DOI:
10.1039/C5RA20507G
(Paper)
RSC Adv., 2016,
6, 21854-21864
Sulfamic acid-functionalized nano-titanium dioxide as an efficient, mild and highly recyclable solid acid nanocatalyst for chemoselective oxidation of sulfides and thiols†
Received
3rd October 2015
, Accepted 18th February 2016
First published on 18th February 2016
Abstract
A highly efficient and retrievable titanium dioxide-based nanocatalyst has been synthesized by covalent grafting of chlorosulfonic acid on amine-functionalized titania as a novel inorganic–organic hybrid heterogeneous nanocatalyst, which was characterized by Fourier transform infrared spectroscopy (FT-IR), field emission scanning electron microscopy (FE-SEM), X-ray diffraction (XRD) and thermogravimetric analysis (TGA). The acid strength of the catalyst was determined by pH analysis and the Hammett acidity function. The potential of the resultant nanocatalyst was effectively evaluated for the chemoselective oxidation of sulfides to sulfoxides and thiols to disulfides using 30% H2O2 as an oxidant at room temperature under solvent-free conditions, which led to high conversion rates and yields. Optimization of the reaction conditions was studied by central composite design (CCD), which is one of the most widely used response surface methodologies. The catalyst can be easily recycled up to 10 times without significant decrease in catalytic activity, which makes it a promising catalyst for practical and large-scale applications. This work is the first report that uses 2,4-toluene diisocyanate as a linker for immobilizing liquid acid on a support.
1. Introduction
Homogeneous acid catalysts are widely used in a variety of organic transformations, including hydrolyses, aldol condensations, acylations, nucleophilic additions, etc. However, several drawbacks such as highly corrosive and hazardous nature, separation problems, reactor corrosion, waste neutralization and especially inability to be reused are associated with the reactions catalyzed by homogeneous liquid acids.1 Hence, overcoming the aforementioned problems remains a challenge to chemists and is still strongly desired. Following the successful immobilization of chlorosulfonic acid on silica support which was reported by Zolfigol,2 avenues for design and engineering of new and interesting solid acid catalysts opens up and there is now a growing interest in the further development of this type of catalysts.3–11 The use of solid heterogeneous catalysts for the development of clean processes is a theme of extreme importance in the chemical industries due to better regio- and stereoselectivity, low toxicity, high stability, easy separation, moisture resistance, air tolerance, easy handling and reusability.12,13
The highest degree of evolution in the surface immobilization strategy is the covalent attachment of an organic component to a solid support.14 The combination of nanometer-sized inorganic moieties with organic compounds in a single material has extraordinary significance for development of multifunctional materials so-called inorganic–organic hybrids and can improve their ability to act in a variety of academic or technological activities. An obvious advantage of such hybrids is the desirable combination of both organic and inorganic properties in one nanomaterial.
In this study, we have reported a novel strategy to immobilize sulfonic acid groups on the surface of TiO2 nanoparticles, by employing 2,4-toluene diisocyanate (TDI) as a covalently bound linker. Compared with the expensive conventional coupling agents (titanate and silane coupling agents) employed to organically modify the surface of TiO2 nanoparticle, TDI is readily available, low-cost, and very active due to its highly unsaturated bonds. Furthermore, the reaction between TiO2 nanoparticle and TDI occurs fast, easily and without any catalyst. To the best of our knowledge, there are no literature reports on investigating the usage of TDI as a linker in the catalyst modifications and catalytic activity of TDI-based compounds. So we have decided to estimate the catalytic applicability of the synthesized catalyst for the oxidation of sulfides to sulfoxides and also oxidative coupling of thiols into corresponding disulfides. Sulfoxides are fine chemicals, pharmaceuticals and valuable intermediates in the synthesis of chemically useful and biologically active molecules.15,16 Likewise, disulfides are essential moieties of biologically active compounds and possess wide variety of chemical and industrial applications.17,18 Concerning their importance, there has been a plethora of research targeting these transformations under either catalyst-free condition19 or in the presence of various catalysts,20–22 using different oxidants.
2. Result and discussion
In extension of our ongoing programs on preparation of new heterogeneous catalysts for organic reactions,23–26 we wish to report the preparation and application of sulfamic acid-functionalized nano-TiO2 as a novel, efficient and recyclable nanocatalyst (Scheme 1). TDI is utilized to establish a bridge between the surface of nano-titania and sulfonic acid to improve the recyclability and lifetime of the inorganic–organic hybrid catalyst. Such a methodology provides a rigid covalent linkage between the support and sulfonic acid moiety.
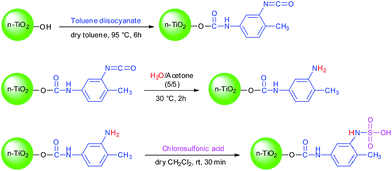 |
| Scheme 1 Preparation of sulfamic acid-functionalized n-TiO2 (n-TiO2-NHSO3H). | |
As illustrated in Scheme 1, at the first step, n-TiO2 was easily synthesized by hydrothermal method according to the literature report.27 Afterward, TiO2 nanoparticle surface was treated with TDI and accessible surface hydroxyls of nano TiO2 preferentially reacted with a para-isocyanate group of TDI to form urethane bond28,29 and left an ortho-isocyanate group unreacted due to the different reactivity of the two isocyanate groups together with steric hindrance in TDI molecule.30 At the next step, the unreacted isocyanate group was converted to amine by treating it with water and a new solid base is produced which could probably find unique uses in various technical, industrial and organic applications. Finally, the n-TiO2-NH2 nanoparticle was transformed to n-TiO2-NH-SO3H via a condensation reaction with chlorosulfonic acid. This novel nanocatalyst was characterized via FT-IR, FE-SEM, XRD, TGA, acid–base titration and Hammett acidity function method.
