DOI:
10.1039/D4QI01040J
(Research Article)
Inorg. Chem. Front., 2024,
11, 3744-3754
RbPbPS4: a promising IR nonlinear optical material achieved by lone-pair-cation-substitution-induced structure transformation†
Received
25th April 2024
, Accepted 18th May 2024
First published on 20th May 2024
Abstract
When stereochemically-active-lone-pair (SCALP) cations are introduced into chalcogenide systems, it is beneficial to produce a remarkable second-harmonic generation (SHG) response (deff), but it also causes a narrow band gap (Eg), which ultimately results in negative two-photon and free-carrier absorption. Hence, how to obtain a wide Eg (>2.33 eV) while maintaining a strong deff remains a significant challenge in this domain. In this work, Rb is partially replaced by SCALP Pb in the known ternary centrosymmetric (CS) Rb3PS4 (space group: Pnma). This results in a quaternary non-centrosymmetric (NCS) RbPbPS4 (space group: P212121), which possesses distinct 2D [PbPS4]− layers and Rb+ occupies the interlayer spaces as the counter cations. Notably, RbPbPS4 exhibits a promising overall performance, including strong deff (2.5 × AgGaS2), wide IR transmittance cutoff edge (up to 18.1 μm) along with the large Eg (2.75 eV), resulting in an improved laser-induced damage threshold (7.5 × AgGaS2). Theoretical calculations further indicated that the favorable balance between strong deff and large Eg in RbPbPS4 can be attributed to the synergies of the [PbS7] and [PS4] nonlinear optical (NLO)-active units. This study not only presents a high-performance Pb-based IR-NLO candidate but also underscores the effectiveness of partial substitution of SCALP cations in inducing a CS-to-NCS structural transformation.
Introduction
Nonlinear optical (NLO) materials have garnered unprecedented attention in laser science and technology because of their ability to facilitate frequency conversion in solid-state laser devices.1 As widely recognized, an excellent NLO candidate should meet several crucial prerequisites: a sufficient second-harmonic generation (SHG) intensity (deff), a large energy gap (Eg), a wide optical transparent window, a moderate birefringence (Δn), chemical stability, and availability for obtaining large single crystals.2 In the infrared (IR) region, numerous vital fields, including optoelectronic instruments, resource exploration, and remote laser communication, has sparked widespread attention and interest. Despite the strong deff exhibited by commercial IR-NLO crystals such as AgGaQ2 (Q = S, Se)3 and ZnGeP2,4 which make them suitable for applications in the IR region, they still suffer from limitations in high-power laser systems due to their low laser-induced damage thresholds (LIDT) or detrimental two-photon absorption, primarily attributed to their small Eg. However, integrating these optical performances into a single crystal is extremely challenging because they typically depend on competing structural requirements, such as the trade-off between wide Eg and strong deff.5 Therefore, it is of scientific and technological significance to explore new IR-NLO crystals with outstanding comprehensive performance to overcome these challenges.
In addition to the performance prerequisites mentioned above, a prerequisite of an IR-NLO crystal is that it has a non-centrosymmetric (NCS) structure.6 The addition of stereochemically-active-lone-pair (SCALP) cations, like As3+, Sb3+, Bi3+, Sn2+, and Pb2+, to chalcogenide systems has attracted the most attention among all the feasible approaches for creating IR-NLO materials because the majority of these chalcogenides exhibit exceptional IR-NLO performances.7 Among these cations, Pb2+ makes a significant contribution to the deff but adversely affects the optical Eg.8 As is known, narrow energy gaps (Eg < 2.33 eV) are unable to mitigate some harmful two-photon or free-carrier absorption under fundamental 1064 nm laser sources.9 Therefore, achieving a wide Eg (>2.33 eV) while maintaining a strong deff remains a significant challenge in Pb-based IR-NLO material design. On the other hand, partial chemical substitution in view of known centrosymmetric (CS) parent structures has shown to be a straightforward but incredibly successful approach in recent years for the design and synthesis of novel high-performance SCALP-based IR-NLO crystals.10 Successful examples include NCS Ba2As2Se5 (parent structure: CS Ba2GaAsSe5),11 NCS K2Ag3Sb3S7 (parent structure: CS K2Sb4S7),12 NCS ABiP2S6 (A = K, Rb) (parent structure: CS A4P2S6),13,14 NCS Sn7Br10S2 (parent structure: CS SnBr2),15 Sr2−xPbxGeSe4 (parent structure: NCS Sr2GeSe4),16 and NCS Pb4SeBr6 (parent structure: CS PbBr2).17 Considering these factors, our focus lies in identifying suitable CS parent structures with wide Eg and achieving CS-to-NCS structural transformation by introducing SCALP Pb for partial chemical substitution. This approach aims to obtain NCS materials with excellent, well-balanced IR-NLO performance.
The ternary chalcophosphate Rb3PS4 has piqued our interest due to its CS space group of Pmna and unique zero-dimensional (0D) cluster structure.18 By incorporating distorted Pb-based motifs, it is possible to disrupt symmetric centers, leading to structural reconstruction and the formation of NCS compounds. Furthermore, Rb3PS4 boasts a sufficiently large Eg (>3.5 eV), which can help offset the potential decrease in Eg resulting from the introduction of SCALP Pb2+ cations into new compounds. Following this approach, we have designed and synthesized the quaternary Pb-based thiophosphate RbPbPS4, featuring a two-dimensional (2D) layered structure composed of [PbS7] polyhedra and [PS4] tetrahedra. Remarkably, RbPbPS4 demonstrates comprehensive IR-NLO performance, including a strong deff (3.2 times that of AgGaS2), one of the widest Eg among Pb-based NCS chalcogenides, high LIDT (7.5 times that of AgGaS2), a broad transmittance range (up to 18.1 μm), and suitable Δn (0.112@2050 nm). In this report, we provide detailed insights into the CS-to-NCS structural transformation and the related optical properties of RbPbPS4. Additionally, theoretical calculations are employed to further elucidate its linear optical and NLO performances.
Results and discussion
There are reports of RbPbPS4's crystal structure exhibiting two distinct phases.19 However, we meticulously determined the structure of the title compound to explore the intricate relationship between its NCS crystal structure and NLO properties. Light yellow lamellar crystals of RbPbPS4, with sizes reaching the millimeter level, were grown using a simple boron–chalcogen method.20 This method differs from previously reported techniques, which often necessitate complex experimental procedures or involve the use of costly and hazardous elemental Rb as a raw material. Elemental distribution maps indicate that Rb, Pb, P, and S are uniformly distributed throughout the crystals (Fig. 1a). Furthermore, EDX results confirmed that the average molar ratios of the title compound correspond to the formula determined by single-crystal XRD analysis (Fig. S1†). Powder XRD testing validated the phase purity of the title compound, as the experimental patterns closely matched the simulated data obtained from single-crystal XRD analyses (Fig. 1b). The UV-vis diffuse reflectance spectrum of RbPbPS4 is depicted in Fig. 1c, and absorption data were derived using the Kubelka–Munk equation.21 With an experimental optical Eg of 2.75 eV, RbPbPS4 exhibits the second-highest recorded value among Pb-based IR-NLO chalcogenides. Additionally, RbPbPS4 demonstrates broad optical transparency across the 0.38–18.1 μm range (Fig. 1d). Thermogravimetric-differential thermal analysis (TG-DTA) was employed to assess the thermal stability of RbPbPS4, revealing that the compound remains stable below 700 K (Fig. 1e).
