DOI:
10.1039/C5RA26746C
(Paper)
RSC Adv., 2016,
6, 21865-21870
Surface-enhanced Raman spectroscopy on porous silicon membranes decorated with Ag nanoparticles integrated in elastomeric microfluidic chips†
Received
14th December 2015
, Accepted 10th February 2016
First published on 12th February 2016
Abstract
A microfluidic sensing device is fabricated by the integration of silver-coated porous silicon membranes as surface enhanced Raman scattering active nanostructures in a PDMS matrix showing plasmon resonances in the visible energy range. The membranes are shaped by defining a suitable pattern of the electrochemically etched silicon using the cover of the elastomeric chip. 4-Mercaptobenzoic acid, used as a probe molecule, shows Raman enhancement larger than 107 in electronic off-resonance conditions. The calibration of the SERS substrate is performed using a Langmuir isotherm fit, evidencing the analyte chemisorption. In order to check the potential of the chip as a multiplexed biosensing platform, model oligonucleotides (polyadenine, polyguanine, polythymine and polycytosine) are analyzed in a multichamber system.
Introduction
Most of the analysis in biological assays, which involve the recognition of a specific target molecule by a suitable binding probe, requires a multianalyte method. In such approaches, different species (i.e. oligonucleotides or proteins) are comparatively tested on the same sensing platform, with applications ranging from biomedicine to food analysis and safety. Virus serotype discrimination or food authenticity assessment using comparisons of known genomic sequences are representative examples.1–3 Moreover, recent advances in biomedicine have demonstrated the relationship between the appearance of some pathologies and the altered relative concentrations of certain molecule families in biofluids. A case of great interest is concerned with micro-RNAs (miRNA), short non-coding ribonucleic acid regulatory sequences, which are over- or under-expressed in tumor patients.4 Early diagnosis should therefore take into account the expression profile of many sequences, rather than the concentration of a single specific miRNA, highlighting the need for a reliable multianalyte sensing platform.
Surface Enhanced Raman Scattering (SERS)-active substrates assembled as arrayed plasmonic elements can be exploited for these applications.5,6 They combine a multiplexing approach with the high sensitivity of the SERS technique taking advantage of a label free detection, which allows the minimization of the sample pre-treatments, in contrast to fluorescent microarray analysis. The analytes can be detected in trace concentrations and recognized through their vibrational fingerprints thanks to the Raman scattering enhancement provided by their interaction with nanostructured noble metal surfaces supporting localized surface plasmons (LPSs), without the need for any labeling steps.7
Recently, the microfluidic integration of SERS substrates has also attracted significant attention focused on chemical and biological analysis.8–10 Actually, the microfluidic set-up can solve problems involving a non-uniform molecular distribution on the SERS substrate surface and reduce the risks of sample contamination.11,12 Moreover, microfluidics can enable the in-flow synthesis of nanoparticles and dynamic detection benefiting from improved reaction kinetics with a limited reagent consumption. In-chip SERS analysis can finally exploit detection with portable Raman spectrometers, reducing instrumentation-related costs for point-of-care applications.
Solid SERS substrates are increasingly employed in microfluidic platforms due to their easier integration and increased stability with respect to colloidal nanoparticles, whose aggregation behavior in the fluidic channels has to be strictly controlled.13 Among them, silver coated porous silicon (Ag-pSi) has been previously studied for its high SERS sensitivity and wide plasmon resonance tunability.14,15 Because of the fabrication process, which involves the electrochemical etching of a silicon wafer in a HF electrolyte, followed by immersion plating in silver nitrate solutions,16,17 such structures have been so far used as “open SERS substrates”.
In this work, we present an easy fabrication method devoted to the preparation of a polydimethylsiloxane (PDMS) multi-chamber SERS-based microfluidic chip hosting Ag-coated porous silicon membranes (Ag-pSi). This device exploits features such as a low cost of fabrication, flexibility and optical transparency in the NIR-Vis energy range. pSi membranes featuring a suitable shape are etched through a thin PDMS mask and transferred onto a PDMS substrate. The silver nanoparticles are grown on the pSi-PDMS membranes on which a PDMS cover is finally bonded.