2.1. Characterization of sulfamic acid-functionalized n-TiO2 (n-TiO2-NHSO3H)
2.1.1. FT-IR spectra. In order to evaluate successful conjugation of sulfamic acid onto the surface of the nano-TiO2, FT-IR spectra of the nano-TiO2 and every step of the reaction was analyzed. As shown in Fig. 1a, the broad intense band below 1200 cm−1 is attributed to Ti–O–Ti vibration and the absorbance at 1623 and 3355 cm−1 are assigned to the surface hydroxyl groups of TiO2.31 Curve b in Fig. 1 shows the FT-IR spectrum of TDI. The absorption peak at 2243 cm−1 owing to stretching vibration of NCO, which agrees with the literature values,32 is its main peak. The peaks at 1550 cm−1 are reasonably attributed to the phenyl ring of TDI. FT-IR spectrum of TDI-functionalized n-TiO2 (Fig. 1c), compared with the spectrum of the pristine TiO2 nanoparticle (Fig. 1a), is revealed additional peaks at 2266, 1649, 1595, and 1533 cm−1. The peak at 2266 cm−1 can be attributed to the unreacted ortho-isocyanate groups of TDI attached to the n-TiO2 surface, while the bands at 1649 and 1595 cm−1 can be assigned to the C
O and C–N stretches indicating that the reaction of toluene diisocyanate with titania takes place through the formation of a urethane bond (OCONH)33 and the peak at 1533 cm−1 is related to the phenyl ring of TDI. These results clearly confirmed that the TDI molecule was covalently linked to TiO2 nanoparticle. According to (Fig. 1d), by using water, the isocyanate peak at 2266 cm−1 is disappeared and converted to amine group which is overlapped with N–H bond of urethane bond at 3267 cm−1. Reaction of n-TiO2-NH2 with chlorosulfonic acid produces n-TiO2-NHSO3H, in which the presence of sulfonyl moiety is indicated by the absorptions in 1222 and 1159 cm−1 which are certified the O
S
O asymmetric and symmetric stretching modes lies respectively and the O–H stretch is appeared as a very broad band from 2400 to 3400 cm−1 as can be seen in Fig. 1e.
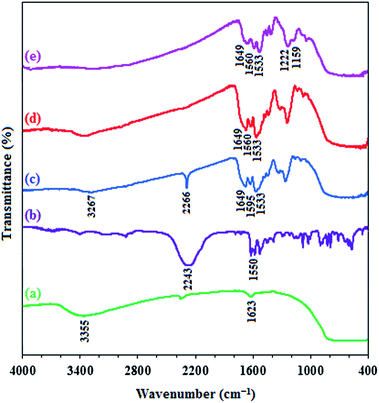 |
| Fig. 1 FT-IR spectra of (a) nano-TiO2, (b) TDI, (c) n-TiO2-NCO, (d) n-TiO2-NH2 and (e) n-TiO2-NHSO3H. | |
2.1.2. Field emission scanning electron microscopy. To study the surface morphology of nano-TiO2 and modified nano-TiO2 nanostructures, FE-SEM micrographs were employed. The field emission scanning electron microscopy (FE-SEM) (Fig. 2a) and transmission electron microscopy (TEM) (Fig. 2b) analysis of nano TiO2 powder indicates the spherical shape with average size of 20–30 nm. The FE-SEM micrographs of the n-TiO2-NH2 (Fig. 2c) and n-TiO2-NHSO3H (Fig. 2d) show an enlargement in particles diameter sizes to about 50–80 nm and 90–140 nm respectively. In n-TiO2-NHSO3H, aggregation of n-TiO2 nanoparticles is clearly seen.
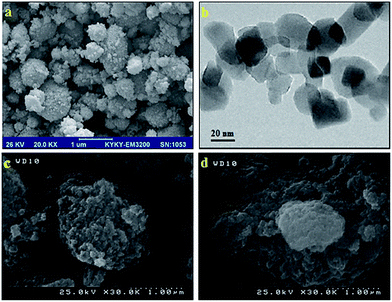 |
| Fig. 2 FE-SEM image of n-TiO2 (a), TEM image of n-TiO2 (b), FE-SEM image of n-TiO2-NH2 (c), FE-SEM image of n-TiO2-NHSO3H (d). | |
2.1.3. X-ray diffraction spectra. X-ray diffraction measurement was performed to investigate the influence of modifications on the surface of nano support. As shown in Fig. 3a, the typical diffraction signals located at (101), (103), (004), (112), (200), (105), (211), (204), (220), (215) and (224) can be well assigned to the diffraction of TiO2 nanoparticles with anatase crystallite phase which matched with the standard XRD data of JCPD card number (89-4921). The average n-TiO2 diameter for 2θ = 25.303° was calculated to be around 22 nm from the XRD results by Debye–Scherrer's equation, D = kλ/β
cos
θ, where k is a constant (generally considered as 0.94), λ is the wavelength of Cu-Kα (1.54 Å), β is the corrected diffraction line full-width at half-maximum (FWHM), and θ is Bragg's angle.34 According to Fig. 3, the position and relative intensities of all peaks in the XRD pattern of n-TiO2-NH2 and n-TiO2-NHSO3H confirm well with XRD pattern of nano-TiO2, indicating retention of the anatase crystallite phase structure during functionalization of nano-TiO2 (Fig. 3b).
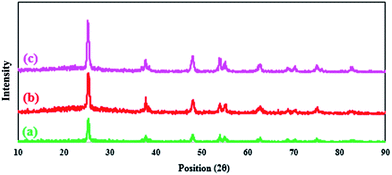 |
| Fig. 3 The X-ray diffraction patterns of the (a) nano-TiO2, (b) n-TiO2-NH2, (c) n-TiO2-NHSO3H. | |
2.1.4. Thermo gravimetric analysis. One indication of bond formation between the nanoparticles and the catalyst can be inferred from thermogravimetric analysis (TGA). Fig. 4 shows thermogravimetric analysis (TGA) curves of nano-TiO2 and n-TiO2-NHSO3H in the temperature range 30–800 °C. The results of Fig. 4a indicate that nano-TiO2 showed a weight change (4 wt%) below 100 °C due to the loss of the physically adsorbed water and then had a slight weight loss (1 wt%) between 100 °C and 800 °C, which could be ascribed to the dehydroxylation of n-TiO2 according to literatures.35 The thermograph of the n-TiO2-NHSO3H depicts three stages decomposition. The first region is below 100 °C which is attributed to the loss of trapped water from the catalyst. The second weight loss (11%) in the region between 225 and 308 °C correlates quite well with that for TDI-modified titania which is in good agreement with data reported by other authors.36 The third step at curve b (T > 314 °C) is attributed to decomposition of either TDI or the grafted SO3H groups. On the basis of these results, the well grafting of TDI and sulfonic acid groups on the n-TiO2 is verified.