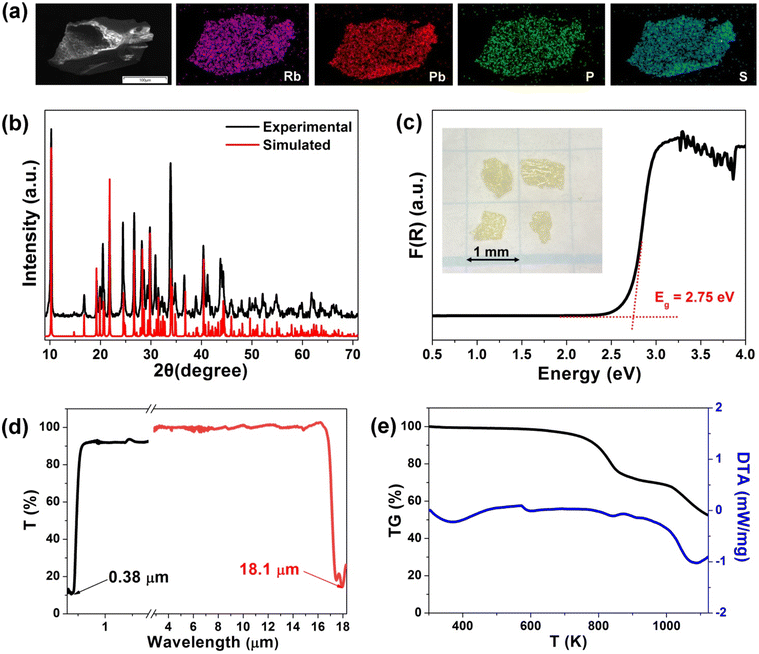 |
| Fig. 1 Experimental characterization results of PbPbPS4: (a) SEM image and corresponding elemental distribution maps; (b) experimental (black) and simulated (red) powder XRD patterns; (c) UV–vis–NIR spectrum (inset: photograph of the title crystals); (d) optical transmittance spectrum; (e) TG-DTA test curves. | |
In the asymmetric unit, ternary Rb3PS4 has two independent Rb atoms (Wyckoff positions: 4c and 8d), one independent P atom (Wyckoff position: 4c), and two independent S atoms (Wyckoff positions: 4c and 8d). The P5+ cation is located in the center of its common tetrahedron, with P–S bond lengthes ranging from 2.043 to 2.055 Å and the bond angles in the range of 108.82–111.48°. Ternary Rb3PS4 belongs to the orthorhombic system [space group: Pnma (no. 62)] and the crystal structure consists of counterbalanced Rb+ cations situated in between discrete tetrahedral [PS4] basic building units (BBUs) aligned parallel in opposing orientations (Fig. 2a). Unfortunately, Rb3PS4 lacks NLO activity due to its CS crystal structure.
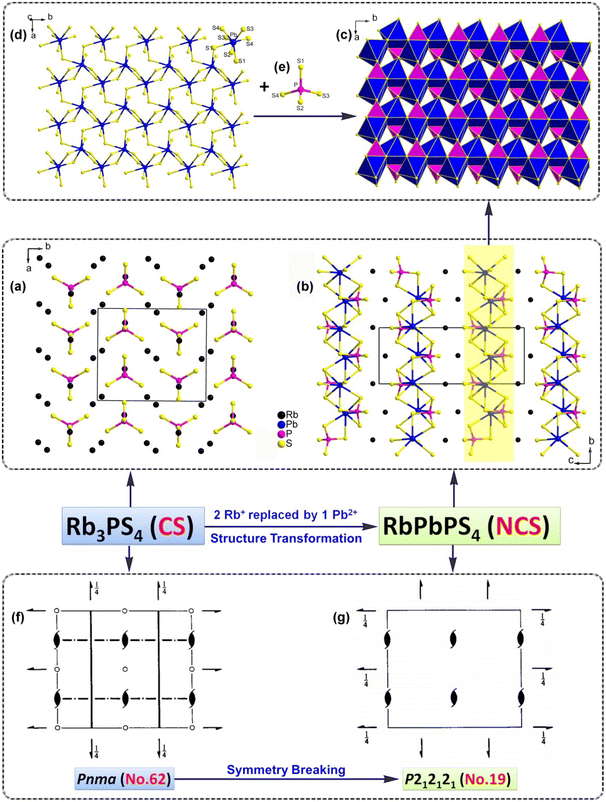 |
| Fig. 2 Structure evolution from ternary CS Rb3PS4 to quaternary NCS RbPbPS4: view of the 2D layered structures of (a) Rb3PS4 and (b) RbPbPS4 along the ab and bc plane, respectively; (c) a polyhedral 2D layer [PbPS4]− composed of (d) 2D Pb–S layer and (e) discrete tetrahedral [PS4] motifs; (f and g) spatial symmetry operation changing from CS Pmna (no. 62) to NCS P212121 (no. 19). | |
Quaternary RbPbPS4 adopts the NCS orthorhombic space group P212121 (no. 19, Table S1†), according to single-crystal XRD investigation. The cell lattice possesses dimensions of a = 6.3981(2) Å, b = 6.6888(2) Å, c = 17.2823(5) Å, and V = 739.61(4) Å3. These crystal parameters are in good agreement with the NCS phase that was previously published. The compound has a 2D layered structure with discrete counterbalanced Rb+ cations inserted in the channels that are arranged in an “ABABAB” mode perpendicular to the ab-plane (Fig. 2b and c). With seven S atoms polyhedral coordinating each Pb atom in the structure, the highly distorted [PbS7] BBUs with Pb–S bond lengths of 2.916–3.281 Å are formed. As shown in Fig. 2d, adjacent [PbS7] BBUs are joined by apex-sharing S atoms to generate a 2D Pb–S layer. While the P–S bonds in RbPbPS4 range in length from 2.031 to 2.069 Å and in bond angles from 106.56 to 111.62°, each P atom is comparable to that of Rb3PS4 and is encircled by four S atoms, forming the deformed [PS4] tetrahedron (Table S2†). Subsequently, apex-sharing between these 2D Pb–S layers (Fig. 2d) and distinct [PS4] BBUs (Fig. 2e) progressively linked them, resulting in the formation of a 2D [PbPS4]− anionic layer (Fig. 2c).