The optofluidic chip enables efficient and calibrated SERS analysis. In particular, a multianalyte detection configuration using different isolated chambers is here demonstrated for model oligonucleotides.
Experimental
Chip materials
The PDMS (Sylgard 184, Dow Corning) cover and substrate of the chip were prepared by casting in micromachined PMMA molds. A schematic diagram of the cover mold is shown in Fig. S1 of the ESI,† while a simple square mold allowed the preparation of the PDMS slice used as the substrate, which was cut according to the rounded shape of the cover after bonding. The PDMS oligomer and curing agent were mixed in a 20
:
1 weight ratio, degassed at room temperature and cured in an oven at 60°. A 10
:
1 mixture was used for PDMS mask preparation. Porous silicon was etched starting from p+-type crystalline silicon18 (resistivity 34–40 mΩ cm) in a 20
:
20
:
60 water
:
hydrofluoric acid
:
ethanol solution. The current density and the anodization time were set to 30 mA cm−2 and 50 s in order to obtain the desired porosity and thickness of the porous layer. A 4
:
4
:
92 HF
:
H2O
:
ethanol solution was employed for the partial detachment of pSi membranes (etching time = 95 s, current density = 4 mA cm−2). The masking procedure and transfer of the membranes on PDMS are detailed in the Results and discussion section.
Synthesis of silver nanoparticles
Before the nanoparticle synthesis, a refreshing pre-treatment of the PDMS-pSi membranes in 10% aqueous HF solution was needed to remove the oxidized porous silicon regions formed during the electrochemical detachment and curing. Silver nanoparticles were then synthesized by immersion plating of the pre-treated substrate in aqueous silver nitrate (10−2 M) for 5 minutes at 55 °C.
Characterization techniques
Field Emission Scanning Electron Microscopy (FESEM) viewgraphs of the detached bare and silver-coated pSi membranes were acquired as secondary electron contrast images with 10 keV electrons using the in-lens detector of a Zeiss SUPRA 40 (Zeiss SMT, Oberkochen, Germany) microscope. Samples were either directly detached with FESEM conductive tape (for bare pSi) or covered with a copper grid anchored to the FESEM stub to allow discharging (for PDMS-supported Ag-pSi membranes).
Specular reflectance spectra of the silvered samples were recorded in the 300–1200 nm range by means of a UV-Vis-NIR spectrophotometer (Agilent, Santa Clara, CA, USA) equipped with a 12.5° reflectance unit.
Raman measurements were performed using a Renishaw InVia Reflex micro-Raman spectrometer (Renishaw plc, Wotton-under-Edge, UK) with a 514.5 nm diode laser excitation using the multichamber chip in backscattering light collection. 5 mW laser power, 5 s of exposure time and 10 accumulations were employed to record each spectrum. 1 s exposure time and 2 accumulations were used instead for the mapping measurements.
4-Mercaptobenzoic acid (4-MBA), chosen as a SERS probe molecule, was diluted in ethanol at different concentrations and 2 μl of each solution was injected into the chambers. After static incubation for 30 minutes the solution was withdrawn with a micropipette and the chambers were washed with ethanol before the SERS measurement. 50 microns × 50 microns Raman maps (2.5 μm step) were acquired along the Ag-pSi membranes to average the 4-MBA signal intensity over a larger area in order to determine a calibration curve.
Multianalyte oligonucleotide detection
Cover blocking was performed before the sealing of the device using incubation of the open PDMS chambers with Bovine Serum Albumin (BSA) 1% buffered solutions (50 mM Na–acetate buffer, pH 4.0) for 1 h at room temperature, followed by washing in TE buffer (pH 7.5) to remove the excess protein. Customized oligonucleotide homosequences (6 nts polyadenine, polyguanine, polycytosine and polythymine) were then dissolved in TE-buffer (pH 7.5) at 100 μM concentration and injected into the four different chambers to probe the multianalyte potential of the chip.
