DOI:
10.1039/D3EY00050H
(Perspective)
EES. Catal., 2023,
1, 665-676
Advanced dual-atom catalysts for efficient oxygen evolution reaction
Received
4th March 2023
, Accepted 15th May 2023
First published on 16th May 2023
Abstract
Single-atom site catalysts (SACs) are widely used in various catalytic reactions because of their high atom utilization, mass activity, and selectivity. However, they have limitations in catalyzing multi-electron reactions due to their inherent mono-metal center feature. In recent years, dual-atom catalysts (DACs) have received substantial attention as they not only inherit the merits of SACs but also overcome their shortcomings. Therefore, this perspective aims to outline recent developments in DACs and deepen our understanding of the synergistic interactions between dual metal sites. The perspective begins by introducing efficient fabrication strategies for DACs, followed by depicting state-of-the-art characterization techniques. Exciting advances of DACs in the oxygen evolution reaction (OER) and their applications in powdered rechargeable battery devices are also discussed. Finally, some critical challenges of DACs are outlined, and futuristic perspectives are presented to enhance their feasibility in sustainable energy.
Broader context
Atomically dispersed metal catalysts with well-defined structures have emerged as a new research frontier in heterogeneous catalysis due to their high atomic utilization efficiency, activity, and selectivity. Dual-atom catalysts (DACs), as an extension of single-atom catalysts (SACs), possess more possibility to optimize the bonding mode and circumvent the scaling relationships due to the more accessible active sites and the consequent synergistic interactions. In this perspective, we discuss the latest intriguing advancements of DACs from controllable synthesis strategies, to the characterization tools and the electrocatalytic applications in the OER and OER-driven energy devices. We also propose technical routes to the rational design of versatile DACs, which are expected to be implemented in energy-related applications.
|
1. Introduction
Catalysis plays a paramount role in driving the development of our modern life, and has been applied to various fields such as the synthesis of fine chemicals, and pharmaceuticals, the production of fuels, and the conversion of renewable energy sources.1–6 Renewable energy storage and conversion technologies, in particular, offer a promising solution to address the pressing issues of the energy crisis, environmental concerns, and the greenhouse effect.7–14 The number of active sites and the intrinsic activity of each active site are well-known as the main factors determining the catalytic performance of a catalyst.15 Therefore, downsizing the catalyst is an effective way to enhance the number of exposed active sites, which not only could increase the catalytic activity but also reduce the cost significantly.16–20 Interestingly, the catalytic behaviors of the catalyst alter dramatically by reducing the size from bulk to nanoparticles, to nanocluster and even to single metal sites (Fig. 1a).21–23 It can be inferred that decreasing the size can also induce the alteration of the electronic structure owing to the changed structure and coordination configurations of the active sites.24,25 This effect is particularly noticeable when the size of the catalyst is below 1 nm, which offers the substantial possibility to tailor the electronic structure and the resulting catalytic activity and selectivity.26 As a result, atomically dispersed metal catalysts (ADMCs) have achieved considerable insights due to the high atom utilization, activity, and selectivity, and therefore have emerged as a new interest frontier.27–32 As one of the most prestigious representatives, single-atom site catalysts (SACs) have been widely used in a variety of catalytic fields, such as batteries,33,34 electrocatalysis,35–38 thermocatalysis,39,40 photocatalysis,41 and environmental protection,42 and have achieved great success.43–51
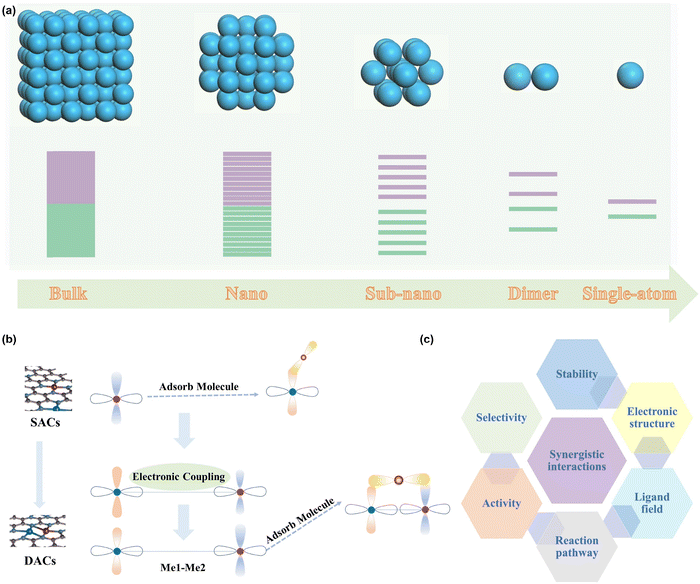 |
| Fig. 1 (a) Representative geometric and electronic structure for bulk metal, nanoparticle, cluster, dimer, and single-atom. (b) The electronic coupling interactions of DACs in tailoring the binding mode. (c) The synergistic interactions of DACs on the electronic structure, ligand field, reaction pathway, activity, selectivity, and stability. | |
Although SACs have demonstrated many exciting advances in various catalytic reactions, such as the hydrogen evolution reaction (HER),52–54 oxygen reduction reaction (ORR),55 and chlorine evolution reaction (CER),56 they also show obvious limitations in catalyzing some complex reactions with multiple intermediates due to their single metal-center.57,58 Therefore, the rational design of advanced ADMCs with much more active centers to provide sufficient adsorption sites for multiple reaction intermediates can be an effective strategy to overcome the weakness of SACs.59 As a consequence, dual-atom catalysts (DACs) featuring two active centers have recently become an energetic research frontier in the field of heterogeneous catalysis.60,61 Due to the synergistic effects between neighboring catalytically active sites, DACs offer significantly greater opportunities to catalyze multi-electron step reactions with diverse reaction products. The electronic coupling between adjacent atoms could optimize their binding mode toward different adsorbates, resulting in substantially reduced energy barriers and accelerated reaction kinetics (Fig. 1b).62,63 Additionally, synergistic interactions of DACs enable tuning the d-band center of active centers and circumvent the scaling relationships, offering more flexibility to tailor the catalytic performance.64–66 Therefore, synergistic effects endow the DACs with the accessibility of the modulation of the electronic structure, ligand field, reaction pathway, activity, selectivity, and stability (Fig. 1c). Due to these fantastic factors, DACs have attached great advances in many electrocatalytic reactions, such as the oxygen evolution action (OER),67–71 oxygen reduction reaction (ORR),72–78 carbon dioxide reduction (CO2RR),79–83 and HER.84–89 However, the inherent high-oxidation characteristic of the OER imposes stricter requirements on DACs, particularly in terms of stability issues. Therefore, a comprehensive perspective on the scientific breakthroughs of DACs applicable to the OER is of paramount significance to related renewable energy technologies.