Fig. 2 shows the CS-to-NCS structural evolution via partial SCALP cation substitution from ternary CS Rb3PS4 to quaternary NCS RbPbPS4. From a structural perspective, the following noteworthy modifications have been made following the partial replacement of Rb by SCALP Pb. First off, the initial highly symmetric structure is broken by the addition of SCALP [PbS7] BBUs and the reassembly of discrete [PS4] BBUs, which convert the 0D cluster structure into a 2D layered structure. Fig. 2f and g show the detailed symmetric operation modifications that occur after the substitution of SCALP Pb2+ cations, resulting in the removal of all center sites and the realization of the CS-to-NCS structural evolution. In particular, space group Pnma (no. 62) converts to P212121 (no. 19) from the Bärnighausen tree via a translationengleiche reduction in index 2's symmetry (Fig. 3a).22 When Pb is substituted for Rb, the atomic positions (4c and 8d) in Rb3PS4 become 4a in RbPbPS4 (see Table S3† for details). To put it briefly, the novel compound that was produced, RbPbPS4, has good structural anisotropy, which helps to get a higher Δn for achieving PM feature. Secondly, the coordination number (CN) of the two crystallographically independent Rb atoms in Rb3PS4 differ (CN = 8 for Rb1 and CN = 9 for Rb2), but are identical in RbPbPS4 (CN = 11) with Rb–S bond distances less than 4.4 Å (refer to Fig. S2 and S3 in ESI†). Finally, considering the flexible CN of Pb2+ cations, the integrated crystal orbital Hamilton population (ICOHP) curve was examined.23 As depicted in Fig. 3b, it is evident that the short Pb–S bond lengthes of 2.916–3.114 Å exhibit stronger bonding interactions compared to the long Pb–S bond lengthes (3.647–3.825 Å), while the Pb–S bond distance of 3.281 Å falls between them, representing a medium interaction (see Table S2† for details). Based on the above analysis, it is reasonable to infer that the Pb atom forms a [PbS7] mono-capped triangular prism. Research on the distinct [PbS7] coordination mode in NCS chalcogenides is notably lacking, and a seven-coordinated example of this has been reported in Pb5Ga6ZnS15.8d Pb2+ cations in NCS chalcogenides are mostly five-coordinated (as in [Na2PbI][Ga7S12]8f) or six-coordinated (as in Pb4Ga4GeS12
8a and Pb0.65Mn2.85Ga3S8
8b), among other compounds that have been found.
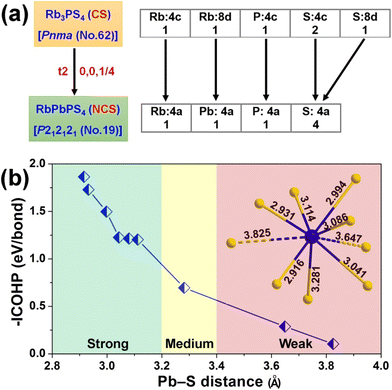 |
| Fig. 3 (a) Group–subgroup relations between CS Rb3PS4 [space group: Pmna (no. 62)] and NCS RbPbPS4 [space group: P212121 (no. 19)]; (b) crystal orbital Hamilton population (COHP) curves for the Pb–S bond length in RbPbPS4 (the inset figure is the coordination geometry of Pb atom). | |
Furthermore, through comparison with known ternary and quaternary chalcophosphates, it is determined that substituting SCALP Pb for partial alkali metal Rb is the most optimal and rational structural approach to accomplish the CS-to-NCS structural transformation of template structure Rb3PS4. Fig. S4† provides a detailed structural evolution. Roughly speaking, these replacements fall into three categories. In the first, three Rb atoms are completely substituted by one trivalent element or three monovalent elements in the absence of SCALP components. CS structures are displayed by the resultant compounds, including REPS4 (space group: I41/acd),24 BPS4 (space group: Ibam),25 GaPS4 (space group: P21/c),26 and Tl3PS4 (space group: Pnma).27 The second category entails complete substitution of SCALP elements, as seen in SbPS4 (space group: P
)28 and BiPS4 (space group: Ibca),29 which also belong to the CS structures. The third category involves partial substitution, where Rb is partially replaced by monovalent or divalent elements. The resulting compounds, such as Rb2AgPS4 (space group: P
),30 RbAg5P2S8 (space group: Pbca),31 RbSrPS4 (space group: Pnma),32 RbBaPS4 (space group: Pnma),33 RbPdPS4 (space group: I4/mcm),34 and RbHgPS4 (space group: P21/n),35 all adopt CS structures. Therefore, it is reasonable to replace partial Rb with SCALP elements to realize a structural transition from CS Rb3PS4 to NCS RbPbPS4. Hence, employing established CS chalcophosphates as the parent structure and partially substituting them with SCALP-cation groups proves to be an effective chemical strategy for accomplishing the CS-to-NCS structural evolution.
As RbPbPS4 belongs to the NCS space group P212121 and possesses the NLO-active motifs [PbS7] and [PS4], a relatively strong deff could be expected. Powder SHG testing for RbPbPS4 was conducted using the modified Kurtz–Perry technology,35 with irradiation from a 2900 nm laser and commercial material AgGaS2 as a reference. As depicted in Fig. 4a, the positive correlation between the particle size and SHG intensity indicates that RbPbPS4 exhibits PM behavior. The SHG intensity of RbPbPS4 is approximately 2.5 times that of benchmark AgGaS2 in a particle size range of 150–210 μm (Fig. 4b). Based on deff = deff,R (I2ω/IR2ω)1/2 (deff,R = 13.4 pm V−1 for AgGaS2), the effective SHG coefficient deff of RbPbPS4 at 2900 nm is 21.2 pm V−1. In addition, powder LIDT measurement was employed to preliminarily evaluate the LIDT value,36 with the increased optical Eg suggesting that the title crystal would exhibit higher LIDT. As illustrated in Fig. 4b, the LIDT of RbPbPS4 (10.88 MW cm−2) is estimated to be 7.5 times that of AgGaS2 (1.45 MW cm−2) under the same testing conditions. Compared with other reported Pb-based IR-NLO materials (Fig. 4c and Table S4†), RbPbPS4 demonstrates a favorable balance between strong deff and large Eg. Notably, RbPbPS4 represents the first quaternary Pb-based chalcogenide to overcome the incompatibility between a wide Eg (>2.56 eV, the Eg of AgGaS2) and a sufficient deff (>1.0 × AgGaS2). This balance IR-NLO performance can be compared to the recently reported quaternary NCS chalcogenides, such as α-Li2ZnGeS4,37 Li4CdSn2S7,38 NaMg3Ga3S8,39 and (CuI)3P4S4.40