Results and discussion
Multichamber chip fabrication
Fig. 1 illustrates the fabrication scheme of the multi-chamber chip (a complete version equipped with pictures at each step is shown in Fig. S2 of ESI†). The first steps involve the patterning of electrochemically etched porous silicon. Different techniques have been developed in the past, including the deposition of silicon nitride or photoresist masks and dry-removal soft lithography, reaching a sub-micrometer resolution in some cases.19–22 Here, an alternative process inspired by micro-contact printing is applied, providing a versatile, easy and inexpensive patterning approach. The geometry of the pattern is defined by the cover of the multichamber microfluidic device, which is employed as a stamp to deliver a PDMS precursor mask on a silicon dice. A thin layer of unpolymerized PDMS mixture is first spread onto a glass slide (Fig. 1a). The PDMS cover, hosting a four drop-shaped chambers design, is then placed onto the uncured PDMS layer (Fig. 1b). The contact occurs only in the regions external to the chambers, where the uncured PDMS thin film is caught on the cover after detachment (Fig. 1c). The PDMS layer is then transferred onto a p+ silicon dice by placing the cover on it for few seconds (Fig. 1d) and stabilized by crosslinking at 80 °C for 1 h. Thanks to this treatment, the PDMS layer acts as a mask during the subsequent porosification process (Fig. 1e), which is achieved by electrochemical etching of the crystalline silicon in hydrofluoric acid solution. After pore growth, the etchant is replaced by low concentration HF and pSi is further anodized at a low current density, enlarging the pores at the Si/pSi interface until a partial membrane detachment occurs. The pSi membranes still lie on the silicon support until a 1 mm thick partially cross-linked PDMS membrane (curing time reduced to 10 minutes at 80 °C) is placed in contact with the etched silicon sample to complete the membrane detachment (Fig. 1f). The porous silicon membranes are readily transferred onto the PDMS substrate thanks to the enhanced adhesive properties of the non-fully cured material, rich with free chains, which allow an efficient bonding. When the initial silicon substrate is removed by stripping, the porous membranes are immobilized on the PDMS support (Fig. 1g). Ultrathin membranes can be fabricated using this method (down to 300 nm or less) as the porous silicon is never totally free-standing during the process. The pSi-PDMS sample is finally cured for 10 minutes at 80 °C to complete the crosslinking reaction of the elastomeric material and reinforce the bonding.
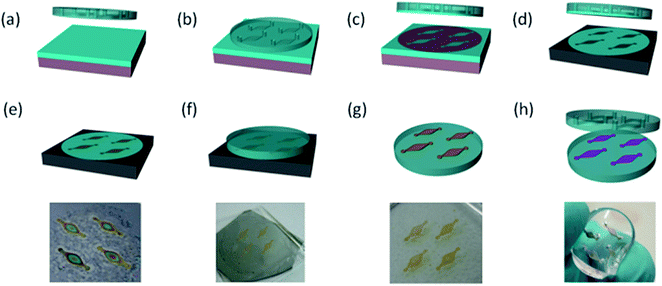 |
| Fig. 1 Scheme of fabrication. (a) PDMS precursor mixture spread on a smooth surface (i.e. a glass slide); (b) PDMS cover (hosting drop-shaped chambers) placed on the mixture; (c) cover removal; (d) stamp of the cover pattern on the silicon dice; (e) patterned pSi membranes after HF anodization; (f) partially cross-linked PDMS on the pSi membranes; (g) pSi membranes transferred onto the PDMS substrate; and (h) expanded vision of the silver-coated optofluidic chip. Steps from (e) to (h) are shown along with the corresponding digital photographs. | |
The silver nanoparticles are synthesized before the sealing of the device by dipping the pSi membranes bonded to the PDMS support in aqueous silver nitrate (Fig. 1h). Finally, the cover is aligned to the substrate and the bonding is achieved by adding a thin PDMS precursor mixture at the interface between the two components, followed by final crosslinking at 60 °C for 1 h. The fabricated device is characterized by four isolated chambers, including one silver-coated porous silicon membrane as the SERS active element, each equipped with inlet and outlet channels.
