed by heat treatment. Observations made using electron microscopy confirm that the Au@AgNRs are oriented along the axes of its constituent nanofibers. The composite nanofibrous membrane produced with a fast thermo-response and stable macroscopic morphology in aqueous media can be used as a smart free-standing SERS substrate with temperature-tunable efficiency. Particularly, it was found that this has the ability to in situ SERS monitor a catalyzed reaction, which is beneficial to understanding the reaction mechanism.
Experimental
Materials
Hexadecyltrimethylammonium bromide (CTAB, 99%), chloroauric acid trihydrate (HAuCl4·3H2O, 99.5%), sodium borohydride (NaBH4, 95%), silver nitrate (AgNO3, 99.8%), glycine (99.5%), hydrochloric acid (HCl, 37%), N-hydroxymethylacrylamide (NMA, 98%), 4-nitrothiophenol (4-NTP, 99%), 2,2′-azobis(isobutyronitrile) (AIBN, 99%) and sodium hydroxide (NaOH, analytical grade) were all purchased from Shanghai Chemical Reagent Co. and used as received. L(+)-Ascorbic acid (AA, 99%) was supplied by Acros Organics. Sodium oleate (NaOL, 97%) and N-isopropylacrylamide (NIPAM, 95%) were purchased from TCI America. NIPAM was purified by recrystallization from a mixture of toluene and hexane (60
:
40, v/v). Deionized water used in synthesis and characterization was made using a Millipore Direct-Q system.
Synthesis of monodisperse Au@AgNRs
Monodispersed AuNRs were synthesized by a binary surfactant method reported by Ye et al.32 The AuNRs were then used as seeds for the synthesis of monodispersed Au@AgNRs.26 Briefly, to 5 mL of 0.4 M glycine buffer solution at pH = 8.9 (adjusted with 1.0 M NaOH solution), 10 mL of the AuNR solution and a certain volume of 0.01 mM AgNO3 aqueous solution were added. Subsequently, 400 μL of 0.1 M ascorbic acid aqueous solution was added under gentle magnetic stirring. After 12 h, the solution was centrifuged for 15 min at 6000 rpm, the supernatant was removed and the precipitate was redispersed in deionized water.
Fabrication of Au@AgNRs loading thermo-responsive composite nanofibrous membrane
Firstly, poly(N-isopropylacrylamide-co-N-hydroxymethylacrylamide) (PNN) was synthesized by free radical polymerization in ethanol, as reported by Chen et al.33 The molar ratio of N-isopropylacrylamide to N-hydroxymethylacrylamide in the feed recipe is 5
:
1. A certain amount of purified Au@AgNRs dispersed in 1 mL of deionized water were then added to 3 mL of PNN aqueous solution of 30 wt% concentration, followed by vigorous stirring at room temperature for 24 h to produce an electrospinning solution of Au@AgNRs with a concentration of 25 nM, 50 nM or 100 nM. Subsequently, the electrospinning solution was loaded into a 10 mL plastic syringe and electrospun at a positive voltage of 13 kV, a working distance of 15 cm (distance between the needle tip and the grounded metal plate), and a flow rate of 0.9 mL h−1 for 4 h at room temperature. Finally, the nanofibrous membrane formed was heated at 110 °C for 5 h. Keeping the polymer concentration constant in the spinning solution, three kinds of Au@AgNRs/PNN composite nanofibrous membranes were fabricated by changing the concentration of the Au@AgNRs (from 25 nM to 50 nM to 100 nM), which are labeled as HNF-1, HNF-2 and HNF-3, respectively.
Characterization
A 1H NMR spectrum of PNN copolymer was obtained using a Bruker AV 400 nuclear magnetic resonance spectrometer based on the residual proton resonance of deuterated dimethylsulfoxide (DMSO-d6). Its number-average molecular weight was obtained using a Brookhaven BI-MwA gel permeation chromatograph equipped with a light scattering detector, using HPLC-grade tetrahydrofuran as eluent.
TEM images of Au@AgNRs/PNN composite nanofibrous membranes were taken with a JOEL 2100F transmission electron microscope at a voltage of 200 kV. The samples were collected on copper grids during electrospinning, followed by heating at 110 °C for 5 h. Their SEM images were obtained with a Hitachi S-4800 field emission scanning electron microscope at a voltage of 5 kV. The samples were sputtered with gold.
UV-vis adsorption spectra of diluted Au@AgNRs/PNN composite nanofiber aqueous dispersions at various temperatures were measured using a Perkin Elmer Lambda 35 UV-vis spectrometer with a temperature controller. A quartz cell with a 1 cm optical path length was used. Fourier transform infrared (FTIR) spectra of uncrosslinked PNN nanofibrous membranes and Au@AgNRs/PNN composite nanofibrous membranes were measured with a Thermo Fisher Nicolet 6700 FTIR spectrometer using an ATR attachment with a horizontal Ge crystal.
SERS measurements were performed using a Renishaw inVia-Reflex micro-Raman system equipped with a multi-channel charge-coupled device detector and a DM2500M Leica confocal microscope upon excitation by a 532, 633 or 785 nm laser source. All the SERS spectra were the results of 10 s accumulations. In order to assess the SERS efficiency of an Au@AgNRs/PNN composite nanofibrous membrane (size: 0.7 cm × 0.7 cm), it was put in the quartz sample cell, then 200 μL of 4-NTP aqueous solutions of different concentrations were injected into the cell, before recording SERS spectra using a Raman microscope with a 50× objective. Temperature-dependent SERS spectra were measured for a piece of membrane (HNF-3, 0.7 cm × 0.7 cm) that was immersed in 10−9 M 4-NTP aqueous solution in a Linkam THMS600 temperature-controllable cell, which was equilibrated thermally for at least 5 min before SERS measurement.