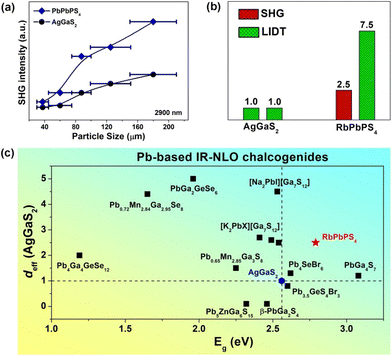 |
| Fig. 4 (a) SHG intensities vs. particle size at λ = 2900 nm for PbPbPS4 and AgGaS2; (b) the relative SHG and LIDT intensities of PbPbPS4 and reference commercial material AgGaS2 in the particle size of 150–210 μm; (c) comparison of deff (×AgGaS2) and Eg (eV) of reported Pb-based IR-NLO chalcogenides. | |
To gain further insights into the underlying structure–property relationship of the title compound, systematic theoretical calculations were conducted, encompassing band structure, densities of states (DOSs), and linear optical and NLO parameters, based on DFT. The calculated electronic band structure reveals a direct Eg of 2.27 eV for RbPbPS4, as the valence band maximum (VBM) and conduction band minimum (CBM) coincide at the same high-symmetry k-points (Fig. 5a). The smaller calculated Eg can be attributed to underestimation by the DFT calculation.41 The partial DOSs of RbPbPS4 are depicted in Fig. 5b, indicating that the tops of the VBs are predominantly composed of S-3p states, with a minor contribution from Pb-5s and P-3p states, while the bottoms of the CBs primarily consist of S-3p, Pb-5p, P-3s/3p, and Rb-5p states. Furthermore, the NLO coefficients of RbPbPS4 can be calculated according to the electronic structure. In the light of Kleinman symmetry,42 RbPbPS4 exhibits only one nonzero independent SHG coefficient, d14 = 31.8 pm V−1 at 2900 nm (ca. 0.43 eV) (Fig. 5c). Furthermore, the calculated deff of RbPbPS4 is 26.88 pm V−1, under 2900 nm irradiation,35 which essentially coincides with the experimental findings. Additionally, the calculated Δn value of RbPbPS4 is 0.109 at 2900 nm, significantly surpassing that of AgGaS2 (Δn ∼ 0.04).43 This suggests that RbPbPS4 may be well-suited to achieve the PM condition for the SHG process. Furthermore, the line refractive dispersion diagrams indicate that the shortest type-I phase-matched output wavelength is 602 nm (Fig. 5d).44
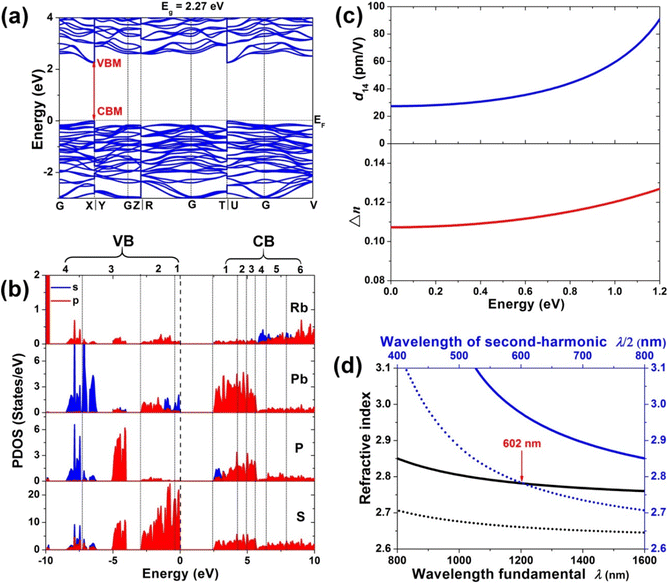 |
| Fig. 5 Theoretical results of electronic structures and optical parameters of PbPbPS4: (a) band structure; (b) partial density of states; (c) calculated nonzero independent SHG coefficient d14 (pm V−1) and calculated birefringence (Δn); (d) calculated refractive index dispersion curves with the shortest type-I PM cut-off wavelength. | |
To visually illustrate the contribution of the constituent BBUs to the SHG effect, the cutoff energy dependence of the static d14 for RbPbPS4 is calculated using a length-gauge formalism method.45 As depicted in Fig. 6a, the d14 values exhibit an upward trend in the VB-1, VB-3, CB-1, CB-3, and CB-5 intervals. This illustrates that the orbitals in these regions have a significant influence on the overall NLO response.46 Moreover, by integrating the partial DOSs (Fig. 5b) with the relevant partial charge density maps (Fig. 6b), it is evident that the enhancement of the deff in RbPbPS4 primarily stems from the strong hybridization of electron states at the top of the VBs and the substantial distortion of [PbS7] and [PS4] groups, indicative of the 2D [PbPS4]− alternating arrangement layer.
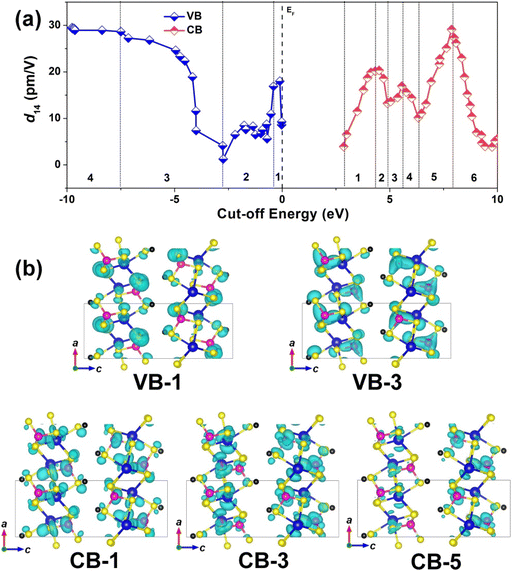 |
| Fig. 6 Theoretical analysis of the intrinsic mechanism of SHG source for PbPbPS4: (a) cut-off energy (eV) dependence of the static d14 (pm V−1); (b) distribution of the partial charge density maps with major contributions in the VB-1, VB-3, CB-1, CB-3 and CB-5 regions. Black atoms: Rb; blue atoms: Pb; pink atoms: P; yellow atoms: S. | |
Conclusions
In summary, building upon the parent ternary CS compound Rb3PS4, we implemented a strategy involving the partial substitution of Rb with SCALP Pb, resulting in the creation of a new quaternary NCS chalcophosphate, RbPbPS4. This substitution also triggered a structural transformation from 0D clusters to 2D layers. As anticipated, experimental findings highlight RbPbPS4 as a promising candidate for IR-NLO applications, boasting a large deff (2.5 × AgGaS2 at 2900 nm), a broad transmittance cutoff window (0.38–18.1 μm), and a significant Eg (2.75 eV) conducive to a high LIDT (7.5 × AgGaS2 at 1064 nm). Detailed structure–property analyses elucidate that the strong calculated deff (26.88 pm V−1@2900 nm) and large calculated birefringence (0.109@2900 nm) in RbPbPS4 primarily stem from the cooperative effects of [PbS7] and [PS4] NLO-active motifs. These findings underscore the efficacy of the lone-pair-cation substitution strategy in achieving both structural transformation and effectively balancing strong deff with a large Eg in SCALP-based chalcogenides.
Author contributions
A-Yang Wang: investigation, formal analysis, writing – original draft. Sheng-Hua Zhou: investigation, methodology, validation. Mao-Yin Ran: investigation, formal analysis, validation. Bingxuan Li: formal analysis, validation. Xin-Tao Wu: conceptualization, writing – review & editing. Hua Lin: supervision, conceptualization, writing – review & editing. Qi-Long Zhu: supervision, writing – review & editing.
Conflicts of interest
There are no conflicts to declare.
Acknowledgements
This work was supported by the National Natural Science Foundation of China (22175175), Natural Science Foundation of Fujian Province (2022L3092 and 2023H0041), Fujian Science & Technology Innovation Laboratory for Optoelectronic Information of China (2021ZR118), and the Youth Innovation Promotion Association CAS (2022303). The authors thank Prof. Yong-Fan Zhang at Fuzhou University for helping with the DFT calculations.