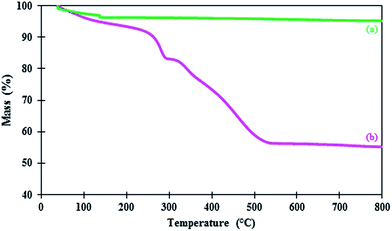 |
| Fig. 4 TGA curves of (a) nano-TiO2, (b) n-TiO2-NHSO3H. | |
2.1.5. pH analysis of catalyst. To determine the Brönsted acid amount on the surface of catalyst, the prepared catalyst (100 mg) was ion-exchanged with 10 mL of 1 M NaCl solution at room temperature by sonication, followed by filtration and washing with 5 mL deionized water. Then, the filtrate was titrated with NaOH (0.01 M) solution in the presence of pH meter. The required volume of NaOH to reach equivalence point was 10 mL which is equal to a loading of 1 mmol g−1 of sulfamic acid group.
2.1.6. Surface acidity studies. The acidity of solid samples is commonly reported as a function of Hammett acidity (H0).37 Using this method, the solid samples are placed in the presence of indicator dyes with known pKa values (mainly substituted nitroanilines), wherein the indicator trapped the dissociated proton of the catalyst. Then, UV-visible spectrometer is used to determine the concentrations of protonated and unprotonated indicator molecules exactly according to the Lambert–Beer law.The Hammett acidity function could be calculated with the following equation:
H0 = pK(I)aq + log([I]s/[IH+]s) |
where, ‘I’ displays the indicator base (here 4-nitroaniline) and [IH
+]
s and [I]
s are the molar concentrations of the protonated and unprotonated forms of indicator respectively. The p
K(I)
aq is the protonation constant of the dye in aqueous solution (for example the p
K(I)
aq value of 4-nitroaniline is 0.99).
In this experiment, 4-nitroaniline was selected as the basic indicator, and CCl4 was chosen as the solvent due to its aprotic property. First, a dilute solution (1.44 × 10−4 M) of 4-nitroaniline in CCl4 was prepared and used as a stock solution. The maximum absorbance for the unprotonated indicator in UV-vis spectra was observed at 331 nm as shown in Fig. 5. Then, 20 mg of the catalyst was added to the solution and mixed for 30 min with magnetic stirring, and the UV spectrum of the solution was recorded. By adding catalyst, the absorbance of the unprotonated form of the indicator decreased to 1.276 due to trapping the acidic protons.
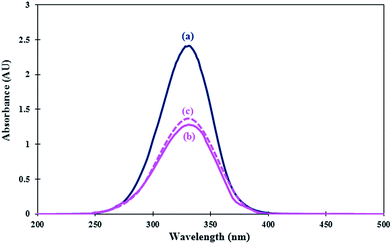 |
| Fig. 5 Absorption spectra of (a) 4-nitroaniline (indicator), (b) nano-TiO2-NHSO3H (catalyst) and (c) recovered catalyst after ten runs in CCl4. | |
The difference in the measured absorbance is used to determine the acid strength of the catalyst (Table 1).
Table 1 Hammett acidity function (H0) data for n-TiO2-NHSO3Ha
Entry |
Catalyst |
Amax |
[I]s (%) |
[IH+]s (%) |
H0 |
Condition for UV-visible spectrum measurement: solvent, CCl4; indicator, 4-nitroaniline (pK(I)aq = 0.99), 1.44 × 10−4 mol L−1; catalyst, n-TiO2-NHSO3H (20 mg), 25 °C. |
1 |
— |
2.410 |
100 |
0 |
— |
2 |
n-TiO2-NHSO3H |
1.276 |
64.79 |
35.21 |
1.44 |
3 |
Recovered after ten runs |
1.359 |
69.44 |
30.56 |
1.50 |
The results of Table 1 approves that the SO3H groups were successfully tethered on the support (Table 1, entry 2) and catalyst leaching is partial and acidic groups are well grafted on TDI (Table 1, entry 3).
2.2. Evaluation of catalytic activity of n-TiO2-NHSO3H for the oxidation of sulfides and thiols
The selective oxidation of sulfides to sulfoxides is a fundamental and important functional group transformation in organic synthesis because organic sulfoxides are useful building blocks in the construction of pharmaceuticals, agrochemicals, and other valuable fine chemicals and in drug metabolisms.38–40 Therefore, we investigated catalytic activity of n-TiO2-NHSO3H as a heterogeneous nanocatalyst for the chemoselective oxidation of structurally diverse sulfides to sulfoxides using 30% H2O2 as an oxidant (Scheme 2).
 |
| Scheme 2 Nano-TiO2-NHSO3H catalyzed oxidation of sulfides to sulfoxides using H2O2. | |
For this purpose, the reaction of methyl phenyl sulfide and hydrogen peroxide as a model reaction was probed to establish the feasibility of the strategy and optimize the reaction conditions. In order to find the optimal conditions, the central composite design (CCD), one of the most applicable types of response surface model (RSM), was applied. It has been accepted as an effective optimization method in attainment to improved responses via a fitted quadratic model which can be expressed by following equation:
In which Y is response, Xi and Xj are independent variables, β0 is the constant model, βi, βii and βij are coefficients for the linear, quadratic and interaction effects, respectively.
First of all, preliminary experiments were carried out to investigate the appropriate parameters and to determine the experimental domain. Considering these experiments, the effects of catalyst amount (X1), H2O2 concentration (X2), and reaction time (X3) were performed on reaction yield as response. A five-level CCD of three independent variables with their corresponding values is shown in Table 2.
Table 2 The experimental levels of variables in CCD for the synthesis of methyl phenyl sulfoxide
Independent variables |
Levels |
Lowest (−1.68) |
Low (−1) |
Central (0) |
High (+1) |
Highest (+1.68) |
X1: catalyst amount (mg) |
6.64 |
8 |
10 |
12 |
13.36 |
X2: H2O2 (equiv.) |
1.13 |
1.4 |
1.8 |
2.2 |
2.47 |
X3: time (min) |
6.59 |
10 |
15 |
20 |
23.40 |
Based on CCD method, total number of experiments was found to be 20 which consisted of eight full factorial points, six axial points and six central points. Conditions of 20 trials companied to their respective yields are shown in Table 3.