In situ SERS monitoring catalytic reaction
To test the ability of the Au@AgNRs/PNN composite nanofibrous membrane to monitor the catalytic reaction by SERS, the membrane (HNF-2) was immersed in 100 μL of a 10−4 M 4-NTP aqueous solution, and then 100 μL of a 0.1 M NaBH4 aqueous solution was injected into the sample cell, as schematically illustrated in Fig. 1(b). The reaction progress was monitored by the continuous collection of SERS signals (each scan from 300 cm−1 to 1800 cm−1 took ca. 5 min) from the membrane surface during the reaction. The reaction temperature in the cell can be controlled as above.
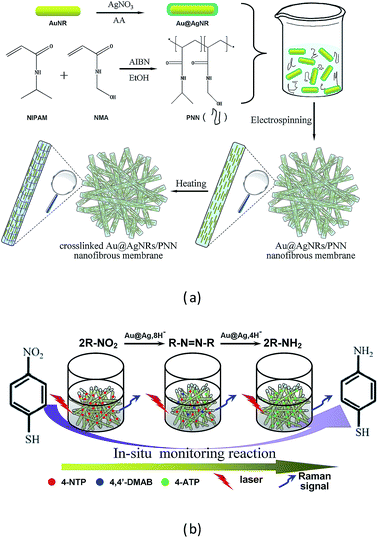 |
| Fig. 1 The fabrication route of thermo-responsive Au@AgNRs/PNN composite nanofibrous membrane (a) and its use in an in situ SERS monitored catalytic reaction (b). | |
Results and discussion
Fabrication and characterization of Au@AgNRs/PNN composite nanofibrous membranes
The fabrication route of an Au@AgNR-loading thermo-responsive composite nanofibrous membrane (Au@AgNRs/PNN composite nanofibrous membrane) is schematically illustrated in Fig. 1(a). Firstly, the fiber-forming thermo-responsive polymer, i.e. poly((N-isopropylacrylamide)-co-(N-hydroxymethylacrylamide)) (PNN), was synthesized via azobisisobutyronitrile-initiated free-radical polymerization in ethanol solution.33
Its NIPAM/NMA composition determined from its 1H NMR spectrum (see Fig. S1(a) in the (ESI)†) is 100
:
20.4 (molar ratio), very close to the feeding ratio of 100
:
20, and its number-average molecular weight estimated by gel permeation chromatography using tetrahydrofuran as eluent is 1.27 × 105 g mol−1. Fig. S1(b) in the ESI† shows the temperature-dependent absorbance (at 500 nm) of the diluted PNN aqueous solution, which exhibits a thermo-responsive hydrophilic-to-hydrophobic phase transition in aqueous media. Its lower critical solution temperature (LCST) is 41.8 °C, which is considerably higher than that of poly(N-isopropylacrylamide) (32 °C), due to the hydrophilicity of its constituting NMA moiety.34 Concurrently, monodispersed Au@AgNRs were prepared and purified by our recently reported method.26 A TEM image of the Au@AgNRs is shown in Fig. S2(a) in the ESI,† and we can note that they have excellent monodispersity and a well-defined core–shell structure. Their average length and diameter obtained by randomly measuring 50 particles are 81 ± 4 nm and 25 ± 2 nm, with the calculated aspect ratio being 3.2 and the average silver shell thicknesses of their lateral facets and two tips all being 2.7 ± 0.2 nm. The ultraviolet visible (UV-vis) spectrum of the diluted Au@AgNR aqueous dispersion and its optical image are shown in Fig. S2(b) in the ESI.† According to a previous report,26 the two bands at 708 nm and 505 nm are attributed to the longitudinal and transverse dipolar plasmon modes of the Au@AgNRs, respectively, and the band at 385 nm is ascribed to their octupolar plasmon mode. It can also be found from Fig. S2(b) in the ESI† that the longitudinal plasmon band of the Au@AgNRs is relatively narrow, further proving their monodispersity. In the electrospinning stage, PNN was mixed with an Au@AgNR aqueous dispersion to generate a spinning solution after stirring for 24 h. Subsequently, the solution was electrospun at a positive voltage of 13 kV, a working distance of 15 cm (distance between the needle tip and the grounded metal plate), and a flow rate of 0.9 mL h−1 at room temperature for 4 h. Finally, the nanofibrous membrane produced was heated at 110 °C for 5 h to form a crosslinking structure between the polymer chains within its constituent fibers, as illustrated in Scheme S1 in the ESI.†
Optical images of the Au@AgNRs/PNN composite nanofibrous membranes and the PNN nanofibrous membrane as control sample are shown in Fig. S3 in the ESI,† and it can be seen that the fabricated products are nonwoven membranes with an area of over 240 cm2 and a thickness of ca. 60 μm, and their colors in the dry state change from white to light green as their Au@AgNR content increases. Fig. 2 exhibits their scanning electron microscopy (SEM) and transmission electron microscopy (TEM) images at low and high magnification. From their SEM images, we can note that these membranes have three-dimensional networked structures consisting of many randomly deposited fibers, and the fibers are homogeneous in their diameters, without a beaded morphology. Their average diameters, determined by randomly measuring 50 fibers within each membrane, are 806 ± 112 nm, 682 ± 104 nm, 635 ± 34 nm and 607 ± 84 nm, decreasing as their Au@AgNR content increases. This behavior is presumably related to the increase in solution conductivity concomitant with the incorporation of Au@AgNRs.35 When observing the amplified SEM images of the nanofibers, the bright spots on their surfaces are the Au@AgNRs orienting along their axes, whose amounts are increased with the Au@AgNR concentration in their spinning solution. From their TEM images, we can clearly see that the Au@AgNRs are embedded within the fibers and are directionally aligned along their axis directions. As the Au@AgNR content increases within the nanofibers, the average end-to-end distance between the adjacent nanorods decreases from 146 nm to 89 nm to 41 nm. The low-magnification TEM images show that the oriented alignments are extremely uniform over long distances throughout the