References
-
(a)
F. J. Duarte, Tunable Laser Applications, CRC Press, Boca Raton, FL, 2008, ch. 2, 9 and 12 CrossRef;
(b) V. Petrov, Frequency down-conversion of solid-state laser sources to the mid-infrared spectral range using non-oxide nonlinear crystals, Prog. Quantum Electron., 2015, 44, 1–106 CrossRef;
(c) X.-T. Wu and L. Chen, Structure-Property Relationships in Nonlinear Optical Crystals II The IR Region, Structure Bonding, 2012, 145, 1–42 Search PubMed;
(d) N. L. B. Sayson, T. Bi, V. Ng, H. Pham, L. S. Trainor, H. G. L. Schwefel, S. Coen, M. Erkintalo and S. G. Murdoch, Octave-spanning tunable parametric oscillation in crystalline Kerr microresonators, Nat. Photonics, 2019, 13, 701–706 CrossRef CAS;
(e) J.-X. Zhang, P. Feng, M.-Y. Ran, X.-T. Wu, H. Lin and Q.-L. Zhu, Ga-based IR nonlinear optical materials: Synthesis, structures, and properties, Coord. Chem. Rev., 2024, 502, 215617 CrossRef CAS.
-
(a) L. Kang, M. Zhou, J. Yao, Z. Lin, Y. Wu and C. Chen, Metal Thiophosphates with Good Mid-infrared Nonlinear Optical Performances: A First-Principles Prediction and Analysis, J. Am. Chem. Soc., 2015, 137, 13049–13059 CrossRef CAS PubMed;
(b) P. S. Halasyamani and W. Zhang, Viewpoint: Inorganic Materials for UV and Deep-UV Nonlinear-Optical Applications, Inorg. Chem., 2017, 56, 12077–12085 CrossRef CAS PubMed;
(c) Y. Pan, S.-P. Guo, B.-W. Liu, H.-G. Xue and G.-C. Guo, Second-order nonlinear optical crystals with mixed anions, Coord. Chem. Rev., 2018, 374, 464–496 CrossRef CAS;
(d) M. Mutailipu, K. R. Poeppelmeier and S. Pan, Borates: A Rich Source for Optical Materials, Chem. Rev., 2021, 121, 1130–1202 CrossRef CAS PubMed;
(e) H. Chen, M.-Y. Ran, W.-B. Wei, X.-T. Wu, H. Lin and Q.-L. Zhu, A comprehensive review on metal chalcogenides with three-dimensional frameworks for infrared nonlinear optical applications, Coord. Chem. Rev., 2022, 470, 214706 CrossRef CAS.
-
(a) A. Harasaki and K. J. Kato, New Data on the Nonlinear Optical Constant, Phase–Matching, and Optical Damage of AgGaS2, Appl. Phys., 1997, 36, 700–703 CAS;
(b) G. C. Catella, L. R. Shiozawa, J. R. Hietanen, R. C. Eckardt, R. K. Route, R. S. Feigelson, D. G. Cooper and C. L. Marquardt, Mid–IR absorption in AgGaSe2 optical parametric oscillator crystals, Appl. Opt., 1993, 32, 3948–3951 CrossRef CAS PubMed.
- G. D. Boyd, E. Buehler and F. G. Storz, Linear and nonlinear optical properties of ZnGeP2 and CdSe, Appl. Phys. Lett., 1971, 18, 301–304 CrossRef CAS.
-
(a) K. Wu and S. Pan, A review on structure-performance relationship toward the optimal design of infrared nonlinear optical materials with balanced performances, Coord. Chem. Rev., 2018, 377, 191–208 CrossRef CAS;
(b) L. Kang, F. Liang, X. Jiang, Z. Lin and C. Chen, First-Principles Design and Simulations Promote the Development of Nonlinear Optical Crystals, Acc. Chem. Res., 2020, 53, 209–217 CrossRef CAS PubMed;
(c) W. Wang, D. Mei, F. Liang, J. Zhao, Y. Wu and Z. Lin, Inherent laws between tetrahedral arrangement pattern and optical performance in tetrahedron-based mid-infrared nonlinear optical materials, Coord. Chem. Rev., 2020, 421, 213444 CrossRef CAS;
(d) H.-D. Yang, M.-Y. Ran, W.-B. Wei, X.-T. Wu, H. Lin and Q.-L. Zhu, Recent advances in IR nonlinear optical chalcogenides with well-balanced comprehensive performance, Mater. Today Phys., 2023, 35, 101127 CrossRef CAS;
(e) L. Luo, L. Wang, J. Chen, J. Zhou, Z. Yang, S. Pan and J. Li, AIB3IIC3IIIQ8VI: A New Family for the Design of Infrared
Nonlinear Optical Materials by Coupling Octahedra and Tetrahedra Units, J. Am. Chem. Soc., 2022, 144, 21916–21925 CrossRef CAS PubMed;
(f) M.-Y. Ran, S.-H. Zhou, W.-B. Wei, B.-X. Li, X.-T. Wu, H. Lin and Q.-L. Zhu, Breaking through the Trade-Off between Wide Band Gap and Large SHG Coefficient in Mercury-based Chalcogenides for IR Nonlinear Optical Application, Small, 2024, 20, 2304563 CrossRef CAS PubMed;
(g) W. Zhou and S.-P. Guo, Rational Design of Novel Promising Infrared Nonlinear Optical Materials: Structural Chemistry and Balanced Performances, Acc. Chem. Res., 2024, 57, 648–660 CAS.
-
(a) P. S. Halasyamani and K. R. Poeppelmeier, Noncentrosymmetric Oxides, Chem. Mater., 1998, 10, 2753–2769 CrossRef CAS;
(b) K. M. Ok, Toward the Rational Design of Novel Noncentrosymmetric Materials: Factors Influencing the Framework Structures, Acc. Chem. Res., 2016, 49, 2774–2785 CrossRef CAS PubMed;
(c) Y. Hu, C. Wu, X. Jiang, Z. Wang, Z. Huang, Z. Lin, X. Long, M. G. Humphrey and C. Zhang, Giant Second-Harmonic Generation Response and Large Band Gap in the Partially Fluorinated Mid-Infrared Oxide RbTeMo2O8F, J. Am. Chem. Soc., 2021, 143, 12455–12459 CrossRef CAS PubMed;
(d) H. Chen, W.-B. Wei, H. Lin and X.-T. Wu, Transition-metal-based chalcogenides: A rich source of infrared nonlinear optical materials, Coord. Chem. Rev., 2021, 448, 214154 CrossRef CAS;
(e) P.-F. Li, J.-G. Mao and F. Kong, A survey of stereoactive oxysalts for linear and nonlinear optical applications, Mater. Today Phys., 2023, 37, 101197 CrossRef CAS.