Morphological, optical and Raman characterizations
FESEM viewgraphs of the bare and silver coated pSi layer are shown (top and bottom respectively) in Fig. 2a. A good quality porous silicon membrane is prepared, presenting an average pore diameter of 20 nm. Homogeneous pore size along the thickness of the membrane (thickness around 870 nm) can be observed (Fig. S3b of the ESI†). These results are in agreement with the values extracted by the simulation of the experimental specular reflectance spectrum of the bare pSi membranes (0.9 microns of thickness and 64% of porosity) characterized by typical Fabry–Perot interference fringes (Fig. 2b dashed line).
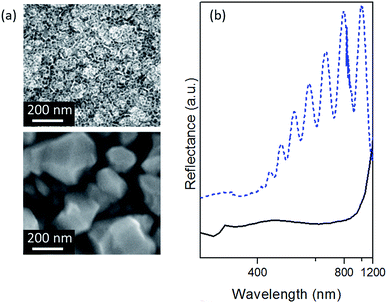 |
| Fig. 2 SERS substrate characterization. (a) FESEM images of the bare porous silicon (top), silver-coated pSi-PDMS sample (bottom) and (b) the corresponding specular reflectance spectra (dashed and solid line, respectively). | |
After immersion plating of the pSi-PDMS specimen in AgNO3, its surface is covered with silver nanoparticles, whose sizes are distributed with a certain polydispersion. Despite the irregular morphology, small inter-particle gaps of a few nanometers are distributed over all of the pSi membrane surface. Under light excitation, the electric near-field intensity can be huge within such nano-gaps, due to LSP coupling. This yields the well-known hot-spots, giving the highest Raman enhancements.23 Specular reflectance spectra of silver-coated pSi (Fig. 2b solid line) are consistent with the observed morphology, showing a very broad dip, covering a wavelength range of 400–800 nm, which arises from the convolution of many plasmon resonances ascribed to enhanced absorption/scattering processes related to LSPs coupled either to individual particles or through inter-particle interactions.
The SERS response of the chip is tested by employing 4-mercaptobenzoic acid (4-MBA), which is increasingly used as a probe molecule in SERS due to its non-resonant electronic behavior under the most common excitation conditions in the visible-NIR range.24,25 This can be easily demonstrated by the collection of a 4-MBA UV-Vis absorption spectrum, which is characterized by two intense bands at 217 and 270 nm (Fig. S4†). Raman measurements are therefore performed using a 514.5 nm laser, which provides an excitation within the plasmon band of the Ag-coated pSi membrane, while keeping off-electronic resonance conditions for the 4-MBA analyte.
Ag-pSi-PDMS samples are tested by filling the chambers of the optofluidic device with 4-MBA ethanolic solutions at increasing concentrations, starting from 10−9 M up to 10−2 M. Selected spectra are reported in Fig. 3a, while the whole series is provided in Fig. S5.† The 4-MBA vibrational fingerprint is dominated by the ν12 of the aromatic ring coupled with the C–S stretching at 1077 cm−1 and the ν8a ring mode at 1584 cm−1.26 These peaks can be already identified with a good signal-to-noise ratio after incubation with the 10−8 M solution. A pSi-PDMS chip sample without nanoparticles is also incubated with 4-MBA solution as a reference, without the final washing step to avoid the removal of the non-specifically adsorbed thiol. A 4-MBA signal cannot be detected until a 10−1 M concentration is reached, due to the absence of the plasmon enhancement giving a SERS regime. This measurement allows the calculation of the external amplified Raman efficiency14 (EARE) of the Ag-pSi-PDMS substrate, defined as the ratio of the minimum concentration of 4-MBA detectable on the Ag-coated sample to the one on the bare pSi-PDMS surface. Due to the complex morphology the estimation of the actual amount of adsorbed molecules is quite hard and the enhancement factor cannot be calculated. An EARE of ∼ 107 is derived, which can be essentially ascribed to the plasmonic resonant excitation of the Ag-pSi-PDMS SERS substrate.