whole sample. In contrast to the spinning solution, where the Au@AgNRs are randomly dispersed, we can conclude that their oriented alignments inside the nanofibers result from the electrospinning process.36 There are two external fields that trigger such an alignment of the nanorods during the electrospinning process. One is the electric field supplied by the needle, and the other is the flow field formed during the formation of the fibers. When a high voltage was applied to the spinning solution, the whole solution was polarized. Furthermore, if the repulsive force within the charged solution was larger than its surface tension, a jet would erupt from the tip of the spinneret, producing the flow field. Shortly, the jet entered a bending instability stage, and the nanofibers were formed by the stretch and acceleration of the fluid filament in the region of instability as the solvent evaporated. During the formation of nanofibers, a sink-flow effect and strong shear forces were exerted on the Au@AgNRs, leading to their oriented alignment along the flow direction, which is the axial direction of the fibers. Even when a high concentration of the Au@AgNRs (100 nM) was present in the spinning solution, the nanorods were all directionally aligned, which demonstrates that electrospinning is a powerful technique for forming an oriented alignment of anisotropic nanorods on a large scale.
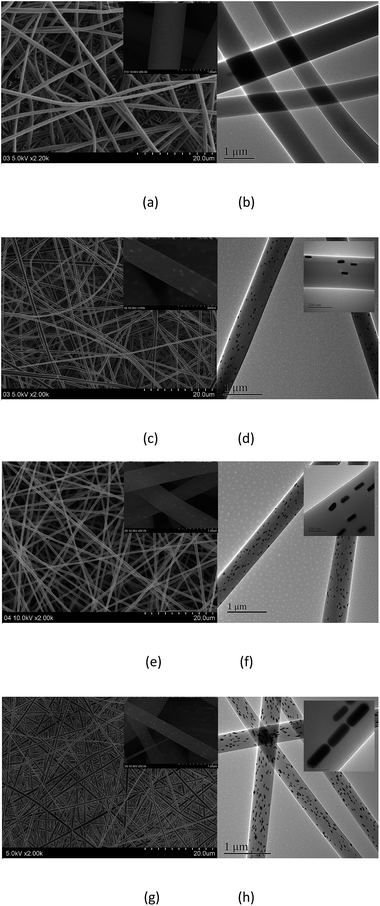 |
| Fig. 2 SEM (left column) and TEM (right column) images of Au@AgNRs/PNN composite nanofibrous membranes with different Au@AgNR contents (insets are their magnified images) (from top to bottom: control sample ((a) and (b)); HNF-1((c) and (d)); HNF-2 ((e) and (f)) and HNF-3 (g) and (h)). | |
Stability of Au@AgNRs/PNN composite nanofibrous membranes
Obviously, the stability of the Au@AgNRs/PNN composite nanofibrous membranes in aqueous media is important to their application, so it is necessary to evaluate it. For this purpose, about 40 mg of the membrane was immersed in 20 mL water and the solution was oscillated at 100 rpm for 2 h. Fig. S4 in the ESI† shows optical images of the control sample and Au@AgNRs/PNN composite nanofibrous membranes in water before and after oscillation. It can be seen that, after harsh oscillation, the control sample was slightly damaged, whereas the composite membranes were left intact, indicating that they have high stability in water. Hence, it could be speculated that interactions between the Au@AgNRs and the PNN polymer are present within the composite nanofibers, acting as physical crosslinking points. This speculation could be confirmed by the FTIR results, as illustrated in Fig. S5 in the ESI,† which shows FTIR spectra of an uncrosslinked PNN nanofibrous membrane and HNF-2. It can be found by comparing the two spectra that the intensity of the 3650 cm−1 peak assigned to the O–H stretching mode of NMA units within PNN is significantly decreased for HNF-2, indicating the occurrence of a crosslinking reaction between the polymer chains after heat treatment, as shown in Scheme S1 in the ESI.† Moreover, it is worthwhile noting that a small blue shift of amide I and II bands (1650 cm−1 and 1530 cm−1), attributed to the amide groups of PNN, appears in the spectrum of HNF-2, which may stem from the interactions between the nitrogen atoms in the groups and the Ag atoms on the loaded Au@AgNRs.12
Fast thermo-responsive properties of Au@AgNRs/PNN composite nanofibrous membranes
Typical optical images of an Au@AgNRs/PNN composite nanofibrous membrane immersed in water at 25 or 50 °C are exhibited in Fig. 3(a), and it can be seen that its area at 50 °C is much smaller than that at 25 °C, implying that it possesses remarkable thermo-responsive properties. To identify their phase-transition temperature, temperature-dependent areas of an Au@AgNRs/PNN composite nanofibrous membrane in water were measured at a heating rate of 0.1 °C min−1, and the results are depicted in Fig. 3(b). We can note that all the composite nanofibrous membranes notably shrink as the temperature increases from 25 °C to 50 °C, and their final area shrinking percentages are lowered from 82% to 52% as their Au@AgNR content rises. They all shrink suddenly around 41 °C, i.e. their phase transition temperature is slightly lower than the LCST of PNN. Moreover, the temperature range for the membrane area variation is wider than that for the hydrophilic-to-hydrophobic phase transition of PNN, which can presumably be attributed to the inhomogeneous distribution of the crosslinking points inside its nanofibers.37 Further investigation of their thermo-responsive properties involves assessing their thermo-response rates by measuring the time-dependent areas of membranes immersed alternately into water at 25 or 50 °C after an interval of 2 min, and the results are shown in Fig. 3(c). It is well-known that a deswelling poly(N-isopropylacrylamide) bulky membrane requires a few days to reach an equilibrium state, which is ascribed to the formation of a dense skin layer on the gel surface hindering diffusion of the water.38 In comparison, Au@AgNRs/PNN composite nanofibrous membranes shrunk to a deswelling equilibrium state within 2 seconds after a jump in temperature from 25 to 50 °C (see the attached video). On the other hand, they reversibly swelled to 95% of their initial areas within 10 seconds and reached a swelling