-
(a) M. Yan, H.-G. Xue and S.-P. Guo, Recent Achievements in Lone-Pair Cation-Based Infrared Second-Order Nonlinear Optical Materials, Cryst. Growth Des., 2021, 21, 698–720 CrossRef CAS;
(b) T. K. Bera, J.-H. Song, A. J. Freeman, J. I. Jang, J. B. Ketterson and M. G. Kanatzidis, Soluble direct-band-gap semiconductors LiAsS2 and NaAsS2: Large electronic structure effects from weak As center dot center dot center dot S interactions and strong nonlinear optical response, Angew. Chem., Int. Ed., 2008, 47, 7828–7832 CrossRef CAS PubMed;
(c) F. Xu, X. Xu, B. Li, G. Zhang, C. Zheng, J. Chen and N. Ye, Hg3AsS4X (X = Cl and Br): two Hg-based chalcogenides as long-wave infrared nonlinear optical crystals with superior comprehensive performances, Inorg. Chem. Front., 2024, 11, 2105–2115 RSC;
(d) H. Lin, Y. Y. Li, M. Y. Li, Z. J. Ma, L. M. Wu, X. T. Wu and Q. L. Zhu, Centric-to-acentric structure transformation induced by a stereochemically active lone pair: a new insight for design of IR nonlinear optical materials, J. Mater. Chem. C, 2019, 7, 4638–4643 RSC;
(e) Y. Xiao, M. M. Chen, Y. Y. Shen, P. F. Liu, H. Lin and Y. Liu, A3Mn2Sb3S8 (A = K, Rb): A new type of multifunctional infrared nonlinear optical materials based on unique three-dimensional open frameworks, Inorg. Chem. Front., 2021, 8, 2835–2843 RSC;
(f) H.-J. Zhao, H.-D. Yang, P.-F. Liu and H. Lin, From Cc to P63mc: Structural Variation in La3S2Cl2[SbS3] and La3OSCl2[SbS3] Induced by the Isovalent Anion Substitution, Cryst. Growth Des., 2022, 22, 1437–1444 CrossRef CAS;
(g) Y. Zhou, L.-T. Jiang, X.-M. Jiang, B.-W. Liu and G.-C. Guo, Mixed-anion square-pyramid [SbS3I2] units causing strong second-harmonic generation intensity and large birefringence, Chin. Chem. Lett., 2024, 109740 CrossRef;
(h) M. Y. Li, B. X. Li, H. Lin, Z. J. Ma, L. M. Wu, X. T. Wu and Q. L. Zhu, Sn2Ga2S5: A Polar Semiconductor with Exceptional Infrared Nonlinear Optical Properties Originating from the Combined Effect of Mixed Asymmetric Building Motifs, Chem. Mater., 2019, 31, 6268–6275 CrossRef CAS;
(i) C. Zhao, B. Zhang, X. Tian, G. Zhou, J. Xu and K. Wu, Na6Sn3P4S16: Sn(II)-chelated PS4 groups inspired an ultra-strong SHG response, Inorg. Chem. Front., 2023, 10, 5726–5733 RSC;
(j) X. Chen, Q. Jing and K. M. Ok, Pb18O8Cl15I5: A Polar Lead Mixed Oxyhalide with Unprecedented Architecture and Excellent Infrared Nonlinear Optical Properties, Angew. Chem., Int. Ed., 2020, 59, 20323–20327 CrossRef CAS PubMed.
-
(a) Y. K. Chen, M. C. Chen, L. J. Zhou, L. Chen and L. M. Wu, Syntheses, Structures, and Nonlinear Optical Properties of Quaternary Chalcogenides: Pb4Ga4GeQ12 (Q = S, Se), Inorg. Chem., 2013, 52, 8334–8341 CrossRef CAS PubMed;
(b) M. Zhou, X. Jiang, Y. Guo, Z. Lin, J. Yao and Y. Wu, Pb0.65Mn2.85Ga3S8 and Pb0.72Mn2.84Ga2.95Se8: Two Quaternary Metal Chalcognides with Open-Tunnel-Framework Structures Displaying Intense Second Harmonic Generation Responses and Interesting Magnetic Properties, Inorg. Chem., 2017, 56, 8454–8461 CrossRef CAS PubMed;
(c) Z. Z. Luo, C. S. Lin, H. H. Cui, W. L. Zhang, H. Zhang, H. Chen, Z. Z. He and W. D. Cheng, PbGa2MSe6 (M = Si, Ge): Two Exceptional Infrared Nonlinear Optical Crystals, Chem. Mater., 2015, 27, 914–922 CrossRef CAS;
(d) R. H. Duan, J. S. Yu, H. Lin, Y. J. Zheng, H. J. Zhao, S. X. Huang-Fu, M. A. Khan, L. Chen and L. M. Wu, Pb5Ga6ZnS15: a noncentrosymmetric framework with chains of T2-supertetrahedra, Dalton Trans., 2016, 45, 12288–12291 RSC;
(e) W. F. Chen, B. W. Liu, X. M. Jiang and G. C. Guo, Infrared nonlinear optical performances of a new sulfide β-PbGa2S4, J. Alloys Compd., 2022, 905, 164090 CrossRef CAS;
(f) Z. X. Wu, W. F. Chen, X. M. Jiang, B. W. Liu and G. C. Guo, [Na2PbI][Ga7S12]: Combining Diamond-Like Anionic Framework with Polycationic Chain toward Achieving Remarkable Nonlinear Optical Response, Chem. Mater., 2024, 36, 3444–3451 CrossRef CAS;
(g) W. F. Chen, B. W. Liu, S. M. Pei, X. M. Jiang and G. C. Guo, [K2PbX][Ga7S12] (X = Cl, Br, I): The First Lead-Containing Cationic Moieties with Ultrahigh Second-Harmonic Generation and Band Gaps Exceeding the Criterion of 2.33 eV, Adv. Sci., 2023, 10, 2207630 CrossRef CAS PubMed;
(h) J. Zhou, H. Wang, J. Liu, X. Su, Y. Chu, J. Qu and X. Jiang, Pb3.5GeS4Br3: The first phase-matching thiogermanate halide infrared nonlinear optical material, Inorg. Chem. Front., 2024, 11, 2681–2689 RSC;
(i) J. Wang, H. Wu, H. Yu, Z. Hu, J. Wang and Y. Wu, Pb4SeBr6: A Congruently Melting Mid-Infrared Nonlinear Optical Material with Excellent Comprehensive Performance, Adv. Opt. Mater., 2022, 10, 2102673 CrossRef CAS;
(j) X. Li, L. Kang, C. Li, Z. Lin, J. Yao and Y. Wu, PbGa4S7: a wide-gap nonlinear optical material, J. Mater. Chem. C, 2015, 3, 3060–3067 RSC.
- J. Chen, Q. Wu, H. Tian, X. Jiang, F. Xu, X. Zhao, Z. Lin, M. Luo and N. Ye, Uncovering a Vital Band Gap Mechanism of Pnictides, Adv. Sci., 2022, 9, 2105787 CrossRef CAS PubMed.
-
(a) H. Lin, W. B. Wei, H. Chen, X. T. Wu and Q. L. Zhu, Rational design of infrared nonlinear optical chalcogenides by chemical substitution, Coord. Chem. Rev., 2020, 406, 213150 CrossRef CAS;
(b) M.-Y. Ran, A. Y. Wang, W. B. Wei, X.-T. Wu, H. Lin and Q.-L. Zhu, Recent progress in the design of IR nonlinear optical materials by partial chemical substitution: Structural evolution and performance optimization, Coord. Chem.
Rev., 2023, 481, 215059 CrossRef CAS.