Table 3 Conditions of predicted experiments in CCD for three effective factors in the synthesis of methyl phenyl sulfoxide
Run |
X1 |
X2 |
X3 |
Yield (%) |
1 |
−1 |
−1 |
+1 |
47.76 |
2 |
0 |
0 |
0 |
90.43 |
3 |
0 |
0 |
0 |
92.88 |
4 |
+1.68 |
0 |
0 |
79.9 |
5 |
0 |
0 |
0 |
92.76 |
6 |
0 |
0 |
0 |
90.16 |
7 |
0 |
0 |
+1.68 |
91.72 |
8 |
−1 |
−1 |
−1 |
31.06 |
9 |
+1 |
+1 |
−1 |
78.55 |
10 |
0 |
0 |
0 |
92.88 |
11 |
0 |
0 |
−1.68 |
62.12 |
12 |
−1 |
+1 |
−1 |
59.23 |
13 |
+1 |
−1 |
−1 |
61.66 |
14 |
−1 |
+1 |
+1 |
81.68 |
15 |
0 |
−1.68 |
0 |
49.44 |
16 |
−1.68 |
0 |
0 |
41.51 |
17 |
+1 |
+1 |
+1 |
93.59 |
18 |
0 |
+1.68 |
0 |
96.15 |
19 |
+1 |
−1 |
+1 |
74.49 |
20 |
0 |
0 |
0 |
90.86 |
The analysis of variance (ANOVA) performed on the model can give valuable information on the significance of fitted model and its terms. From the ANOVA, as shown in Table 4, p-values of model and lack of fit are lower and higher than 0.05, respectively which means that, fitted model is significant in confidence level of 95% and it does not require to reduce to lower orders. Also, the amounts of R-squared and adj R-squared are above 0.9 and close to each other which indicates fitted model has high accuracy and reliability in prediction of the reaction yield. In other words, there is a good agreement between experimental and the predicted responses which confirms the suitability of the following fitted model in terms of significantly linear, quadratic and interaction terms on the basis of their p-values.
Y = −617.31 + 72.77X1 + 229.18X2 + 9.20X3 − 4.08X1X2 − 2.88X12 − 45.42X22 − 0.23X32 |
Table 4 The results of ANOVA in CCD for the synthesis of methyl phenyl sulfoxide
Source |
Sum of squares |
df |
Mean square |
F value |
p-Value prob > F |
Model |
7822.81 |
9 |
869.20 |
190.93 |
<0.0001 |
X1: catalyst amount |
1716.86 |
1 |
1716.86 |
377.13 |
<0.0001 |
X2: H2O2 |
2284.60 |
1 |
2284.60 |
501.84 |
<0.0001 |
X3: time |
998.95 |
1 |
998.95 |
219.43 |
<0.0001 |
X1X2 |
85.15 |
1 |
85.15 |
18.70 |
0.0015 |
X1X3 |
15.90 |
1 |
15.90 |
3.49 |
0.0911 |
X2X3 |
7.92 |
1 |
7.92 |
1.74 |
0.2166 |
X12 |
1919.99 |
1 |
1919.99 |
421.75 |
<0.0001 |
X22 |
761.26 |
1 |
761.26 |
167.22 |
<0.0001 |
X32 |
486.40 |
1 |
486.40 |
106.84 |
<0.0001 |
Residual |
45.52 |
10 |
4.55 |
|
|
Lack of fit |
36.93 |
5 |
7.39 |
4.30 |
0.0677 |
Pure error |
8.59 |
5 |
1.72 |
|
|
Cor total |
7868.33 |
19 |
|
|
|
R2 = 0.99 |
Adj-R2 = 0.98 |
Pred-R2 = 0.96 |
|
|
|
It can be realized from response equation that the X2 and X1 have a linear effect on the product yield, so the amount of H2O2 and the catalyst can be considered as the main factors in progressing of the reaction.
In order to investigate the main interaction effects between two parameters on the yield of reaction, three and two-dimensional profiles of yield versus a pair of parameters were applied (Fig. 6).
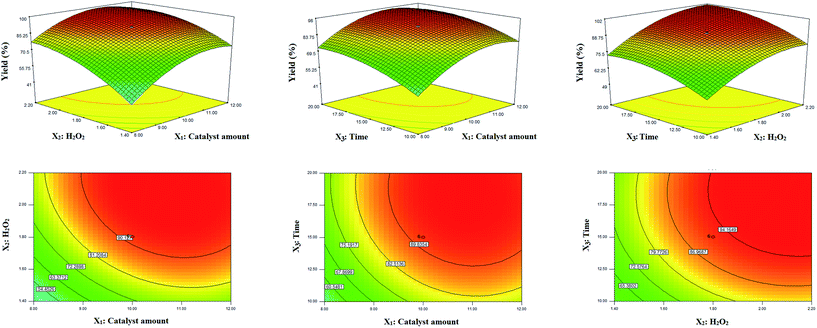 |
| Fig. 6 Three and two dimensional response surfaces for the effect of factors on the reaction yield. | |
According to their p-values, interaction of X1X2 is significant; it means that simultaneous increment of the catalyst amount and H2O2 concentration cause to increase the product yield until 10 mg of the catalyst and 2 equivalent of H2O2.
The main goal of this design was to optimize and maximize the yield of the reaction corresponded to the conditions of an experiment in which the response equation was maximized. In this work, determination of optimal conditions was done with the aid of desirability function by using Design-Expert 7.0.0. It was firstly selected desired goals for each factor and for response to obtain the maximum product yield with high desirability function (close to one). Then, conditions possessing high desirability were tested three times. Negligible difference between the average yields and the prediction values of software, confirms the high accuracy and precision of optimum conditions. The results showed that 10 mg of the catalyst, 2 equivalent of H2O2, and 15 min reaction time were the optimum conditions for the synthesis of methyl phenyl sulfoxide.