This perspective briefly summarizes the exciting progress of DACs in the OER, emphasizing the fundamental aspects of the synergistic interactions between neighboring metal atoms, with the aim of providing a useful guideline for the rational design and application of DACs (Fig. 2). First, a brief discussion of the fabrication strategy, with a special focus on the pyrolysis method, is provided. Next, the state-of-the-art characterization techniques for DACs are discussed, along with the latest theoretical insights for a better understanding of the underlying structure–performance relationships. Subsequently, the recent developments of DACs in the OER and OER-driven energy devices, such as Zn/Al–air batteries (ZABs) and Zn–CO2 batteries, are presented. Finally, the remaining challenges and future perspectives of DACs in the OER are outlined to provide possible guidelines for the development of DACs. We anticipate that this perspective could inspire more efforts in the construction of advanced DACs for the OER and other catalytic reactions.
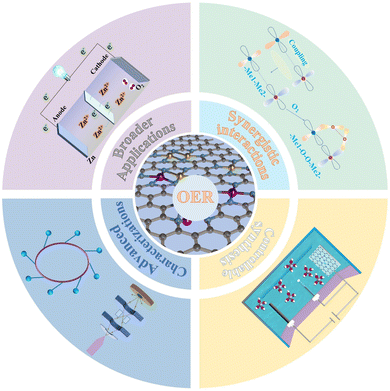 |
| Fig. 2 Schematic view of topics covered in this perspective for the advancement of DACs in the oxygen evolution reaction. | |
2. Construction strategies of DACs
Similar to the SACs, many synthesis methods such as pyrolysis,90–92 the wet chemical method,93,94 atomic layer deposition,95,96 electrochemical deposition,97,98 and photochemical deposition,99,100 have been developed to fabricate DACs. Among them, pyrolysis has been considered one of the most universal methods for the rational construction of DACs, especially for carbon-supported DACs. It can be realized by the pyrolysis process of organic substances encapsulated with metal precursors in an inert atmosphere. In this section, some representative DACs are listed to show the universality of pyrolysis in fabricating various ADMCs.
Li and coworkers have developed a series of SACs and DACs by the pyrolysis synthesis strategy.101,102 For example, they reported a 2D N-doped carbon nanosheet-supported Fe–Mn dual site catalyst (FeMn–NC DAC) using a molten salt-assisted pyrolysis method (Fig. 3a).103 They encapsulated the Fe(acac)3 into Zn-based zeolitic imidazolate frameworks (ZIF-8) to form an Fe/ZIF-8 composite and then Mn ions were trapped by Fe/ZIF-8 by the chelation of glucose–melamine. The mixture composed of Fe/ZIF-8@Mn/GM and KCl/ZnCl2 was subjected to a pyrolysis process at 900 °C for 2 h under an N2 environment to obtain the final FeMn–NC DAC. Bu et al. reported a “pre-constrained metal twins” strategy to synthesize the N-doped carbon-supported Fe–Co dual-atom catalyst (FeCo–NC DAC) (Fig. 3b).104 They fabricated the bimetallic phthalocyanine macromolecules (FeCoPc) as the structural moiety and encapsulated them in ZIF-8 followed by a high-temperature pyrolysis process to produce FeCo–NC DAC. Except for the ZIF-8, pyrolyzing metal–organic frameworks (MOFs)/metal precursor composites is also an effective way to fabricate DACs. Peng and co-workers reported an N-doped carbon Fe–Ni dual-site catalyst (FeNi–NC DAC) as a bifunctional catalyst for the OER and ORR (Fig. 3c).105 The FeNi–NC DAC was synthesized by pyrolyzing polydopamine (PDA) coated FeNi MOFs under NH3 followed by acid etching and a second annealing procedure. The obtained bifunctional FeNi–NC DAC shows great excellent performance in flexible quasi-solid-state Zn/Al–air batteries. Also, Xu et al. prepared a unique MOF with ordered aromatic ring arrays to load different trinuclear complexes, and then the obtained composite was then annealed at 1000 °C in Ar gas to produce the final DACs (Fig. 4d).106
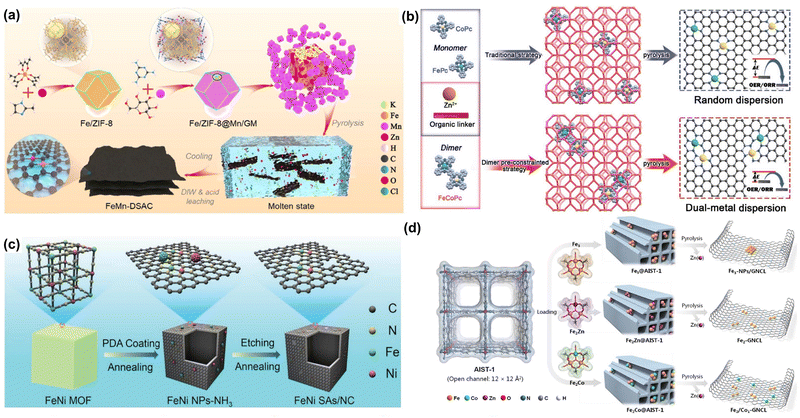 |
| Fig. 3 Representative pyrolysis synthesis methods of DACs. (a) Schematic illustration of the synthetic procedure of FeMn-DACs. Reprinted with permission.103 Copyright 2022 Wiley-VCH GmbH. (b) Pre-constrained metal twins strategy for fabricating FeCo/NC DACs. Reprinted with permission.104 Copyright 2022 Wiley-VCH GmbH. (c) Illustration scheme of FeNi/NC DACs. Reprinted with permission.105 Copyright 2021 Wiley-VCH GmbH. (d) Heteroatom modulator approach for fabricating Fe2-GNCL DACs. Reprinted with permission.106 Copyright 2020 Wiley-VCH GmbH. | |
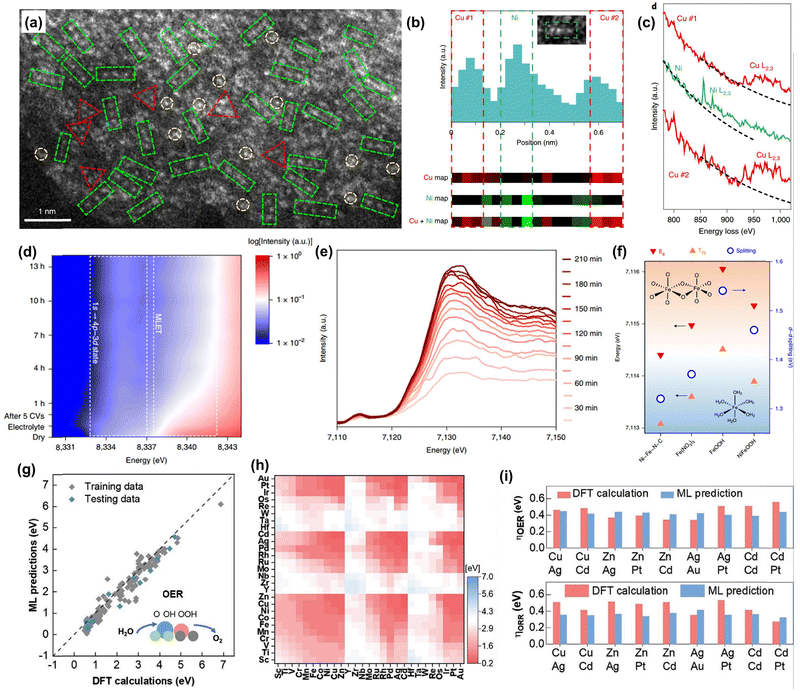 |
| Fig. 4 Advanced characterizations for DACs. (a) HAADF-STEM image of Ni1Cu2/g-C3N4. (b) Intensity profile and atomic-resolution EELS elemental mapping of a linear trimer indicated in the inset. (c) Atomic-resolution EELS spectra conducted at the corresponding positions in (b). Reprinted with permission.110 Copyright 2021 Springer Nature Publishing Group. (d) Operando Ni K-edge HERFD-XAS of NiFe–NC. (e) Operando Fe K-edge HERFD-XAS of NiFe–NC at different activation times. (f) Energy levels of Eg and T2g, and the ligand field splitting energy in different catalysts. Reprinted with permission.67 Copyright 2022 Springer Nature Publishing Group. (g) DFT calculated OER overpotentials versus the results from the ML model on the training and testing subsets. (h) ML predicted OER overpotentials. (i) Comparison of (top) the OER overpotential and (bottom) ORR overpotential of nine kinds of best DACs. Reprinted with permission.112 Copyright 2022 Wiley-VCH GmbH. | |