equilibrium state after the temperature dropped from 50 to 25 °C. The reversible deswelling–swelling cycle was repeated at least 3 times. With respect to the fast stimulus-response rate of the smart nanofibrous membranes, this is generally thought to be associated with the very small diameters of the constituting nanofibers and the porous structure of the membrane, which facilitates the transfer of the stimulus to the whole material.9,16 To clarify the reason for their fast and remarkable response to temperature change, we tried to visualize the microstructures of Au@AgNRs/PNN composite nanofibrous membranes at swelling or deswelling equilibrium states comparatively. For this purpose, an equilibrium-swollen nanofibrous membrane in water at 25 °C or 50 °C was rapidly transferred into liquid nitrogen for freezing and then freeze-dried for SEM observation. The rapid freezing by liquid nitrogen should immobilize the microstructure of the membrane in water and the sizes of its constituent nanofibers. From the SEM images of two freeze-dried samples, as shown in Fig. 3(a), we can find that the nanofibers are well separated with a very large mesh structure within the membrane being obtained from its equilibrium-swelling state in water at 25 °C, whereas they adhere with each other to form bundles of fibers, leading to a dense mesh structure within that from its equilibrium-deswelling state in water at 50 °C. When carefully visualizing their amplified SEM images, it can be noted that the nanofibers within the shrunken membrane are slightly thinner than those inside the swollen membrane. Thus, we can suppose that the shrinkage of the composite nanofibrous membrane in water at 50 °C should mostly arise from adhesion between the nanofibers and formation of fibrous bundles, leading to a steep decrease in the free space inside its interior. With regard to what makes the nanofibers adhere to each other, we think that it is the hydrophobic interaction between the nanofibers after their polymers undergo a temperature-induced hydrophilic-to-hydrophobic phase transition. If too many Au@AgNRs are located on their surfaces, the hydrophobic interactions will be weakened. This is why the area-shrinking percentage of the Au@AgNRs/PNN composite nanofibrous membranes is lowered as their Au@AgNR content rises, as depicted in Fig. 3(b).
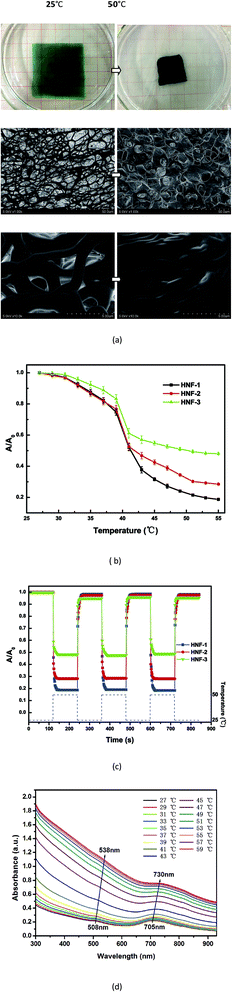 |
| Fig. 3 (a) Optical images of HNF-2 immersed in water at 25 and 50 °C and SEM images of the membranes prepared by liquid nitrogen freezing and then freeze-drying. (b) Temperature-dependent area change of Au@AgNRs/PNN composite nanofibrous membranes (3.6 cm × 3.6 cm) in pure water measured at a heating rate of 0.1 °C min−1. (c) Reversibility of time-dependent deswelling and swelling of Au@AgNRs/PNN composite nanofibrous membranes (3.6 cm × 3.6 cm) immersed alternately in water at 25 °C or 50 °C after 2 min. (d) UV-visible spectra of an aqueous dispersion of the nanofibers at different temperatures. | |
Subsequently, it is essential to investigate the effect of temperature change on the plasmonic properties of the Au@AgNRs embedded within the composite nanofibrous membrane. To this end, an aqueous dispersion of nanofibers was prepared by adding a small amount of the broken membrane (HNF-2) to water, followed by sonicating the solution for 5 h, and UV-vis spectra in the range 300–1100 nm at different temperatures were obtained, as shown in Fig. 3(d). Firstly, the longitudinal plasmon band of the Au@AgNRs embedded within the membrane becomes broader compared to that before electrospinning (Fig. S1(b) in the ESI†), which may arise from their plasmonic coupling due to their approaching inside its constituent nanofibers. Secondly, as the temperature rises from 39 °C to 47 °C, close to the temperature range of the area change of the membrane, both their transverse and longitudinal plasmon bands are abruptly enhanced, due to the refractive index increase of the dispersed nanofibers after the temperature-induced phase transition. Thirdly, their maximum wavelengths are both red-shifted within the same temperature range, which could be due to two reasons. One is that the collapsed polymer network around the Au@AgNRs could increase their surrounding refractive index.39 However, as investigated by Schmidt et al.,40 this contribution to the red-shift of the wavelengths should be limited owing to a slight variation in the refractive index before and after the phase transition. Another is that the temperature-triggered phase transition of the composite nanofibers could reduce the distances between the loaded Au@AgNRs. As reported by Sánchez-Iglesias, in the gold-nanorod-pair system, side-to-side interactions lead to a red-shift of the transverse plasmon band and a blue-shift of the longitudinal plasmon band, while tip-to-tip ones would red-shift both bands.41 In our system, both the tip-to-tip and side-to-side distances between the Au@AgNRs should be shortened after the temperature-driven phase transition of their lodged nanofibers due to their directional alignment along the fibers' axes, as evidenced above. However, it can be deduced from the red-shift of their longitudinal plasmon band that their tip-to-tip interactions are dominant over their side-to-side ones, due to the possibility that the longitudinal percent shrinkage of the nanofibers is larger than the transverse one.