To compare the efficiency of the solvent-free versus solvent conditions, the model reaction was performed under optimum condition in the presence of EtOH, H2O, CH3CN and phCH3. The results showed that higher yield and shorter reaction time were obtained when the reaction was carried out under solvent-free condition.
These results prompted us to investigate the scope and generality of this protocol for various sulfides (aliphatic and aromatic) under the optimized conditions.
As shown in Table 5, a variety of sulfides such as dialkyl sulfides (Table 5, entries 1–5), alkyl aryl sulfides (Table 5, entries 6–9), and other sulfides with different functional groups (Table 5, entries 10–15) could be smoothly converted to corresponding sulfoxides with high conversion rate and excellent selectivity and no overoxidation products such as sulfones were detected in the reaction mixtures. Moreover, the influence of steric effects could be observed in this catalytic system. For example, the oxidation of diphenyl sulfide required much longer reaction time in comparison to isopropyl phenyl sulfide and its product yield was much lower too (Table 5, entries 9 and 12). The structures of some of the products were well characterized by using 1H NMR spectral data.
Table 5 Oxidation of sulfides to sulfoxides in the presence of nano-TiO2-NHSO3Ha
Entry |
Sulfide |
Sulfoxide |
Time (min) |
Yieldb (%) |
Selectivity (%) |
Reaction condition: sulfide (1 mmol), n-TiO2-NHSO3H (10 mg), H2O2 (2 eq.), solvent-free, at room temperature. Isolated yields. The starting material was prepared according to ref. 41. Tow diastereoisomers were separated. |
1 |
 |
 |
23 |
92 |
100 |
2 |
 |
 |
15 |
90 |
100 |
3 |
 |
 |
10 |
98 |
100 |
4 |
 |
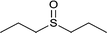 |
13 |
95 |
100 |
5 |
 |
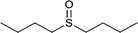 |
8 |
98 |
100 |
6 |
 |
 |
15 |
98 |
100 |
7 |
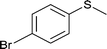 |
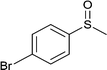 |
25 |
93 |
100 |
8 |
 |
 |
10 |
97 |
100 |
9 |
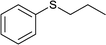 |
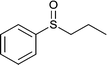 |
10 |
97 |
100 |
10 |
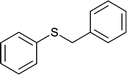 |
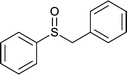 |
30 |
85 |
100 |
11 |
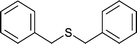 |
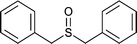 |
25 |
95 |
100 |
12 |
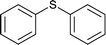 |
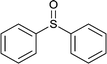 |
60 |
80 |
100 |
13 |
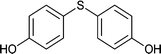 |
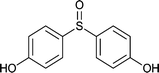 |
10 |
95 |
100 |
14 |
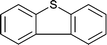 |
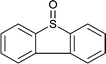 |
30 |
93 |
100 |
15c |
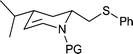 |
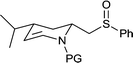 |
65 |
90d |
100 |
There are two proposed mechanisms for the oxidation of sulfides using hydrogen peroxide in the presence of an acidic catalyst which are depicted in Scheme 3.42 One explanation for this process is the in situ formation of peroxyacid by the reaction of catalyst with hydrogen peroxide, followed by the oxygen transfer to the organic substrate (Scheme 3a). Another explanation is that catalyst might act as a protic acid that acid interaction or hydrogen bonding of sulfonic acid with hydrogen peroxide polarizes the O–O bond in H2O2 to produce the reactive oxygen transfer agent.43 At the same time, the deporting of water as a leaving group would be assisted by nucleophilic attack of sulfide (Scheme 3b).
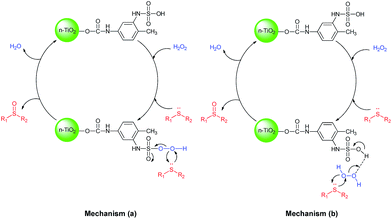 |
| Scheme 3 Proposed mechanisms for the oxidation of sulfides using H2O2 catalyzed by n-TiO2-NHSO3H. | |
The chemoselectivity of the reaction may be controlled by hydrogen bonding, since the hydrogen bonds between the acidic catalyst and the oxygen of the sulfoxides could decrease the nucleophilicity of the sulfur atom in the sulfoxides and prevent overoxidation of the sulfoxide to a sulfone.44
Encouraged by these results and in order to generalize the catalytic efficiency of n-TiO2-NHSO3H, our study was followed by the oxidative coupling of thiols into their corresponding disulfides under the optimized condition reaction of sulfoxides (Scheme 4).
 |
| Scheme 4 Nano-TiO2-NHSO3H catalyzed oxidative coupling of thiols to disulfides using H2O2. | |
The generality of this approach has been demonstrated in Table 6. As shown, a variety of thiols bearing aliphatic thiols (Table 6, entries 1 and 2), aromatic thiols (Table 6, entries 3–6), and other thiols with different functional groups were successfully employed to prepare the corresponding disulfides in short reaction time and excellent yields. Moreover, in all the reactions no any byproducts was observed.
Table 6 Oxidative coupling of thiols into disulfides in the presence of nano-TiO2-NHSO3Ha
Entry |
Thiol |
Disulfide |
Time (min) |
Yieldb (%) |
Reaction condition: thiols (1 mmol), n-TiO2-NHSO3H (10 mg), H2O2 (2 eq.), solvent-free, at room temperature. Isolated yields. |
1 |
 |
 |
5 |
97 |
2 |
 |
 |
10 |
98 |
3 |
 |
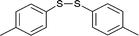 |
7 |
95 |
4 |
 |
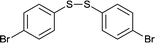 |
15 |
97 |
5 |
 |
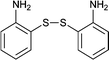 |
15 |
93 |
6 |
 |
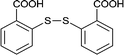 |
17 |
90 |
7 |
 |
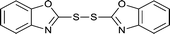 |
20 |
90 |
8 |
 |
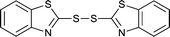 |
25 |
89 |
The suggested mechanism for the oxidative coupling of thiols is illustrated in Scheme 5.22 Combination of hydrogen peroxide with catalyst would generate OH radical which would subsequently react with thiol to produce thiol radical, followed by dimerization to afford corresponding disulfide.