The pyrolysis strategy is highly useful in producing ADMCs with good conductivity, high specific surface area, and stability. Structurally well-defined metal precursors with precise nuclearity and organic supports are the critical factors during the synthesis process. Consequently, they should be precisely devised at the atomic level during the rational design of DACs using the pyrolysis strategy. However, it should be noted that it does appear challenging to obtain DACs in the absence of SACs, as previous research has shown. As a result, both single and dual metal active centers coexist, and their synergistic interactions could lead to the emergence of novel electronic properties that activate specific intermediates or optimize the bonding mode in tandem reaction steps, ultimately reducing the energy barrier of the reaction.
3. Advanced characterizations and theoretical insights
The identification of the geometric and electronic structure of the active metal centers of atomic catalysts plays a vital role in revealing the underlying structure–performance relationships.107 Aberration-corrected scanning transmission electron microscopy (AC-STEM) and X-ray absorption spectroscopy (XAS) are two of the most important characterization techniques for identifying the presence of single-metal sites, their corresponding ligand fields, and electronic properties in atomic catalysts. Furthermore, theoretical simulations, high-throughput computational calculations, and machine learning (ML) techniques have become increasingly valuable in exploring catalytic mechanisms and prescreening catalysts. In this context, we will provide a brief overview of their significance in characterizing DACs with the help of some representative examples.
High-angle annular dark-field scanning transmission electron microscopy (HAADF-STEM) is a powerful technique to identify the presence of single metal atom species and discern the coordination configurations of active centers.108,109 The dual metal atoms can be usually observed by the nearest brighter pair spots in the DACs, especially for the carbon-based DACs. This is usually regarded as one kind of evidence to prove the presence of a dimeric metal structural motif. However, HAADF-STEM has obvious limitations in identifying the metal species, especially for those of adjacent atomic numbers, where the contrast of atoms is close and therefore it is unlikely to distinguish them directly. As a result, the STEM equipped with electron energy loss spectroscopy (EELS) shows great capability in differentiating the elements of adjacent atomic numbers, which can be a critical technique in characterizing atomic catalysts. Due to the specific characteristic peak of different metal species, they can be easily distinguished by the energy position difference in the EELS spectrum. Lu et al. applied the EELS elemental mapping and spectrum techniques to assist in the identification of Ni and Cu atoms and their structural configurations in a Ni1Cu2 trimer anchored C3N4 catalyst. (Fig. 4a–c).110 In addition, the EELS technique can provide electronic and coordination structure information of metal species, and hence the STEM coupled with EELS will play a vital role in characterizing DACs.
In addition, the X-ray absorption spectroscopy (XAS) technique is usually used to discern the electronic and ligand properties of ADMCs. The XAS technique mainly includes X-ray absorption near edge structure (XANES) and extended X-ray adsorption fine structure (EXAFS), which could provide the chemical states and local coordination environment information of metal centers, respectively.40,111 Different from SACs, there is usually an exclusive peak that appeared after the main peak in the first shell in the Fourier transform (FT)-EXAFS spectrum of DACs, which can be attributed to the contribution of adjacent metal atoms. Therefore, the Fourier transform (FT)-EXAFS technique has been applied to identify the presence of a dimeric metal moiety. Also, it should be noted that wavelet transform (WT)-EXAFS can be an important complementary approach to confirm the existence of metal–metal scattering.
It should be mentioned that the operando XAS technique plays an increasing role in deciphering the dynamic catalytic mechanisms.113,114 The chemical valence states and coordination environment evolution of the metal center can be tracked during the catalytic process by conducting XAS analysis.115 Hu and co-workers reported a universal strategy to synthesize double-atom catalysts from their SAC precursors by in situ electrochemical deposition.67 They conducted an operando XAS experiment and analysis to confirm the successful synthesis of NiFe–NC DAC and the mass loading of Fe atoms. Also, the dynamic oxidation states and coordination environment of Ni and Fe dimer were monitored during the catalytic process (Fig. 4d–f).
Theoretical calculations have been regarded as a highly useful tool to discern the underlying catalytic mechanism and reaction pathways.116,117 Atomically precise theoretical simulations of DACs could offer significant insights into the possible elementary kinetic steps and activation energetics. In addition, the electronic properties of metal centers in DACs, involving d-band center, orbital filling, and surface charge distribution, can be discerned at the atomic level.21 For example, Qiu and co-workers conducted DFT calculations to screen the optimized oxygen electrocatalysts M1M2–NC (M = Fe, Co, and Ni) by examining the adsorption energy, electronic properties, and synergistic effects.118 It was found that FeCo–NC DAC can be the best bifunctional electrocatalyst due to the synergistic interaction between Fe and Co to enhance their OER and ORR activity. It should be worth noting that DFT calculations could provide valuable information in deciphering the synergistic effects between different metal atoms in the DACs. Through carefully examining these factors such as eg filling, spin state, charge difference and electronegativity, the origin of the synergistic effects of DACs can be revealed.