Use of Au@AgNRs/PNN composite nanofibrous membrane as a SERS substrate and its temperature-tunable SERS efficiency
Compared to previously reported smart composite microgels,13,14 the Au@AgNRs/PNN composite nanofibrous membrane should be more suitable for use as a SERS substrate because of its free-standing and flexible features.36 Here, its SERS efficiency was evaluated using 4-NTP as a standard Raman-active probe molecule. Fig. S6(a) in the ESI† shows the SERS spectra of a 4-NTP aqueous solution with the same concentration, obtained using three excitation wavelengths, i.e. 532 nm, 633 nm and 785 nm. The typical Raman peaks of 4-NTP are reflected in the spectra, including peaks at 1080 cm−1, 1334 cm−1 and 1571 cm−1, attributed to C–S stretching, O–N
O symmetric stretching and the phenyl ring stretching modes, respectively.42 From Fig. S6(a) in the ESI,† we can see that the membrane as a substrate exhibits the strongest SERS efficiency with an excitation wavelength of 785 nm, since this is the closest to the longitudinal plasmon resonance wavelength of its entrapped Au@AgNRs, resulting in the strongest surface electric field enhancement effect. Au@AgNRs/PNN composite nanofibrous membranes with three different Au@AgNR contents were used as substrates to measure the SERS spectra of a 10−7 M 4-NTP aqueous solution at 25 °C, and the results are given in Fig. S6(b) in the ESI.† A plot of their 1334 cm−1 peak intensities against the Au@AgNRs concentrations in their spinning solutions is depicted in Fig. S6(c) in the ESI.† It can be seen that their SERS efficiency is nearly proportionally increased with the Au@AgNR concentration, revealing that their SERS efficiency comes from the bimetallic nanorods. The composite nanofibrous membranes were used as substrates to measure the SERS spectra of different concentrations of 4-NTP solution from 10−13 M to 10−7 M at 25 °C; the result for HNF-2 is given in Fig. 4(a) and the others in Fig. S7 in the ESI.† As the 4-NTP concentration increases, its SERS intensity is progressively raised. Even on the HNF-1 substrate, a SERS signal of 10−12 M 4-NTP can be clearly observed (see Fig. S7(b) in the ESI†). When the membrane, HNF-3, was used as a SERS substrate, 10−13 M 4-NTP in aqueous solution could be detected (Fig. S7(e) in the ESI†). A plot of the 1334 cm−1 peak intensities in Fig. 4(a) versus the logarithmic concentrations (log(c)) of 4-NTP is shown in Fig. 4(b) and can be fitted into a line with a correlation coefficient of 0.993 (n = 9). This result shows that there is a good linear relationship between the SERS intensity and the logarithmic concentration of the analyte, indicating that the SERS signal based on the Au@AgNRs/PNN composite nanofibrous membrane as substrate is highly reproducible. A high SERS signal reproducibility should arise from the monodispersed and homogeneous distribution of the Au@AgNRs within its constituent nanofibers. In addition, fifteen measurements of 10−6 M 4-NTP aqueous solution were carried out on the same membrane (HNF-2), and the obtained SERS spectra are exhibited in Fig. 4(c). The estimated relative standard deviation (RSD) of the intensities of their 1334 cm−1 peaks is 3.87%, further confirming high reproducibility of the SERS signals from the membrane. These results mean that the Au@AgNRs/PNN composite nanofibrous membrane could be used as a SERS substrate for quantitative analysis.