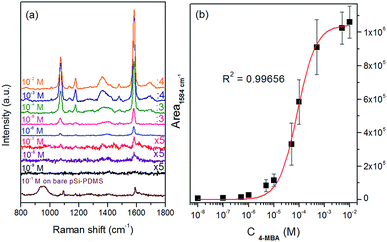 |
| Fig. 3 Raman of 4-MBA. (a) SERS spectra of 4-MBA from 10−9 to 10−2 M on the Ag-pSi-PDMS substrate. Spectra are rescaled by the factors indicated on the right side of the plot. (b) Langmuir fit of the area of the 1584 cm−1 peak vs. 4-MBA concentration. | |
The integrated area of the most intense peak (1584 cm−1) of 4-MBA as a function of the concentration is extracted by averaging the Raman signal using SERS maps in order to obtain a calibration curve. The Raman intensity data, which show a monotonic increase vs. the analyte concentration, can be successfully fitted by a Langmuir isotherm with a very good R2 value of 0.99. Actually, the Langmuir model only applies to equilibrium processes in which chemisorption of the analyte on a fixed number of binding sites occurs. This behavior is reasonable for 4-MBA whose thiol group is able to strongly bind to the silver surface.27 Saturation of the Raman signal at concentrations higher than 5 × 10−3 M can be also explained by the saturation of the surface adsorption sites.
The analyses here discussed imply that the chip can be calibrated and employed in quantitative analysis.
Multianalyte biosensing
The multianalyte SERS detection is finally performed in the four chambers of the chip. Model oligonucleotides, consisting of short homonucleotidic sequences of the four DNA nucleobases are employed, as a consequence of their chemical and structural similarity to microRNAs, whose analysis can represent one of the possible applications of the presented SERS-based optofluidic device. Although the selected sequences do not show the complexity of real targets that are interesting for diagnostic purposes, which are characterized by the presence of different bases, their detection in the four isolated chambers represents a proof-of-concept of the applicability of the chip to multianalyte SERS biodetection.
A pre-treatment of the cover with BSA is needed to avoid oligonucleotide adsorption on the PDMS walls, which exhibit a high affinity to the pyrimidine and purine fragments of the oligos.28
Polyadenine (polyA), polyguanine (polyG), polythymine (polyT) and polycytosine (polyC) solutions are prepared in a TE buffer and injected into the four chambers respectively. The SERS signal can be recorded sequentially under the 20× objective of the Raman microscope both with the chambers filled by the solutions and after solvent evaporation. A picture of the four-chamber chip used in the experiment is shown in Fig. 4b along with the spectra recorded at 514.5 nm after drying of the oligo solution (Fig. 4a).
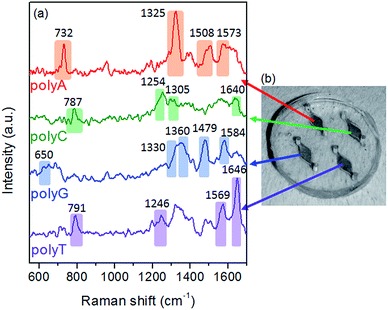 |
| Fig. 4 Multianalyte SERS. (a) SERS spectra of polyA, polyC, polyG and polyT in the four different chambers. (b) Picture of the optofluidic chip. | |
The spectra are dominated by nucleobase modes, which preferably adsorb on the silver surface, while the backbone (OPO and PO2− stretching vibrations at 760, 830 and 1090 cm−1) and deoxyribose modes (870 and 1030 cm−1)29 are poorly visible. Taking into account previous experimental findings concerning the Raman detection of oligonucleotides by SERS colloidal systems or by TERS, the fingerprint of each sequence can be easily recognized. The polyA spectrum is characterized by its distinctive ring breathing at 730 cm−1 and the intense C–N stretching band at 1325 cm−1. The polyG can be recognized by a C–N ring stretching peak located at a higher Raman shift (1360 cm−1) and the intense 1480 cm−1 and 1580 cm−1 bands, attributed to C–N stretching/N–H bending and NH2 scissoring respectively.30 Pyrimidine based oligonucleotides show typical features around 790 cm−1 (6-member ring breathing) and 1640 cm−1 (carbonyl stretching). Moreover, the spectra can be distinguished by the different fingerprints in the 1200–1300 cm−1 range, where a doublet is observed for polyC at 1256–1305 cm−1, while a single peak at 1246 cm−1 represents the C–N stretching for polyT.31
It is worth underlining that the PDMS peaks expected as interfering features in the Raman spectra are not observed despite the elastomeric cover of the device being crossed twice by the excitation beam. This can be ascribed to the focusing of the excitation beam at the membranes surface, which provides the SERS enhancement close to the Ag nanoparticles.