- M.-M. Chen, Z. Ma, B.-X. Li, W.-B. Wei, X.-T. Wu, H. Lin and Q.-L. Zhu, M2As2Q5 (M = Ba, Pb Q = S, Se): A source of infrared nonlinear optical materials with excellent overall performance activated by multiple discrete arsenate anions, J. Mater. Chem. C, 2021, 9, 1156–1163 RSC.
- C. Liu, S.-H. Zhou, Y. Xiao, C. Zhang, H. Lin and Y. Liu, Aliovalent-cation-substitution-induced structure transformation: a new path toward high-performance IR nonlinear optical materials, J. Mater. Chem. C, 2021, 9, 15407–15414 RSC.
- M. M. Chen, S. H. Zhou, W. B. Wei, B. X. Li, M. Y. Ran, X. T. Wu, H. Lin and Q. L. Zhu, RbBiP2S6: A Promising IR Nonlinear Optical Material with a Giant Second-Harmonic Generation Response Designed by Aliovalent Substitution, ACS Mater. Lett., 2022, 4, 1264–1269 CrossRef CAS.
- V. Nguyen, B. Ji, K. Wu, B. Zhang and J. Wang, Unprecedented mid-infrared nonlinear optical materials achieved by crystal structure engineering, a case study of (KX)P2S6 (X = Sb, Bi, Ba), Chem. Sci., 2022, 13, 2640–2648 RSC.
- X. H. Li, Z. H. Shi, M. Yang, W. Liu and S. P. Guo, Sn7Br10S2: The First Ternary Halogen–Rich Chalcohalide Exhibiting a Chiral Structure and Pronounced Nonlinear Optical Properties, Angew. Chem., Int. Ed., 2022, 61, e202115871 CrossRef CAS PubMed.
- L. T. Menezes, A. Assoud, W. Zhang, P. S. Halasyamani and H. Kleinke, Effect of Pb Substitution in Sr(2-x)PbxGeSe4 on Crystal Structures and Nonlinear Optical Properties Predicted by DFT Calculations, Inorg. Chem., 2020, 59, 15028–15035 CrossRef CAS PubMed.
- J. Wang, H. Wu, H. Yu, Z. Hu, J. Wang and Y. Wu, Pb4SeBr6: A Congruently Melting Mid-Infrared Nonlinear Optical Material with Excellent Comprehensive Performance, Adv. Opt. Mater., 2022, 10, 2102673 CrossRef CAS.
- L.-B. Wu and F.-Q. Huang, Crystal structure of trirubidium tetrathiophosphate, Rb3PS4, Z. Kristallogr. – New Cryst. Struct., 2005, 220, 122–122 CAS.
-
(a) J. Yao and J. A. Ibers, RbPbPS4, Acta Crystallogr., Sect. E: Struct. Rep. Online, 2004, 60, i108–i110 CrossRef CAS;
(b) I. Belkyal, M. ElAzhari, Y. D. Wu, W. Bensch and W. Depmeier, Phase transition of rubidium lead tetrathiophosphate RbPbPS4, Z. Anorg. Allg. Chem., 2009, 635, 1600–1603 CrossRef CAS.
- Y. Z. Huang, L. Chen and L. M. Wu, Submicrosized Rods, Cables, and Tubes of ZnE (E = S, Se, Te): Exterior–Interior Boron-Chalcogen Conversions and Optical Properties, Inorg. Chem., 2008, 47, 10723–10728 CrossRef CAS PubMed.
- P. Kubelka and F. Munk, Reflection characteristics of paints, Z. Tech. Phys., 1931, 12, 593–601 Search PubMed.
-
U. Englert, Symmetry Relationships between Crystal Structures, Oxford University Press: Oxford, UK, 2013 Search PubMed.
- R. Dronskowski and P. E. Bloechl, Crystal orbital Hamilton populations (COHP): energy-resolved visualization of chemical bonding in solids based on density-functional calculations, J. Phys. Chem., 1993, 97, 8617–8624 CrossRef CAS.
- T. Komm, D. Gudat and T. Schleid, Die Lanthanid (III)-ortho-Thiophosphate(V) vom Typ M[PS4](M = La–Nd, Sm, Gd–Er): Synthese, Kristallstruktur und 31P-NMR-Untersuchungen, Z. Naturforsch., 2006, 61b, 766–774 CrossRef.
- F. Pielnhofer, L. M. Schoop, A. Kuhn, R. Eger, J. Nuss, H. Nuss and B. V. Lotsch, New Light on an Old Story: The Crystal Structure of Boron Tetrathiophosphate Revisited, Z. Anorg. Allg. Chem., 2019, 645, 267–271 CrossRef CAS.
- P. Buck and C. D. Carpentier, The Crystal Structure of Gallium Thiophosphate, GaPS4, Acta Crystallogr., Sect. B: Struct. Crystallogr. Cryst. Chem., 1973, 29, 1864–1868 CrossRef CAS.
- P. Toffoli, P. Khodadad and N. Rodier, Structure cristalline du tetrathiomonophosphate (V) de thallium(I) Tl3PS4, Bull. Soc. Chim. Fr., 1981, 429–432 CAS.
- C. D. Malliakas and M. G. Kanatzidis, Inorganic Single Wall Nanotubes of SbPS4(-xSex (0 ≤ x≤3) with Tunable Band Gap, J. Am. Chem. Soc., 2006, 128, 6538–6539 CrossRef CAS PubMed.
- D. Tiwari, D. Alibhai, D. Cherns and D. J. Fermin, Crystal and Electronic Structure of Bismuth Thiophosphate, BiPS4: An Earth-Abundant Solar Absorber, Chem. Mater., 2020, 32, 1235–1242 CrossRef CAS.
- F. Alahmari, B. Davaasuren, J. Khanderi and A. Rothenberger, Synthesis and Characterization of the Rubidium Thiophosphate Rb6(PS5)(P2S10) and the Rubidium Silver Thiophosphates Rb2AgPS4, RbAg5(PS4)2 and Rb3Ag9(PS4)4, Z. Anorg. Allg. Chem., 2016, 642, 361–367 CrossRef CAS.
- A. Mesbah, J. Prakash, D. Rocca, S. Lebègue, J. C. Beard, B. A. Lewis and J. A. Ibers, Syntheses, crystal structure, and electronic properties of the five ABaMQ4 compounds RbBaPS4, CsBaPS4, CsBaVS4, RbBaVSe4, and CsBaVSe4, J. Solid State Chem., 2016, 233, 217–220 CrossRef CAS.
- Y. Huang, J. Huang and Y. Zhang, Wide band gap thiophosphates ASrPS4 (A = Li, Na, K, Rb, Cs): cation size effect induced successive structural transformation, Dalton Trans., 2022, 51, 15067–15073 RSC.
- S. Coste, J. Hanko, M. Bujoli-Doeuff, G. Louarn, M. Evain, R. Brec, B. Alonso, S. Jobic and M. G. Kanatzidis, NaPdPS4 and RbPdPS4: systems with infinite straight
[PdPS4]− chains soluble in polar solvents and the structure of cubic RbPdPS4{Rb0.33P0.4S2.23Ox}, J. Solid State Chem., 2003, 175, 133–145 CrossRef CAS.