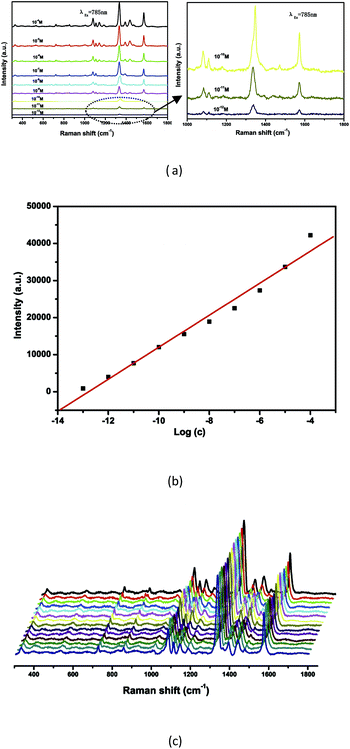 |
| Fig. 4 (a) SERS spectra of different concentrations of a 4-NTP aqueous solution at 25 °C based on HNF-2 as substrate with 785 nm laser excitation; (b) plot of the SERS intensity of the 1334 cm−1 peak in (a) vs. log(c) of 4-NTP; (c) SERS spectra of 10−6 M 4-NTP acquired from 15 different spots on the membrane, HNF-2. | |
The effect of temperature on the SERS efficiency of the Au@AgNRs/PNN composite nanofibrous membrane was investigated using 10−9 M 4-NTP aqueous solution as the analyte, as illustrated in Fig. 5(a). One can observe that all the peaks in its SERS spectrum are remarkably enhanced as the temperature is increased from 20 °C to 50 °C. A plot of the 1334 cm−1 peak intensity as a function of temperature is exhibited in Fig. 5(b). A marked change occurs within the temperature range from 35 °C to 45 °C, in agreement with that for the area change of the composite nanofibrous membrane with temperature. It can be inferred from this that the temperature-driven SERS signal enhancement possibly results from plasmonic coupling between approaching Au@AgNRs after temperature-triggered adhesion between their constituent nanofibers, as previously discussed.13 This result implies that the detection sensitivity based on the Au@AgNRs/PNN composite nanofibrous membrane as a SERS substrate can be improved by elevating the detection temperature. This estimation has been confirmed through SERS detecting of 10−13 M 4-NTP in aqueous solution at 50 °C using HNF-2 as substrate, as shown in Fig. 5(c) and (d). By comparing the results obtained at 25 °C and 50 °C, the low detection limit of 4-NTP at the higher detection temperature is lowered by one order of magnitude. Because of its fast thermo-response rate, the swelling or deswelling equilibrium of the membrane as a SERS substrate at each detection temperature can be quickly reached, which is beneficial with respect to shortening the measurement time.
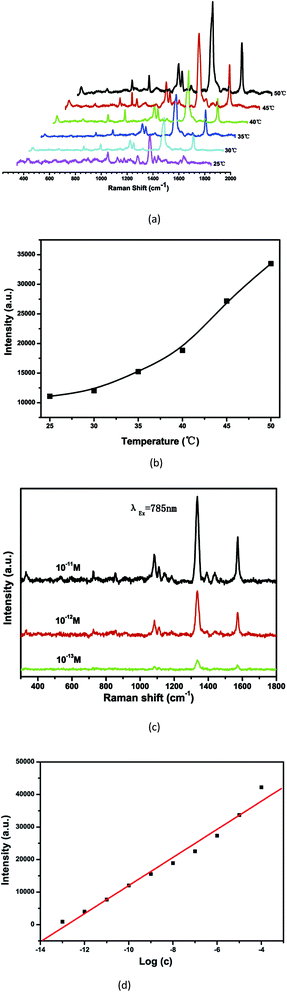 |
| Fig. 5 (a) SERS spectra of 10−9 M 4-NTP aqueous solution using the membrane, HNF-3, as substrate at different temperatures; (b) the peak intensity at 1334 cm−1 in (a) as a function of temperature; (c) SERS spectra of different concentrations of 4-NTP in aqueous solution at 50 °C based on the membrane, HNF-2, as substrate; (d) plot of the intensity of the 1334 cm−1 peak in (c) vs. log(c) of 4-NTP. | |
Application of Au@AgNRs/PNN composite nanofibrous membrane for in situ SERS monitoring of its catalyzed reaction
As reported recently,31 Au@AgNRs possess high catalytic activity and selectivity for some reactions, so it is expected that a flexible free-standing Au@AgNRs/PNN composite nanofibrous membrane with macroscopic strength can be used for label-free in situ SERS monitoring of its catalyzed reaction. To validate this expectation, the reduction of 4-NTP to 4-aminothiophenol (4-ATP) by NaBH4 in the presence of the membrane was selected as a model reaction, as schematically illustrated in Fig. 1(b). This can be considered as a two-step consecutive reaction (see Scheme S2 in the ESI†), either by a direct route via a hydroxylamine intermediate (4-thiolphenylhydroxylamine) or a condensation route through the formation of an azobenzene intermediate (4,4′-dimercaptoazobenzene (4,4′-DMAB)).43,44 In the direct route, the nitro group of 4-NTP is reduced into nitroso, hydroxylamine, and amino groups successively. In the condensation route, an azobenzene compound is generated as an intermediate from the condensation of a nitroso compound and a hydroxylamine compound, and it is finally reduced into two amino compounds. Therefore, detection of the intermediate is crucial to clarifying the reaction mechanism. Fig. 6 displays the time-dependent SERS spectra, which were recorded at 5 min intervals after adding the NaBH4 solution to 10−4 M 4-NTP solution containing the membrane (HNF-2) at 25 °C (from top to bottom). At the top of the SERS spectrum, only the Raman peaks of 4-NTP are present. After addition of NaBH4, its peaks are gradually weakened and three new peaks at 1140, 1385 and 1427 cm−1 appear accordingly, which can be assigned to the C–N symmetric stretching, N
N stretching, and C–H in-plane bending modes of 4,4′-DMAB, respectively.42 Their intensities initially increase with reaction time (from black to green spectra) and then gradually decrease to near-zero (from green to yellow ones), corroborating its character as an intermediate. In addition to the peaks of 4,4′-DMAB, a new peak at 1590 cm−1 shows up almost simultaneously and gradually dominates the spectrum, which is attributed to the amino vibrational mode of the product, 4-ATP. The results indicate that the reduction of 4-NTP into 4-ATP by NaBH4, catalyzed by the Au@AgNRs/PNN composite nanofibrous membrane, proceeds via a condensation route. The mechanism can be explained by the speculation that the transformation of 4-NTP to 4,4′-DMAB may be caused by plasmon-induced charge transfer under the laser irradiation.45 Concretely, the reaction could be catalyzed via electron transfer between the laser-irradiation-induced plasmon resonance surface of Au@AgNR and the nitro group of 4-NTP through the molecule, because of its chemisorption on the Ag surface via its thiol group.