In addition, it should be mentioned that high-throughput calculations and machine-learning techniques have recently emerged as an energetic research frontier in the field of DACs.112,119 They have been widely exploited for catalyst prescreening and discovery without a considerable trial-and-error process. For instance, Li et al. reported a data-driven high-throughput design strategy to screen optimized OER/ORR catalysts from 16767 DACs (Fig. 4g–i).112 Using topological information-based machine-learning (ML) algorithms, they were able to screen 511 kinds of good OER DACs and 248 excellent bifunctional DACs. Importantly, this high-throughput method not only ensures outstanding prediction accuracy but also significantly reduces the catalyst screening time when compared with DFT calculations and experimental trial-and-error. This highlights the great potential of these techniques in accelerating the rational design of highly efficient and durable DACs.
4. Application in the OER-driven battery devices
DACs have emerged as highly promising catalysts for electrocatalytic reactions, including the HER, OER, CO2RR, and ORR. These catalysts exhibit remarkable activity, high selectivity, and outstanding durability due to the presence of dual metal sites and the resulting synergistic effects. Among these electrocatalytic reactions, the OER is particularly significant as it plays a paramount role in enhancing the energy usage efficiency of renewable energy technologies, such as water catalysis and metal–air battery devices.120–126 Considerable research works have verified the superiority of DACs in driving the OER with excellent activity and stability as well as high selectivity by enhancing the sluggish reaction kinetics and decoupling the inherent scaling relationships. Therefore, we will outline the recent exciting development of DACs in the OER and its powered renewable battery devices. In addition, Table 1 offers a summary of the OER performance of different DACs.
Table 1 Summary of the OER performance of DACs
Catalyst |
Support |
Overpotential@10 mA cm−2 (mV) |
Tafel slope (mV dec−1) |
Metal loading (wt%) |
Electrode |
Electrolyte |
Ref. |
NiCo–PNC |
PNC |
390 |
117 |
Co-2.15 |
Glassy carbon |
1 M KOH |
127
|
Ni-0.68 |
FeNi–NC |
NC |
∼320 |
40 |
|
Carbon-cloth |
1 M KOH |
67
|
FePt–NC |
NC |
310 |
62 |
Pt-2.1 |
Glassy carbon |
0.1 M KOH |
71
|
Fe-3.75 |
NiFe–PCN |
PCN |
310 |
38 |
Ni-15.36 |
Glassy carbon |
1 M KOH |
128
|
Fe-8.82 |
FeCo–NC |
NC |
349 |
99.93 |
Co-0.98 |
Glassy carbon |
0.1 M KOH |
69
|
Fe-0.34 |
NiCo–NC |
NC |
252 |
49 |
Ni-0.56 |
Carbon paper |
1 M KOH |
129
|
Co-1.04 |
FeCo–NC |
NC |
309 |
37 |
Co-1.77 |
Glassy carbon |
1 M KOH |
97
|
Fe-0.14 |
Ru–Co/ELCO |
ELCO |
247 |
49.1 |
|
Carbon paper |
1 M KOH |
130
|
FeNi–WCx |
WCx |
237 |
44 |
Ni-0.74 |
Glassy carbon |
1 M KOH |
131
|
Fe-0.62 |
NiCo–NC |
NC |
360 |
72 |
Ni-0.9 |
Glassy carbon |
0.1 M KOH |
132
|
Co-1.59 |
FeMn–NC |
NC |
405 |
96 |
|
Glassy carbon |
0.1 M KOH |
103
|
NiFe–GHS |
GHS |
390 |
81 |
Ni-1.9 |
Glassy carbon |
0.1 M KOH |
133
|
Fe-3.0 |
Fe2Co–GNCL |
GNCL |
350 |
70 |
Fe-0.48 |
Glassy carbon |
1 M KOH |
106
|
Co-0.19 |
FeNi–NC |
NC |
340 |
54 |
Ni-0.33 |
Glassy carbon |
1 M KOH |
134
|
Fe-0.57 |
FeCo–NC |
NC |
370 |
82.7 |
Co-0.8 |
Glassy carbon |
1 M KOH |
104
|
Fe-0.76 |
FeNi–NC |
NC |
270 |
74 |
Ni-2.2 |
Glassy carbon |
1 M KOH |
115
|
Fe-5.3 |
IrCo–NC |
NC |
330 |
79 |
Ir-0.18 |
Glassy carbon |
0.1 M KOH |
68
|
Co-1.02 |
It is found that DACs exhibit much higher OER activity than SACs due to the presence of dual metal sites. For instance, previous works have confirmed that N-doped carbon-supported DACs such as IrCo–NC, FePt–NC, and FeCo–NC deliver much lower overpotential than their SAC counterparts. However, they suffer from long-term stability issues and unsatisfactory activity during the OER process, especially under high potential due to the inevitable carbon corrosion. Therefore, developing efficient DACs with good corrosion-resistance capability in an oxidative environment is highly desired and urgent.108 Previous studies have demonstrated that some non-carbon-supported DACs not only exhibit intriguing OER activity but also outstanding long-term durability. Thomas and co-workers reported a highly efficient DAC for the OER comprised of WCx nanocrystalline-supported bi-atomic Fe–Ni metal sites (Fig. 5a).131 Microscopic and spectroscopic characterizations verified the presence of Fe/Ni atoms on the surface of the WCx support (Fig. 5b and c). Benefiting from its unique structure, the FeNi–WCx DAC showed an overpotential of only 237 mV to reach 10 mA cm−2, which is much lower than that of Fe–WCx and Ni–WCx SACs (Fig. 5e and f). Meanwhile, the FeNi–WCx DAC could deliver a high turnover frequency value of 4.96
s−1 at the overpotential of 300 mV and remarkable durability with 1000 h of operation (Fig. 5g). Similarly, Li et al. proposed a single-site cation coordination modulation strategy and established a Ru–Co pair-site catalyst by a single Ru atom substituting Co in LiCoO2 (Fig. 5g).130 Microscopic and spectroscopic characterizations demonstrated the presence of Ru–Co metal sites and their strong electronic interactions (Fig. 5h and i). Due to the strong electronic coupling between Ru and Co metal sites, the obtained Ru–Co/ELCO DAC showed superior OER activity with only 247 mV at 10 mA cm−2, 114 mV smaller than the benchmark of IrO2, and accelerated OER kinetics as well as excellent durability (Fig. 5j–l). Moreover, it displayed good long-term cycling stability in a Zn–air battery. Although there are already some works regarding non-carbon-supported DACs for the OER, the demand for versatile DACs still calls for more endeavors.