Conclusions
An elastomeric multi-chamber SERS active optofluidic chip is developed by patterning pSi membranes through a PDMS mask, defined by the chip cover shape, with a successive transfer of the pSi membranes, providing the synthesis of Ag nanoparticles, on a PDMS substrate. The good SERS efficiency (enhancement of > 7 orders of magnitude) and calibrated response are demonstrated using 4-MBA as non-resonant probe molecule. The multianalyte detection of different oligonucleotides is successfully performed in the four isolated chambers. These results validate the optofluidic chip as an efficient label-free detection system in a multiplexing approach.
Acknowledgements
This research received funding from the Italian Flagship Project NANOMAX and the Project FIRB NEWTON.
Notes and references
- M. B. Miller and Y. W. Tang, Clin. Microbiol. Rev., 2009, 22, 611–633 CrossRef CAS PubMed.
- S. Fernandez, E. D. Cisney, A. P. Tikhonov, B. Schweitzer, R. J. Putnak, M. Simmons and R. G. Ulrich, Clin. Vaccine Immunol., 2011, 18, 523–532 CrossRef CAS PubMed.
- M. M. Voorhuijzen, J. P. Van Dijk, T. W. Prins, A. M. A. Van Hoef, R. Seyfarth and E. J. Kok, Anal. Bioanal. Chem., 2012, 402, 693–701 CrossRef CAS PubMed.
- H. Dong, J. Lei, L. Ding, Y. Wen, H. Ju and X. Zhang, Chem. Rev., 2013, 113, 6207–6233 CrossRef CAS PubMed.
- C. Novara, F. Petracca, A. Virga, P. Rivolo, S. Ferrero, A. Chiolerio, F. Geobaldo, S. Porro and F. Giorgis, Nanoscale Res. Lett., 2014, 9, 527 CrossRef PubMed.
- Q. Yang, M. Deng, H. Li, M. Li, C. Zhang, W. Shen, Y. Li, D. Guo and Y. Song, Nanoscale, 2015, 7, 421–425 RSC.
- K. Kneipp, H. Kneipp, I. Itzkan, R. R. Dasari and M. S. Feld, J. Phys.: Condens. Matter, 2002, 14, 597–624 CrossRef.
- W. Ruan, W. Ji, X. Xue, Y. Cui, L. Chen, T. Zhou, L. Niu, X. Li, J. Zhang and B. Zhao, J. Raman Spectrosc., 2011, 42, 1492–1496 CrossRef CAS.
- M. Lee, K. Lee, K. H. Kim, K. W. Oh and J. Choo, Lab Chip, 2012, 12, 3720–3727 RSC.
- A. Lamberti, A. Virga, A. Chiadò, A. Chiodoni, K. Bejtka, P. Rivolo and F. Giorgis, J. Mater. Chem. C, 2015, 3, 6868–6875 RSC.
- Q. Li, B. Li and Y. Wang, RSC Adv., 2013, 3, 13015 RSC.
- A. Lamberti, A. Virga, A. Angelini, A. Ricci, E. Descrovi, M. Cocuzza and F. Giorgis, RSC Adv., 2015, 5, 4404–4410 RSC.
- C. Wang and C. Yu, Nanotechnology, 2015, 26, 092001 CrossRef CAS PubMed.