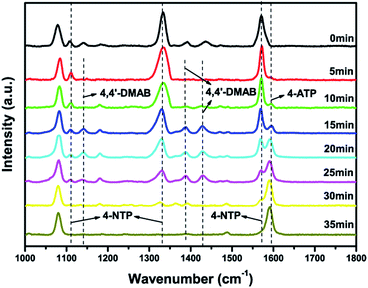 |
| Fig. 6 SERS spectra for in situ monitoring the reduction of 4-NTP catalyzed by the membrane, HNF-2. | |
Conclusion
In summary, monodispersed Au@AgNRs were successfully loaded into a thermo-responsive nanofibrous membrane via an electrospinning process, followed by heat treatment. The prominent structural feature of the produced composite nanofibrous membrane is oriented alignment of the nanorods along the axes of its constituent fibers, so their tip-to-tip and side-to-side interactions can be tuned by temperature, resulting in temperature-tunable plasmonic properties. Compared to previously reported thermo-responsive composite materials based on thermo-responsive bulky hydrogels or microgels, the composite nanofibrous membrane has both a fast thermo-response and macroscopic mechanical strength. It can be used as a smart free-standing SERS substrate with high signal reproducibility for quantitative analysis, and its SERS efficiency can be increased by elevating the temperature. Moreover, the composite nanofibrous membrane has the ability to in situ SERS monitor its catalyzed reaction, which is beneficial to understanding the reaction mechanism.
Acknowledgements
The authors are grateful to the National Natural Science Foundation of China (51373030, 51503033) and the Fundamental Research Funds for the Central Universities (2232014D3-43). They gratefully acknowledge Prof. S. Agarwal of Universität Bayreuth for her valuable suggestions.
Notes and references
- M. A. C. Stuart, W. T. S. Huck, J. Genzer, M. Mueller, C. Ober, M. Stamm, G. B. Sukhorukov, I. Szleifer, V. V. Tsukruk, M. Urban, F. Winnik, S. Zauscher, I. Luzinov and S. Minko, Nat. Mater., 2010, 9, 101–113 CrossRef PubMed.
- H. Zhang, J. S. Han and B. Yang, Adv. Funct. Mater., 2010, 20, 1533–1550 CrossRef CAS.
- X. H. Li, G. Y. Chen, L. B. Yang, Z. Jin and J. H. Liu, Adv. Funct. Mater., 2010, 20, 2815–2824 CrossRef CAS.
- M. Molina, M. Asadian-Birjand, J. Balach, J. Bergueiro, E. Miceli and M. Calderon, Chem. Soc. Rev., 2015, 44, 6161–6186 RSC.
- M. Karg, Colloid Polym. Sci., 2012, 290, 673–688 CAS.
- I. Tokarev and S. Minko, Soft Matter, 2012, 8, 5980–5987 RSC.
- R. Messing and A. M. Schmidt, Polym. Chem., 2011, 2, 18–32 RSC.
- S. G. Cao, B. H. Hu and H. Q. Liu, Polym. Int., 2009, 58, 545–551 CrossRef CAS.
- S. H. Jiang, F. Y. Liu, A. Lerch, L. Ionov and S. Agarwal, Adv. Mater., 2015, 27, 4865–4870 CrossRef CAS PubMed.
- J. Perez-Juste, I. Pastoriza-Santos and L. M. Liz-Marzan, J. Mater. Chem. A, 2013, 1, 20–26 CAS.
- M. Karg and T. Hellweg, J. Mater. Chem., 2009, 19, 8714–8727 RSC.
- Y. Y. Liu, X. Y. Liu, J. M. Yang, D. L. Lin, X. Chen and L. S. Zha, Colloids Surf., A, 2012, 393, 105–110 CrossRef CAS.
- X. Y. Liu, X. Q. Wang, L. S. Zha, D. L. Lin, J. M. Yang, J. F. Zhou and L. Zhang, J. Mater. Chem. C, 2014, 2, 7326–7335 RSC.
- X. Y. Liu, C. Zhang, J. M. Yang, D. L. Lin, L. Zhang, X. Chen and L. S. Zha, RSC Adv., 2013, 3, 3384–3390 RSC.
- X. Dong, X. B. Zou, X. Y. Liu, P. Lu, J. M. Yang, D. L. Lin, L. Zhang and L. S. Zha, Colloids Surf., A, 2014, 452, 46–50 CrossRef CAS.
- C. B. Huang, S. J. Soenen, J. Rejman, B. Lucas, K. Braeckmans, J. Demeester and S. C. De Smedt, Chem. Soc. Rev., 2011, 40, 2417–2434 RSC.