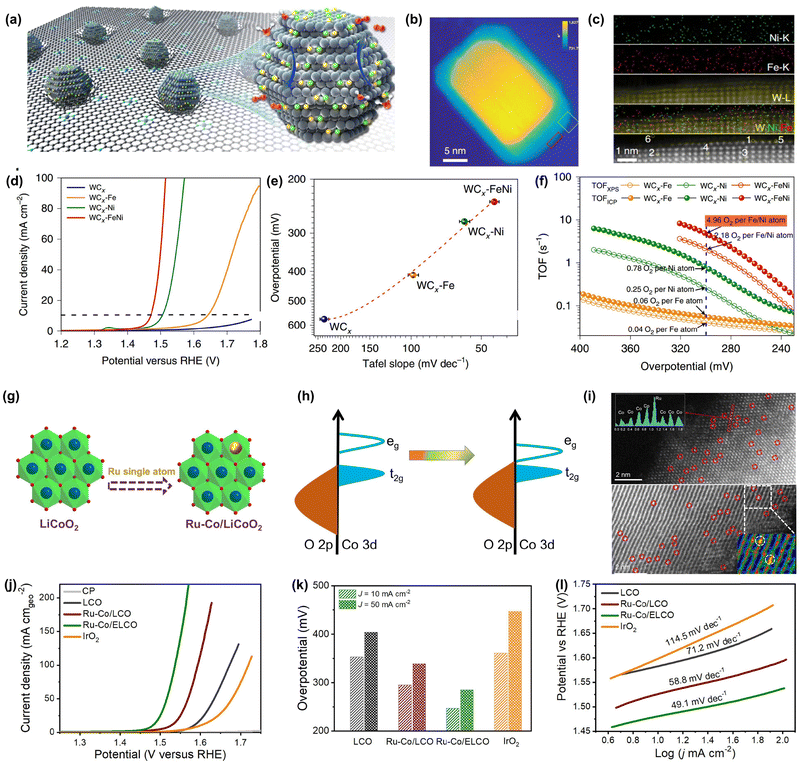 |
| Fig. 5 Representative DACs for the OER. (a) Schematic illustration of the Fe and Ni atom anchored WCx catalyst (FeNi–WCx). (b) HAADF-STEM image of WCx. (c) STEM-EDS mapping of FeNi–WCx. (d) LSV curves of FeNi–WCx and the corresponding counterparts. (e) The overpotential and Tafel slopes. (f) TOFs of FeNi–WCx and the corresponding counterparts at different overpotentials. Reprinted with permission.131 Copyright 2021 Springer Nature Publishing Group. (g) Schematic illustration of Ru–Co/LCO SAC. (h) Qualitative one electron energy diagram of Ru–Co/ELCO. (i) HAADF-STEM image of Ru–Co/ELCO. (j) LSV curve of Ru–Co/ELCO and (k) overpotentials at 10 and 50 mA cm−2. (l) Tafel slopes. Reprinted with permission.130 Copyright 2022 Wiley-VCH GmbH. | |
The presence of diatomic metal sites endows the catalyst with more possibility to alter the binding mode to diverse intermediates and the resulting binding strength, which enables the DACs to show multifunctionality in driving various reactions.115 This feature is particularly valuable for sustainable renewable energy technologies, such as metal–air batteries, where multifunctional catalysts are highly desired. Interestingly, some DACs have been found to exhibit superior activity for both the OER and ORR, making them a promising catalyst for renewable battery devices.118,134,135 For instance, Bu and co-workers constructed FeCo–NC DACs by a “pre-constrained metal twins” strategy, which showed both excellent OER and ORR activity.104 Inspired by this bifunctionality, a liquid rechargeable ZAB was assembled with FeCo–NC DAC as an air cathode, and it displayed high power density (175 mW cm−2) and outstanding stability after 240 h of operation, much better than that of ZAB assembled with Pt/C (Fig. 6a–c). Impressively, FeCo–NC DAC also delivered excellent flexibility with outstanding anti-deformation performance and durability in an all-solid-state ZAB (Fig. 6d). Similarly, Peng et al. prepared a bifunctional (OER/ORR) FeNi–NC DAC for flexible quasi-solid-state Zn/Al–air batteries.105 It was found that both Zn/Al–air batteries assembled with FeNi–NC DAC delivered high peak power densities and specific capacities as well as good stability under deformation conditions (Fig. 6e), which implies the great potential of DACs in the field of wearable devices. In addition, DACs may have great potential in rechargeable energy devices working under harsh conditions such as ultra-low temperature and high oxidation potential. Li and co-workers designed a FeMn–NC DAC consisting of Fe–N4 and Fe–N4 metal sites on N-doped carbon nanosheets for a flexible ZAB.103 Surprisingly, the assembled ZAB could operate at −40 °C and showed excellent cycling stability (Fig. 6f and g). Besides, DACs have extended their capability to drive other energy technologies. Chen et al. fabricated a FeNi–NC DAC by a pyrolysis method and the obtained catalysts showed both good CO2RR and OER activity and stability.136 The Zn–CO2 battery assembled with FeNi–NC DAC displayed high faradaic efficiency and superior cycling durability (Fig. 6h and i). Due to the versatility of DACs, it is expected that much more endeavors will be devoted to extending their application in various electrochemical applications.