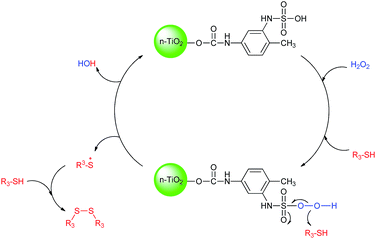 |
| Scheme 5 Proposed mechanism for the oxidative coupling of thiols using H2O2 catalyzed by n-TiO2-NHSO3H. | |
2.3. Recycling and leaching of the catalyst
Reusability is one of the important criteria for an efficient heterogeneous catalyst in organic transformations and makes it useful for commercial applications. The synthesized catalyst is capable to run the oxidation reaction after 10 runs efficiently and all reactions were led to the desired product without significant changes in terms of the reaction time and yield which clearly demonstrates practical recyclability of n-TiO2-NHSO3H (Fig. 7). In addition, the weight of the recovered catalyst was the same as that of the fresh catalyst used for the first time in the reaction.
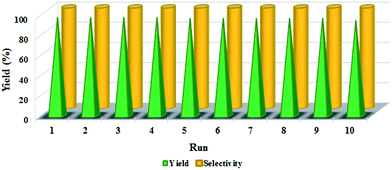 |
| Fig. 7 Reusability of n-TiO2-NHSO3H for the synthesis of methyl phenyl sulfoxide; reaction conditions: methyl phenyl sulfide (1 mmol), H2O2 (2 eq.), catalyst (10 mg), reaction time: 15 min at room temperature under solvent-free condition. | |
Conversion as a function of reaction time for the recycling of n-TiO2-NHSO3H is illustrated in Fig. 8. As can be seen from the kinetic curves, initial rates for the ten successive recycling runs are similar and reaction rates are nearly constant and no further significant loss of activity is detected.
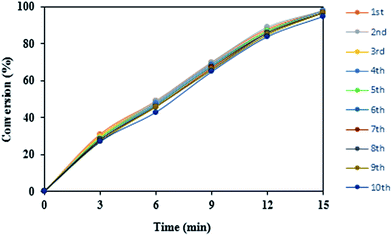 |
| Fig. 8 Plot of conversion vs. reaction time for the recycled n-TiO2-NHSO3H in the synthesis of methyl phenyl sulfoxide; reaction conditions: methyl phenyl sulfide (1 mmol), H2O2 (2 eq.), catalyst (10 mg), reaction time: 15 min at room temperature under solvent-free condition. | |
The identity of the recovered catalyst was checked by FE-SEM which suggested that the nature of the catalyst remains intact and there was no change in the morphology during the reaction and recycling stages as compared to the fresh catalyst (Fig. 9).
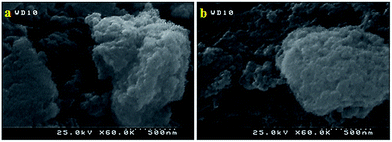 |
| Fig. 9 FE-SEM images of n-TiO2-NHSO3H before use (a) and after reused ten times (b). | |
Additionally, the FT-IR spectrum of the catalyst after ten cycles are identical to the corresponding spectrum before catalytic reaction which confirmed the existence of acidic groups on the surface of catalyst (Fig. 10).
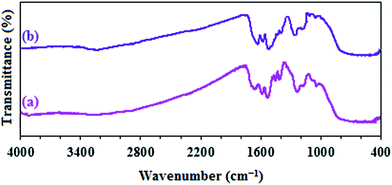 |
| Fig. 10 FT-IR spectra of n-TiO2-NHSO3H before use (a) and after reused ten times (b). | |
To explore the catalyst leaching in the oxidation of ethyl phenyl sulfide, the reaction was stopped at half the reaction time (5 min) and the solid catalyst was completely separated from the solution by centrifuge. Then, the solution was kept back for oxidation under the same conditions. As can be seen in Fig. 11, no appreciable progress and increase in the product yield was observed, even after 20 min (monitored by TLC). These results confirm that the reaction is attributed to the heterogeneous catalyst and catalyst leaching is negligible.
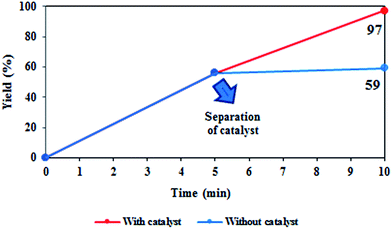 |
| Fig. 11 Leaching experiment; reaction conditions: ethyl phenyl sulfide (1 mmol), H2O2 (2 eq.), catalyst (10 mg), at room temperature under solvent-free condition. | |
3. Experimental
3.1. Materials and instruments
All chemicals were used without any further purification and purchased from the Merck and Aldrich chemical companies. Toluene diisocyanate (TDI) was in industrial grade with 80
:
20 mixture of 2,4 and 2,6 isomers and it was used as received. Solvents were purified by conventional methods. The purity of products was checked by thin layer chromatography (TLC) on commercial plates coated with silica gel 60 F254 using n-hexane/ethyl acetate mixture as mobile phase. Melting points were recorded on THERMO SCIENTIFIC 9100 apparatus. Fourier transform infrared spectroscopy (FT-IR) were recorded on a Shimadzu 8400 spectrometer in the range of 400–4000 cm−1 using KBr pressed powder discs. Field emission scanning electron microscope (FE-SEM) images were acquired with a Philips XL30 field emission scanning electron microscope (Royal Philips Electronics, Amsterdam, The Netherlands) instrument operating at 25 kV. Particles were mounted on a double sided adhesive carbon disk and sputter-coated with a thin layer of gold to prevent sample charging problems. Wide-angle X-ray diffraction (XRD) measurements were performed at room temperature on a Siemens D5000 (Siemens AG, Munich, Germany) using Cu-Kα radiation of wavelength 1.54 Å. Thermogravimetric analyses (TGA) were conducted using a Du Pont 2000 thermal analysis apparatus heated from 25 °C to 800 °C at ramp 5 °C min−1 under air atmosphere. The NMR spectra were measured in pure deuterated chloroform with a Bruker Advance 400 MHz instruments (1H NMR 400 MHz) with tetramethylsilane (TMS) as the internal reference. The absorption spectra in the UV-visible regions were recorded by a UV-1650 PC-UV-Vis spectrophotometer (Shimadzu, Japan).