- S. Ramakrishna, K. Fujihara, W. E. Teo, T. Yong, Z. W. Ma and R. Ramaseshan, Mater. Today, 2006, 9, 40–50 CrossRef CAS.
- I. D. Kim, A. Rothschild, B. H. Lee, D. Y. Kim, S. M. Jo and H. L. Tuller, Nano Lett., 2006, 6, 2009–2013 CrossRef CAS PubMed.
- C. L. Zhang and S. H. Yu, Chem. Soc. Rev., 2014, 43, 4423–4448 RSC.
- F. Mitschang, H. Schmalz, S. Agarwal and A. Greiner, Angew. Chem., Int. Ed., 2014, 53, 4972–4975 CrossRef CAS PubMed.
- W. E. Teo and S. Ramakrishna, Nanotechnology, 2006, 17, R89–R106 CrossRef CAS PubMed.
- C. Huang, S. J. Soenen, J. Rejman, B. Lucas, K. Braeckmans, J. Demeester and S. C. De Smedt, Chem. Soc. Rev., 2011, 40, 2417–2434 RSC.
- R. B. Jiang, H. J. Chen, L. Shao, Q. Li and J. F. Wang, Adv. Mater., 2012, 24, OP200–OP207 CAS.
- Z. Y. Bao, D. Y. Lei, R. B. Jiang, X. Liu, J. Y. Dai, J. F. Wang, H. L. W. Chan and Y. H. Tsang, Nanoscale, 2014, 6, 9063–9070 RSC.
- E. C. Cho, P. H. C. Camargo and Y. N. Xia, Adv. Mater., 2010, 22, 744–748 CrossRef CAS PubMed.
- X. Dong, J. F. Zhou, X. Y. Liu, D. L. Lin and L. S. Zha, J. Raman Spectrosc., 2014, 45, 431–437 CrossRef CAS.
- T. N. A. Dao, P. Singh, C. Shankar, D. Mott and S. Maenosono, Appl. Phys. Lett., 2011, 99, 073107 CrossRef.
- M. Erol, Y. Han, S. K. Stanley, C. M. Stafford, H. Du and S. Sukhishvili, J. Am. Chem. Soc., 2009, 131, 7480–7481 CrossRef CAS PubMed.
- X. Guo, Q. Zhang, Y. H. Sun, Q. Zhao and J. Yang, ACS Nano, 2012, 6, 1165–1175 CrossRef CAS PubMed.
- S. Jayabal and R. Ramaraj, Appl. Catal., A, 2014, 470, 369–375 CrossRef CAS.
- A. Monga and B. Pal, Colloids Surf., A, 2015, 481, 158–166 CrossRef CAS.
- X. Ye, C. Zheng, J. Chen, Y. Gao and C. B. Murray, Nano Lett., 2013, 13, 765–771 CrossRef CAS PubMed.
- L. N. Chen, Y. C. Chiu, J. J. Hung, C. C. Kuo and W. C. Chen, Macromol. Chem. Phys., 2014, 215, 286–294 CrossRef CAS.
- W. D. Zhang, W. Zhang, N. C. Zhou, Z. P. Cheng, J. Zhu and X. L. Zhu, Polymer, 2008, 49, 4569–4575 CrossRef CAS.
- C. D. Saquing, J. L. Manasco and S. A. Khan, Small, 2009, 5, 944–951 CrossRef CAS PubMed.
- C. L. Zhang, K. P. Lv, H. P. Cong and S. H. Yu, Small, 2012, 8, 648–653 CrossRef CAS PubMed.
- C. Wu, S. Q. Zhou, S. C. F. Auyeung and S. H. Jiang, Angew. Makromol. Chem., 1996, 240, 123–136 CrossRef CAS.
- R. Yoshida, K. Uchida, Y. Kaneko, K. Sakai, A. Kikuchi, Y. Sakurai and T. Okano, Nature, 1995, 374, 240–242 CrossRef CAS.
- H. X. Xu, J. Xu, Z. Y. Zhu, H. W. Liu and S. Y. Liu, Macromolecules, 2006, 39, 8451–8455 CrossRef CAS.
- S. Schmidt, H. Motschmann, T. Hellweg and R. von Klitzing, Polymer, 2008, 49, 749–756 CrossRef CAS.
- A. Sánchez-Iglesias, M. Grzelczak, J. Pérez-Juste and L. M. Liz-Marzán, Angew. Chem., Int. Ed., 2010, 49, 9985–9989 CrossRef PubMed.
- G. C. Zheng, L. Polavarapu, L. M. Liz-Marzan, I. Pastoriza-Santos and J. Perez-Juste, Chem. Commun., 2015, 51, 4572–4575 RSC.
- A. Grirrane, A. Corma and H. Garcia, Science, 2008, 322, 1661–1664 CrossRef CAS PubMed.
- A. Corma, P. Concepcion and P. Serna, Angew. Chem., Int. Ed., 2007, 46, 7266–7269 CrossRef CAS PubMed.
- W. Xie, B. Walkenfort and S. Schluecker, J. Am. Chem. Soc., 2013, 135, 1657–1660 CrossRef CAS PubMed.
Footnote |
† Electronic supplementary information (ESI) available. See DOI: 10.1039/c6ra04247c |
|
This journal is © The Royal Society of Chemistry 2016 |
Click here to see how this site uses Cookies. View our privacy policy here.