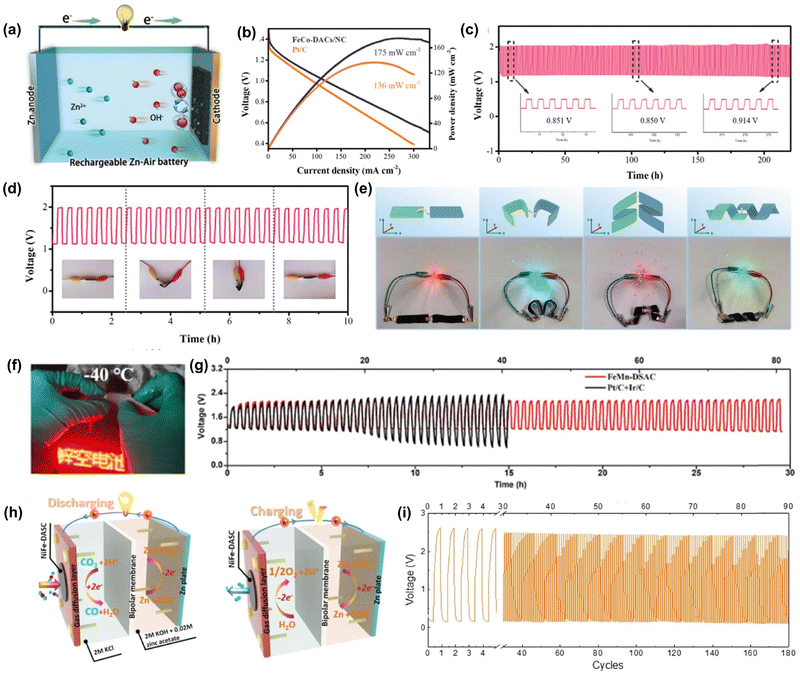 |
| Fig. 6 The electrocatalytic applications of DACs in the different rechargeable battery devices. (a) Schematic of the aqueous ZAB. (b) Discharge curves and power density of FeCo-DACs/NC ZAB. (c) Galvanostatic stability test of FeCo-DACs/NC ZAB at 10 mA cm2. (d) Galvanostatic stability test of the flexible all-solid-state ZAB assembled with FeCo-DACs/NC. Reprinted with permission.104 Copyright 2022 Wiley-VCH GmbH. (e) Schematic diagram and digital pictures of LEDs powered by two assembled Al–air batteries in different distortion states. Reprinted with permission.105 Copyright 2021 Wiley-VCH GmbH. (f) The optical figure of an LED powered by FeMn-DSAC ZAB upon bending at −40 °C. (g) Galvanostatic cycling stability of FeMn-DSAC ZAB at 2 mA cm2 under −40 °C. Reprinted with permission.103 Copyright 2022 Wiley-VCH GmbH. (h) Schematic illustration of the Zn–CO2 battery. (i) Galvanostatic discharge–charge cycling curves of FeNi–NC DAC at the discharge (5 mA cm2) and charge (2 mA cm2) current density for 180 cycles. Reprinted with permission.136 Copyright 2021 Springer Nature Publishing Group. | |
5. Summary and outlook
Highly efficient catalysts are essential for various catalytic reactions and energy-related applications. Despite the success of SACs in various catalytic fields, they still have limitations in catalyzing reactions with multiple intermediates. DACs have recently emerged as a new area of research that not only inherit the advantages of SACs but also overcome their shortcomings. Due to their more accessible active sites and the synergistic interactions between them, DACs have the potential to optimize bonding modes, overcome scaling relationships, reduce energy barriers, and generate diverse products. In this perspective, we summarize the recent developments in DACs, including controllable synthesis strategies, characterization tools, and electrocatalytic applications in the OER and related energy devices. We introduce the controllable fabrication strategies of DACs, with a special focus on the pyrolysis method. We describe state-of-the-art characterization techniques, including STEM-EELS and operando XAS, for identifying DACs and understanding their dynamic catalytic mechanisms. We also discuss DFT calculations, high-throughput calculations, and machine-learning techniques that reveal the origin of synergistic interactions and accelerate the discovery of DACs. Finally, we review recent progress in the OER and related renewable energy devices, highlighting the great potential of DACs for driving various reactions.
Despite some achievements made, there are still enormous scientific and technical challenges in the large-scale application of DACs. First, there is a need to establish universal and scalable fabrication strategies that can be applied to a wide range of DACs. Although various methods exist for synthesizing DACs, each approach has its limitations and cannot serve as a comprehensive solution. Therefore, it is crucial to develop advanced methods that can be broadly applied. Furthermore, the ability to synthesize DACs with precision and at a large scale remains a significant challenge, which hinders their commercialization and widespread use. To address this issue, there is a pressing need for simple and easy-to-implement synthesis methods that can produce atomically precise DACs. This can be achieved by carefully adjusting the metal precursors, host materials, and fabrication methods.
Second, the application of DACs is still limited, especially for OER-driven energy devices. While there are numerous DACs available for the OER, most are designed for alkaline environments and there is a scarcity of research on their performance in acidic conditions. Consequently, there is a need to develop highly effective and durable DACs that can perform exceptionally well and resist corrosion in acidic and oxidative environments. Some reported DACs such as RuCo/NC and FeNi/NC show promising potential for catalyzing the OER in acidic media owing to their chemical stability, which may make them suitable for use in proton-exchange membrane water electrolyzers (PEMWE). In addition, beyond their use in Zn–air batteries, DACs can be deployed in other sustainable energy devices such as Li/Na–O2/CO2 and Li–S batteries to exploit their multifunctionality, driving the rapid development of sustainable energy involving the practical application and fundamental studies.
Third, to enhance the understanding of catalytic mechanisms, it is essential to explore operando characterization techniques. Advanced technologies, such as operando XAS, Raman, and FTIR, can provide valuable information on identifying catalytically active centers, monitoring dynamic evolution of electronic and coordination environments, and exploring underlying catalytic mechanisms. Thus, further development of more efficient and sophisticated characterization techniques for DACs is highly desirable. Additionally, high-throughput calculations and machine-learning techniques could significantly aid in the discovery of advanced DACs using universal descriptors. Despite the remaining challenges, we are optimistic that with continued efforts in the field of DACs, significant progress will be made.
Conflicts of interest
The authors declare no conflict of interest.
Acknowledgements
This work was partially supported by the Beijing Natural Science Foundation (2224096), the National Key R&D Program of China (2018YFA0702003), the Australian Research Council Discovery Project (DP210102215) and the China Postdoctoral Science Foundation (2021M690086, 2021TQ0170). X. B. Zheng acknowledges funding support from the Office of China Postdoctoral Council (YJ20200277) and the “Shuimu Tsinghua Scholar Program (2020SM109)” of Tsinghua University, China.
References
- M. Luo, Z. Zhao, Y. Zhang, Y. Sun, Y. Xing, F. Lv, Y. Yang, X. Zhang, S. Hwang, Y. Qin, J. Y. Ma, F. Lin, D. Su, G. Lu and S. Guo, Nature, 2019, 574, 81–85 CrossRef CAS PubMed
.
- X. Tian, X. Zhao, Y. Q. Su, L. Wang, H. Wang, D. Dang, B. Chi, H. Liu, E. J. M. Hensen, X. W. D. Lou and B. Y. Xia, Science, 2019, 366, 850–856 CrossRef CAS PubMed
.
- L. Wang, Z. Zeng, W. Gao, T. Maxson, D. Raciti, M. Giroux, X. Pan, C. Wang and J. Greeley, Science, 2019, 363, 870–874 CrossRef CAS PubMed
.
- C. L. Yang, L. N. Wang, P. Yin, J. Liu, M. X. Chen, Q. Q. Yan, Z. S. Wang, S. L. Xu, S. Q. Chu, C. Cui, H. Ju, J. Zhu, Y. Lin, J. Shui and H. W. Liang, Science, 2021, 374, 459–464 CrossRef CAS PubMed
.
- Q. Zhang, S. Dong, P. Shao, Y. Zhu, Z. Mu, D. Sheng, T. Zhang, X. Jiang, R. Shao, Z. Ren, J. Xie, X. Feng and B. Wang, Science, 2022, 378, 181–186 CrossRef CAS PubMed
.
- X. Zheng, J. Yang, P. Li, Z. Jiang, P. Zhu, Q. Wang, J. Wu, E. Zhang, W. Sun, S. Dou, D. Wang and Y. Li, Angew. Chem., Int. Ed., 2023, 62, e202217449 CrossRef CAS PubMed
.
- T. Wang, X. Cao and L. Jiao, eScience, 2021, 1, 69–74 CrossRef
.