- A. Virga, P. Rivolo, F. Frascella, A. Angelini, E. Descrovi, F. Geobaldo and F. Giorgis, J. Phys. Chem. C, 2013, 117, 20139–20145 CAS.
- A. Virga, P. Rivolo, E. Descrovi, A. Chiolerio, G. Digregorio, F. Frascella, M. Soster, F. Bussolino, S. Marchiò, F. Geobaldo and F. Giorgis, J. Raman Spectrosc., 2012, 43, 730–736 CrossRef CAS.
- F. A. Harraz, T. Tsuboi, J. Sasano, T. Sakka and Y. H. Ogata, J. Electrochem. Soc., 2002, 149, C456 CrossRef CAS.
- H. Lin, J. Mock, D. Smith, T. Gao and M. J. Sailor, J. Phys. Chem. B, 2004, 1, 11654–11659 CrossRef.
- M. J. Sailor, Porous Silicon in Practice: Preparation, Characterization and Applications, Wiley-VCH, Weinheim, 2012 Search PubMed.
- A. G. Nassiopoulos, S. Grigoropoulos, L. Canham, A. Halimaoui, I. Berbezier, E. Gogolides and D. Papadimitriou, Thin Solid Films, 1995, 255, 329–333 CrossRef CAS.
- D. J. Sirbuly, G. M. Lowman, B. Scott, G. D. Stucky and S. K. Buratto, Adv. Mater., 2003, 15, 149–152 CrossRef CAS.
- I. Rea, A. Lamberti, I. Rendina, G. Coppola, M. Gioffrè, M. Iodice, M. Casalino, E. De Tommasi, L. De Stefano, I. Rea, A. Lamberti, I. Rendina and G. Coppola, J. Appl. Phys., 2013, 107, 014513 CrossRef.
- B. S. Flavel, M. J. Sweetman, C. J. Shearer, J. G. Shapter and N. H. Voelcker, ACS Appl. Mater. Interfaces, 2011, 3, 2463–2471 CAS.
- E. C. Le Ru and P. G. Etchegoin, J. Chem. Phys., 2009, 130, 4–7 CrossRef PubMed.
- J. Yan, X. Han, J. He, L. Kang, B. Zhang, Y. Du, H. Zhao, C. Dong, H. L. Wang and P. Xu, ACS Appl. Mater. Interfaces, 2012, 4, 2752–2756 CAS.
- S. Zhu, C. Fan, J. Wang, J. He and E. Liang, Appl. Phys. A, 2014, 117, 1075–1083 CrossRef CAS.
- A. Michota and J. Bukowska, J. Raman Spectrosc., 2003, 34, 21–25 CrossRef CAS.
- Y. Fleger, Y. Mastai, M. Rosenbluh and D. H. Dressler, Surf. Sci., 2009, 603, 788–793 CrossRef CAS.
- C. Thibault, V. Le Berre, S. Casimirius, E. Trévisiol, J. François and C. Vieu, J. Nanobiotechnol., 2005, 3, 1 CrossRef PubMed.
- S. Najjar, D. Talaga, L. Schué, Y. Coffinier, S. Szunerits, R. Boukherroub, L. Servant, V. Rodriguez and S. Bonhommeau, J. Phys. Chem. C, 2014, 118, 1174–1181 CAS.
- B. Giese and D. McNaughton, Phys. Chem. Chem. Phys., 2002, 4, 5171–5182 RSC.
- S. E. J. Bell and N. M. S. Sirimuthu, J. Am. Chem. Soc., 2006, 128, 15580–15581 CrossRef CAS PubMed.
Footnote |
† Electronic supplementary information (ESI) available: Cover mold scheme, detailed fabrication scheme, FESEM cross section of the transferred pSi membrane, 4-MBA UV-Vis absorption spectrum, SERS spectra of 4-MBA at several concentrations. See DOI: 10.1039/c5ra26746c |
|
This journal is © The Royal Society of Chemistry 2016 |
Click here to see how this site uses Cookies. View our privacy policy here.