3.2. Preparation of nano-TiO2
The nano-TiO2 was synthesized by hydrothermal method according to the previously reported procedure.27 Briefly, NH3·H2O was added to TiCl4 solution until the pH value become 1.8. After stirring for 2 h at 70 °C, the final pH of the solution was adjusted to 6. The resulting suspension was aged at ambient temperature for 24 h. The final product was filtered, washed with NH4Ac-HOAc until no Cl− was detected. Then, the precipitate was separated using a centrifuge and was washed with ethanol, dried in a vacuum. After 2 h treatment at 650 °C, TiO2 nanoparticles were obtained.
3.3. Functionalization of TiO2 nanoparticle with TDI (n-TiO2-NCO)
The n-TiO2-NCO was prepared following the reported procedure.36 A mixture of 1.0 g TiO2 nanoparticle and 1.40 g TDI was dispersed in 50 mL dried toluene and placed in an ultrasonic bath for 10–15 min. Then the reaction mixture was maintained at the temperature of 95 °C by a thermostat and stirred for 6 h under the atmosphere of nitrogen. The powder product was separated by centrifugation and then carefully washed with dry toluene to remove the unreacted and physical absorbed TDI. The product, was dried in vacuum at 80 °C for 24 h.
3.4. Preparation of amine-functionalized n-TiO2 (n-TiO2-NH2)
A round bottom flask of 100 mL capacity was charged with 1.0 g (n-TiO2-TDI) and 50 mL of H2O–acetone mixture at mass ratio of 5/5. The flask was immersed in a water bath at 30 °C, under stirring. The reaction was allowed to continue for 2 h, followed by centrifugation to separate the solid, which was rinsed twice by acetone and dried at 110 °C under vacuum to get a powder product.
3.5. Preparation of sulfamic acid-functionalized n-TiO2 (n-TiO2-NHSO3H)
Nano-TiO2-NH2 was modified using a suction flask equipped with a constant pressure dropping funnel containing 0.25 mL chlorosulfonic acid (3.75 mmol) and a gas inlet tube for conducting HCl gas over an adsorbing solution such as water. The flask was charged with 1.0 g n-TiO2-NH2 in 10 mL dry CH2Cl2 and chlorosulfonic acid was then added drop wisely over a period of 30 min at room temperature. Upon completion of the addition, the mixture was stirred for a further 1 h to allow for the complete dissipation of HCl from the reaction vessel. Then, the CH2Cl2 was removed under reduced pressure and the solid powder was washed twice with ethanol (10 mL) and dried at 70 °C for 4 h.
3.6. General procedure for the catalytic oxidation of sulfides
To a mixture of the sulfide (1 mmol) and 30% H2O2 (2 eq.), the catalyst (10 mg) was added and the mixture was stirred at room temperature for a specified time. The progress of the reaction was monitored by TLC (n-hexane/EtOAc, 9/1). After completion of the reaction, the reaction mixture was eluted with Et2O (10 mL) and centrifuged to filter the catalyst. The combined organic layers were dried over anhydrous Na2SO4 and evaporation of diethyl ether under reduced pressure gave the pure products in 80–98% yields.
3.7. General procedure for the catalytic oxidative coupling of thiols
To a mixture of thiol (1 mmol) and 30% H2O2 (2 eq.), the catalyst (10 mg) was added and the mixture was stirred at room temperature for a specified time. The progress of the reaction was monitored by TLC (n-hexane/EtOAc, 8/2). After completion of the reaction, the reaction mixture was eluted with CH2Cl2 (10 mL) and centrifuged to filter the catalyst. The combined organic layers were dried over anhydrous Na2SO4 and evaporation of CH2Cl2 under reduced pressure gave the pure products in high yields.
3.8. General procedure for recycling of n-TiO2-NHSO3H
In order to investigate the recoverability and recyclability of catalyst, the oxidation of methyl phenyl sulfide (1 mmol) under the optimized conditions was chosen as model reaction. Upon completion of the reaction, the catalyst was separated via centrifuge, washed with ether (2 × 5 mL) and acetone (2 × 5 mL) dried at 70 °C for 1 h to be used in the next cycles.
4. Conclusions
In conclusion, for the first time, we described here a new class of inorganic–organic hybrid nanocatalyst based on toluene 2,4-toluene diisocyanate as a linker for potential synthetic application. This efficient and environmentally friendly Brønsted acidic catalyst was used successfully for the chemoselective oxidation of sulfides and thiols. Operational simplicity, low cost and non-toxic nature, excellent chemoselectivity, applicability to large-scale reactions, high yields of products, and high recyclability are the main superiorities of our new catalyst, which make it as a promising catalyst for technical, industrial and organic applications. Studies toward further applications of this catalyst for other organic transformations and also exploring the powerful potential of using TDI as immobilization strategy for the preparation of recoverable heterogeneous nanocatalysts are underway in our laboratory.
Acknowledgements
We gratefully acknowledge the Faculty of chemistry of Semnan University for supporting this work.
References
- A. Corma and H. Garcia, Adv. Synth. Catal., 2006, 348, 1391–1412 CrossRef CAS.
- M. A. Zolfigol, Tetrahedron, 2001, 57, 9509–9511 CrossRef CAS.
- R. I. Kureshy, I. Ahmad, K. Pathak, N. H. Khan, S. H. R. Abdi and R. V. Jasra, Catal. Commun., 2009, 10, 572–575 CrossRef CAS.
- F. Shirini, M. Mamaghani and S. V. Atghia, Catal. Commun., 2011, 12, 1088–1094 CrossRef CAS.
- S. Rostamnia, H. Xin, X. Liu and K. Lamei, J. Mol. Catal. A: Chem., 2013, 374–375, 85–93 CrossRef CAS.
- S. V. Atghia and S. Sarvi Beigbaghlou, J. Organomet. Chem., 2013, 745–746, 42–49 CrossRef CAS.
- X.-N. Zhao, G.-F. Hu, M. Tang, T.-T. Shi, X.-L. Guo, T.-T. Li and Z.-H. Zhang, RSC Adv., 2014, 4, 51089–51097 RSC.