- X. Guo, X. Wan, Q. Liu, Y. Li, W. Li and J. Shui, eScience, 2022, 2, 304–310 CrossRef
.
- J. X. Guo, Y. Zheng, Z. P. Hu, C. Y. Zheng, J. Mao, K. Du, M. Jaroniec, S. Z. Qiao and T. Ling, Nat. Energy, 2023, 8, 264–272 CAS
.
- K. Jiao, J. Xuan, Q. Du, Z. Bao, B. Xie, B. Wang, Y. Zhao, L. Fan, H. Wang, Z. Hou, S. Huo, N. P. Brandon, Y. Yin and M. D. Guiver, Nature, 2021, 595, 361–369 CrossRef CAS PubMed
.
- Y. Wang, Y. Zheng, C. Han and W. Chen, Nano Res., 2020, 14, 1682–1697 CrossRef
.
- H. Wang, C. Tsai, D. Kong, K. Chan, F. Abild-Pedersen, J. K. Nørskov and Y. Cui, Nano Res., 2015, 8, 566–575 CrossRef CAS
.
- Q. He, Y. Wan, H. Jiang, C. Wu, Z. Sun, S. Chen, Y. Zhou, H. Chen, D. Liu, Y. A. Haleem, B. Ge, X. Wu and L. Song, Nano Res., 2018, 11, 1687–1698 CrossRef CAS
.
- M. Hu, J. Zhang, W. Zhu, Z. Chen, X. Gao, X. Du, J. Wan, K. Zhou, C. Chen and Y. Li, Nano Res., 2017, 11, 905–912 CrossRef
.
- Z. W. Seh, J. Kibsgaard, C. F. Dickens, I. Chorkendorff, J. K. Norskov and T. F. Jaramillo, Science, 2017, 355, 146 CrossRef PubMed
.
- Y. Liu, B. Wang, Q. Fu, W. Liu, Y. Wang, L. Gu, D. Wang and Y. Li, Angew. Chem., Int. Ed., 2021, 60, 22522–22528 CrossRef CAS PubMed
.
- Y. Xiong, W. M. Sun, Y. H. Han, P. Y. Xin, X. S. Zheng, W. S. Yan, J. C. Dong, J. Zhang, D. S. Wang and Y. D. Li, Nano Res., 2021, 14, 2418–2423 CrossRef CAS
.
- S. Chen, W. H. Li, W. Jiang, J. Yang, J. Zhu, L. Wang, H. Ou, Z. Zhuang, M. Chen, X. Sun, D. Wang and Y. Li, Angew. Chem., Int. Ed., 2022, 61, e202114450 CAS
.
- Z. Hou, L. Dai, J. Deng, G. Zhao, L. Jing, Y. Wang, X. Yu, R. Gao, X. Tian, H. Dai, D. Wang and Y. Liu, Angew. Chem., Int. Ed., 2022, 61, e202201655 CAS
.
- R. Z. Li and D. S. Wang, Nano Res., 2022, 15, 6888–6923 CrossRef CAS
.
- L. Sun, V. Reddu and X. Wang, Chem. Soc. Rev., 2022, 51, 8923–8956 RSC
.
- Z. Liu, Y. Du, R. Yu, M. Zheng, R. Hu, J. Wu, Y. Xia, Z. Zhuang and D. Wang, Angew. Chem., Int. Ed., 2023, 62, e202212653 CAS
.
- T. Sun, L. Xu, D. Wang and Y. Li, Nano Res., 2019, 12, 2067–2080 CrossRef CAS
.
- W. Zhang, Y. Chao, W. Zhang, J. Zhou, F. Lv, K. Wang, F. Lin, H. Luo, J. Li, M. Tong, E. Wang and S. Guo, Adv. Mater., 2021, 33, e2102576 CrossRef PubMed
.
- P. Zhu, X. Xiong and D. S. Wang, Nano Res., 2022, 15, 5792–5815 CrossRef CAS
.
- J. Wang, C.-X. Zhao, J.-N. Liu, Y.-W. Song, J.-Q. Huang and B.-Q. Li, Nano Energy, 2022, 104, 107927 CrossRef CAS
.
- Y. Pan, C. Zhang, Z. Liu, C. Chen and Y. Li, Matter, 2020, 2, 78–110 CrossRef
.
- H. Rong, S. Ji, J. Zhang, D. Wang and Y. Li, Nat. Commun., 2020, 11, 5884 CrossRef CAS PubMed
.
- S. Mitchell and J. Perez-Ramirez, Nat. Rev. Mater., 2021, 6, 969–985 CrossRef
.
- Z. Zhang, J. Zhu, S. Chen, W. Sun and D. Wang, Angew. Chem., Int. Ed., 2023, 62, e202215136 CrossRef CAS PubMed
.
- Z. Zhuang, L. Xia, J. Huang, P. Zhu, Y. Li, C. Ye, M. Xia, R. Yu, Z. Lang, J. Zhu, L. Zheng, Y. Wang, T. Zhai, Y. Zhao, S. Wei, J. Li, D. Wang and Y. Li, Angew. Chem., Int. Ed., 2023, 62, e202212335 CrossRef CAS PubMed
.
- Y. Wang, J. Wu, S. Tang, J. Yang, C. Ye, J. Chen, Y. Lei and D. Wang, Angew. Chem., Int. Ed., 2023, 62, e202219191 CrossRef CAS PubMed
.
- C. Lu, R. Fang and X. Chen, Adv. Mater., 2020, 32, e1906548 CrossRef PubMed
.
- Z. Zhuang, Q. Kang, D. Wang and Y. Li, Nano Res., 2020, 13, 1856–1866 CrossRef CAS
.
- E. Zhang, L. Tao, J. An, J. Zhang, L. Meng, X. Zheng, Y. Wang, N. Li, S. Du, J. Zhang, D. Wang and Y. Li, Angew. Chem., Int. Ed., 2022, 61, e202117347 CAS
.
- Y. Wang, M. Zheng, Y. Li, C. Ye, J. Chen, J. Ye, Q. Zhang, J. Li, Z. Zhou, X. Z. Fu, J. Wang, S. G. Sun and D. Wang, Angew. Chem., Int. Ed., 2022, 61, e202115735 CAS
.
- X. Li, H. Rong, J. Zhang, D. Wang and Y. Li, Nano Res., 2020, 13, 1842–1855 CrossRef CAS
.
- B. Wang, C. Cheng, M. Jin, J. He, H. Zhang, W. Ren, J. Li, D. Wang and Y. Li, Angew. Chem., Int. Ed., 2022, 61, e202207268 CAS
.
- L. Liu and A. Corma, Chem. Rev., 2018, 118, 4981–5079 CrossRef CAS PubMed
.
- X. Wang, Y. Zhang, J. Wu, Z. Zhang, Q. Liao, Z. Kang and Y. Zhang, Chem. Rev., 2022, 122, 1273–1348 CrossRef CAS PubMed
.