- W. Xing, C. Tang, P. Gong, J. Wu, Z. Lin, J. Yao, W. Yin and B. Kang, Investigation into Structural Variation from 3D to 1D and Strong Second Harmonic Generation of the AHgPS4 (A+ = Na+, K+, Rb+, Cs+) Family, Inorg. Chem., 2021, 60, 18370–18378 CrossRef CAS PubMed.
- S. K. Kurtz and T. T. Perry, A powder technique for the evaluation of nonlinear optical materials, J. Appl. Phys., 1968, 39, 3798–3813 CrossRef CAS.
- M. J. Zhang, X. M. Jiang, L. J. Zhou and G. C. Guo, Two phases of Ga2S3: promising infrared second-order nonlinear optical materials with very high laser induced damage thresholds, J. Mater. Chem. C, 2013, 1, 4754–4760 RSC.
- J.-H. Zhang, D. J. Clark, J. A. Brant, K. A. Rosmus, P. Grima, J. W. Lekse, J. I. Jang and J. A. Aitken, α-Li2ZnGeS4: A Wide-Bandgap Diamond-like Semiconductor with Excellent Balance between Laser-Induced Damage Threshold and Second Harmonic Generation Response, Chem. Mater., 2020, 32, 8947–8955 CrossRef CAS.
- J.-H. Zhang, S. S. Stoyko, A. J. Craig, P. Grima, J. W. Kotchey, J. I. Jang and J. A. Aitken, Phase Matching, Strong Frequency Doubling, and Outstanding Laser-Induced Damage Threshold in the Biaxial, Quaternary Diamond-like Semiconductor Li4CdSn2S7, Chem. Mater., 2020, 32, 10045–10054 CrossRef CAS.
- L. Luo, L. Wang, J. Chen, J. Zhou, Z. Yang, S. Pan and J. Li, AIBII3CIII3QVI8: A New Family for the Design of Infrared Nonlinear Optical Materials by Coupling Octahedra and Tetrahedra Units, J. Am. Chem. Soc., 2022, 144, 21916–21925 CrossRef CAS PubMed.
- S. Yang, C. Lin, H. Fan, K. Chen, G. Zhang, N. Ye and M. Luo, Polar Phosphorus Chalcogenide Cage Molecules: Enhancement of Nonlinear Optical Properties in Adducts, Angew. Chem., Int. Ed., 2023, 62, e202218272 CrossRef CAS PubMed.
- K. Burke, Perspective on density functional theory, J. Chem. Phys., 2012, 136, 150901 CrossRef PubMed.
- D. A. Kleinman, Nonlinear Dielectric Polarization in Optical Media, Phys. Rev., 1962, 126, 1977–1979 CrossRef CAS.
-
(a) M. Y. Li, Z. J. Ma, B. X. Li, X. T. Wu, H. Lin and Q. L. Zhu, HgCuPS4: An Exceptional Infrared Nonlinear Optical Material with Defect Diamond-like Structure, Chem. Mater., 2020, 32, 4331–4339 CrossRef CAS;
(b) M. Y. Ran, Z. J. Ma, H. Chen, B. X. Li, X. T. Wu, H. Lin and Q. L. Zhu, Partial Isovalent Anion Substitution to Access Remarkable Second-Harmonic Generation Response: A Generic and Effective Strategy for Design of Infrared Nonlinear Optical Materials, Chem. Mater., 2020, 32, 5890–5896 CrossRef CAS;
(c) M. M. Chen, S. H. Zhou, W. B. Wei, X. T. Wu, H. Lin and Q. L. Zhu, Phase Matchability Transformation in the Infrared Nonlinear Optical Materials with Diamond-Like Frameworks, Adv. Opt. Mater., 2022, 10, 2102123 CrossRef CAS.
-
(a) M. Y. Ran, S. H. Zhou, B. X. Li, W. B. Wei, X. T. Wu, H. Lin and Q. L. Zhu, Enhanced Second-Harmonic-Generation Efficiency and Birefringence in Melillite Oxychalcogenides Sr2MGe2OS6 (M = Mn, Zn, and Cd), Chem. Mater., 2022, 34, 3853–3861 CrossRef CAS;
(b) A.-Y. Wang, S. H. Zhou, M. Y. Ran, X. T. Wu, H. Lin and Q. L. Zhu, Regulating the key performance parameters for Hg-based IR NLO chalcogenides via bandgap engineering strategy, Chin. Chem. Lett., 2024, 35, 109377 Search PubMed;
(c) M. Y. Ran, S. H. Zhou, X. T. Wu, H. Lin and Q. L. Zhu, The first Hg-based oxychalcogenide Sr2HgGe2OS6: Achieving balanced IR nonlinear optical properties through synergistic cation and anion substitution, Mater. Today Phys., 2024, 44, 101442 CrossRef.
-
(a) C. Aversa and J. E. Sipe, Nonlinear optical susceptibilities of semiconductors: Results with a length-gauge analysis, Phys. Rev. B: Condens. Matter Mater. Phys., 1995, 52, 14636–14645 CrossRef CAS PubMed;
(b) S. N. Rashkeev, W. R. L. Lambrecht and B. Segall, Efficient ab initio method for the calculation of frequency-dependent second-order optical response in semiconductors, Phys. Rev. B: Condens. Matter Mater. Phys., 1998, 57, 3905–3919 CrossRef CAS.
-
(a) H. Chen, Y.-Y. Li, B.-X. Li, P.-F. Liu, H. Lin, Q.-L. Zhu and X.-T. Wu, Salt-Inclusion Chalcogenide [Ba4Cl2][ZnGa4S10]: Rational Design of an IR Nonlinear Optical Material with Superior Comprehensive Performance Derived from AgGaS2, Chem. Mater., 2020, 32, 8012–8019 CrossRef CAS;
(b) H.-D. Yang, M.-Y. Ran, S.-H. Zhou, B.-X. Li, X.-T. Wu, H. Lin and Q.-L. Zhu, Rational Design via Dual-Site Aliovalent Substitution Leads to an Outstanding IR Nonlinear Optical Material with Well-Balanced Comprehensive Properties, Chem. Sci., 2022, 13, 10725–10733 RSC;
(c) M.-Y. Ran, S.-H. Zhou, W.-B. Wei, B.-X. Li, X.-T. Wu, H. Lin and Q.-L. Zhu, Rational Design of a Rare-Earth Oxychalcogenide Nd3[Ga3O3S3][Ge2O7] with Superior Infrared Nonlinear Optical Performance, Small, 2023, 19, 2300248 CrossRef CAS PubMed;
(d) H. Chen, M.-Y. Ran, S.-H. Zhou, X.-T. Wu, H. Lin and Q.-L. Zhu, Simple yet extraordinary: super-polyhedra-built 3D chalcogenide framework of Cs5Ga9S16 with excellent infrared nonlinear optical performance, Chin. Chem. Lett., 2023, 34, 107838 CrossRef CAS.
Footnotes |
† Electronic supplementary information (ESI) available: Additional experimental and theory results, together with additional tables and figures. CCDC 2347371. For ESI and crystallographic data in CIF or other electronic format see DOI: https://doi.org/10.1039/d4qi01040j |
‡ A. Y. Wang and S. H. Zhou contributed equally to this work. |
|
This journal is © the Partner Organisations 2024 |