- P. Gholamzadeh, G. Mohammadi Ziarani, N. Lashgari, A. Badiei and P. Asadiatouei, J. Mol. Catal. A: Chem., 2014, 391, 208–222 CrossRef CAS.
- H. Jin, M. B. Ansari and S.-E. Park, Catal. Today, 2015, 245, 116–121 CrossRef CAS.
- R. H. Vekariya and H. D. Patel, RSC Adv., 2015, 5, 49006–49030 RSC.
- G. Mohammadi Ziarani, N. Lashgari and A. Badiei, J. Mol. Catal. A: Chem., 2015, 397, 166–191 CrossRef CAS.
- K. Tanabe and W. F. Hölderich, Appl. Catal., A, 1999, 181, 399–434 CrossRef CAS.
- J. S. Yadav, B. V. S. Reddy, K. Sadashiv, S. Raghavendra and K. Harikishan, Catal. Commun., 2004, 5, 111–114 CrossRef CAS.
- O. G. da Silva, E. C. da Silva Filho, M. G. da Fonseca, L. N. H. Arakaki and C. Airoldi, J. Colloid Interface Sci., 2006, 302, 485–491 CrossRef CAS PubMed.
- M. C. Carreno, Chem. Rev., 1995, 95, 1717–1760 CrossRef CAS.
- R. Fernández de la Pradilla, I. Colomer and A. Viso, Org. Lett., 2012, 14, 3068–3071 CrossRef PubMed.
- S. Thurow, V. A. Pereira, D. M. Martinez, D. Alves, G. Perin, R. G. Jacob and E. J. Lenardão, Tetrahedron Lett., 2011, 52, 640–643 CrossRef CAS.
- M. Hajjami and S. Rahmani, J. Porous Mater., 2015, 22, 1265–1274 CrossRef CAS.
- B. Yu, A.-H. Liu, L.-N. He, B. Li, Z.-F. Diao and Y.-N. Li, Green Chem., 2012, 14, 957–962 RSC.
- S. Kumar, S. Verma, S. L. Jain and B. Sain, Tetrahedron Lett., 2011, 52, 3393–3396 CrossRef CAS.
- M. Nikoorazm, A. Ghorbani-Choghamarani, H. Mahdavi and S. M. Esmaeili, Microporous Mesoporous Mater., 2015, 211, 174–181 CrossRef CAS.
- A. Ghorbani-Choghamarani, B. Tahmasbi, F. Arghand and S. Faryadi, RSC Adv., 2015, 5, 92174–92183 RSC.
- S. Rahmani, A. Amoozadeh and E. Kolvari, Catal. Commun., 2014, 56, 184–188 CrossRef CAS.
- A. Amoozadeh and S. Rahmani, J. Mol. Catal. A: Chem., 2015, 396, 96–107 CrossRef CAS.
- A. Amoozadeh, S. Golian and S. Rahmani, RSC Adv., 2015, 5, 45974–45982 RSC.
- S. Otokesh, E. Kolvari, A. Amoozadeh and N. Koukabi, RSC Adv., 2015, 5, 53749–53756 RSC.
- J. Chen, M. Liu, L. Zhang, J. Zhang and L. Jin, Water Res., 2003, 37, 3815–3820 CrossRef CAS PubMed.
- H. Xia and M. Song, J. Mater. Chem., 2006, 16, 1843–1851 RSC.
- Y. S. Chun, K. Ha, Y.-J. Lee, J. S. Lee, H. S. Kim, Y. S. Park and K. B. Yoon, Chem. Commun., 2002, 1846–1847, 10.1039/b205046c.
- S. Li and Y. Liu, Polyurethane Adhesive, Chemical Industry Press, Beijing, China, 1998, pp. 13–20 Search PubMed.
- S. Zhang, J. Zhou, Z. Zhang, Z. Du, A. V. Vorontsov and Z. Jin, Chin. Sci. Bull., 2000, 45, 1533–1536 CrossRef CAS.
- F. Solymosi and J. Raskó, J. Catal., 1980, 63, 217–225 CrossRef CAS.
- H. R. Li, J. Lin, H. J. Zhang, L. S. Fu, Q. G. Meng and S. B. Wang, Chem. Mater., 2002, 14, 3651–3655 CrossRef CAS.
- B. D. Cullity and S. R. Stock, Elements of X-ray Diffraction, Prentice-Hall, Englewood Cliffs, 3rd edn, 2001 Search PubMed.
- S. Atghia and S. Beigbaghlou, J. Nanostruct. Chem., 2013, 3, 38 CrossRef.
- B. Ou, D. Li, Q. Liu, Z. Zhou and B. Liao, Mater. Chem. Phys., 2012, 135, 1104–1107 CrossRef CAS.
- H. Xing, T. Wang, Z. Zhou and Y. Dai, J. Mol. Catal. A: Chem., 2007, 264, 53–59 CrossRef CAS.
- K. A. Stingl and S. B. Tsogoeva, Tetrahedron: Asymmetry, 2010, 21, 1055–1074 CrossRef CAS.
- I. F. A. N. Khiar, Chem. Rev., 2003, 103, 3651–3706 CrossRef PubMed.
- H. B. Kagan and P. Diter, Organosulfur Chemistry, Academic Press, 1998, vol. 2, pp. 1–39 Search PubMed.
- D. Gravel, A. Amoozadeh and Y. Wang, Tetrahedron Lett., 1998, 39, 8039–8042 CrossRef CAS.
- A. Rostami, B. Tahmasbi, F. Abedi and Z. Shokri, J. Mol. Catal. A: Chem., 2013, 378, 200–205 CrossRef CAS.
- S. Verma, R. Singh, D. Tripathi, P. Gupta, G. M. Bahuguna and S. L. Jain, RSC Adv., 2013, 3, 4184–4188 RSC.
- K. Bahrami, M. M. Khodaei and P. Fattahpour, Catal. Sci. Technol., 2011, 1, 389–393 CAS.
Footnote |
† Electronic supplementary information (ESI) available: 1HNMR spectra of sulfoxides. See DOI: 10.1039/c5ra20507g |
|
This journal is © The Royal Society of Chemistry 2016 |