- G. Wang, Y. Wu, Z. Li, Z. Lou, Q. Chen, Y. Li, D. Wang and J. Mao, Angew. Chem., Int. Ed., 2023, 62, e202218460 CAS
.
- N. Zhang, C. Ye, H. Yan, L. Li, H. He, D. Wang and Y. Li, Nano Res., 2020, 13, 3165–3182 CrossRef CAS
.
- H. Su, M. A. Soldatov, V. Roldugin and Q. Liu, eScience, 2022, 2, 102–109 CrossRef
.
- L. Zhang, Y. Ren, W. Liu, A. Wang and T. Zhang, Natl. Sci. Rev., 2018, 5, 653–672 CrossRef CAS
.
- A. Beniya and S. Higashi, Nat. Catal., 2019, 2, 590–602 CrossRef
.
- X. Cui, W. Li, P. Ryabchuk, K. Junge and M. Beller, Nat. Catal., 2018, 1, 385–397 CrossRef CAS
.
- A. Q. Wang, J. Li and T. Zhang, Nat. Rev. Chem., 2018, 2, 65–81 CrossRef CAS
.
- X. Li, L. Liu, X. Ren, J. Gao, Y. Huang and B. Liu, Sci. Adv., 2020, 6, eabb6833 CrossRef CAS PubMed
.
- C. Gao, J. Low, R. Long, T. Kong, J. Zhu and Y. Xiong, Chem. Rev., 2020, 120, 12175–12216 CrossRef CAS PubMed
.
- S. Ji, Y. Chen, X. Wang, Z. Zhang, D. Wang and Y. Li, Chem. Rev., 2020, 120, 11900–11955 CrossRef CAS PubMed
.
- W. H. Li, B. C. Ye, J. Yang, Y. Wang, C. J. Yang, Y. M. Pan, H. T. Tang, D. Wang and Y. Li, Angew. Chem., Int. Ed., 2022, 61, e202209749 CAS
.
- M. F. Li, K. N. Duanmu, C. Z. Wan, T. Cheng, L. Zhang, S. Dai, W. X. Chen, Z. P. Zhao, P. Li, H. L. Fei, Y. M. Zhu, R. Yu, J. Luo, K. T. Zang, Z. Y. Lin, M. N. Ding, J. Huang, H. T. Sun, J. H. Guo, X. Q. Pan, W. A. Goddard, P. Sautet, Y. Huang and X. F. Duan, Nat. Catal., 2019, 2, 495–503 CrossRef CAS
.
- Z. Luo, Y. Ouyang, H. Zhang, M. Xiao, J. Ge, Z. Jiang, J. Wang, D. Tang, X. Cao, C. Liu and W. Xing, Nat. Commun., 2018, 9, 2120 CrossRef PubMed
.
- K. Jiang, B. Liu, M. Luo, S. Ning, M. Peng, Y. Zhao, Y. R. Lu, T. S. Chan, F. M. F. de Groot and Y. Tan, Nat. Commun., 2019, 10, 1743 CrossRef PubMed
.
- Y. Xu, L. Zhu, X. Cui, M. Zhao, Y. Li, L. Chen, W. Jiang, T. Jiang, S. Yang and Y. Wang, Nano Res., 2020, 13, 752–758 CrossRef CAS
.
- J. Yang, W. H. Li, K. Xu, S. Tan, D. Wang and Y. Li, Angew. Chem., Int. Ed., 2022, 61, e202200366 CAS
.
- C.-C. Hou, H.-F. Wang, C. Li and Q. Xu, Energy Environ. Sci., 2020, 13, 1658–1693 RSC
.
- Y. Ying, X. Luo, J. Qiao and H. Huang, Adv. Funct. Mater., 2020, 31, 107927 Search PubMed
.
- Y. Gao, B. Liu and D. Wang, Adv. Mater., 2023 DOI:10.1002/adma.202209654
.
- R. Li and D. Wang, Adv. Energy Mater., 2022, 12, 2103564 CrossRef CAS
.
- W. H. Li, J. Yang and D. Wang, Angew. Chem., Int. Ed., 2022, 61, e202213318 CAS
.
- J. Zhu, M. Xiao, D. Ren, R. Gao, X. Liu, Z. Zhang, D. Luo, W. Xing, D. Su, A. Yu and Z. Chen, J. Am. Chem. Soc., 2022, 144, 9661–9671 CrossRef CAS PubMed
.
- Y. Li, B. Wei, M. Zhu, J. Chen, Q. Jiang, B. Yang, Y. Hou, L. Lei, Z. Li, R. Zhang and Y. Lu, Adv. Mater., 2021, 33, e2102212 CrossRef PubMed
.
- Y. Li, H. Su, S. H. Chan and Q. Sun, ACS Catal., 2015, 5, 6658–6664 CrossRef CAS
.
- Y. Ouyang, L. Shi, X. Bai, Q. Li and J. Wang, Chem. Sci., 2020, 11, 1807–1813 RSC
.
- M. Xiao, Y. Chen, J. Zhu, H. Zhang, X. Zhao, L. Gao, X. Wang, J. Zhao, J. Ge, Z. Jiang, S. Chen, C. Liu and W. Xing, J. Am. Chem. Soc., 2019, 141, 17763–17770 CrossRef CAS PubMed
.
- L. C. Bai, C. S. Hsu, D. T. L. Alexander, H. M. Chen and X. L. Hu, Nat. Energy, 2021, 6, 1054–1066 CrossRef CAS
.
- M. Xiao, J. Zhu, S. Li, G. Li, W. Liu, Y.-P. Deng, Z. Bai, L. Ma, M. Feng, T. Wu, D. Su, J. Lu, A. Yu and Z. Chen, ACS Catal., 2021, 11, 8837–8846 CrossRef CAS
.
- Y. He, X. Yang, Y. Li, L. Liu, S. Guo, C. Shu, F. Liu, Y. Liu, Q. Tan and G. Wu, ACS Catal., 2022, 12, 1216–1227 CrossRef CAS
.
- F. Pan, T. Jin, W. Yang, H. Li, Y. Cao, J. Hu, X. Zhou, H. Liu and X. Duan, Chem. Catal., 2021, 1, 734–745 CrossRef CAS
.
- X. Zeng, J. Shui, X. Liu, Q. Liu, Y. Li, J. Shang, L. Zheng and R. Yu, Adv. Energy Mater., 2018, 8, 1701345 CrossRef
.
- J. Wang, W. Liu, G. Luo, Z. Li, C. Zhao, H. Zhang, M. Zhu, Q. Xu, X. Wang, C. Zhao, Y. Qu, Z. Yang, T. Yao, Y. Li, Y. Lin, Y. Wu and Y. Li, Energy Environ. Sci., 2018, 11, 3375–3379 RSC
.
- J. Wang, Z. Huang, W. Liu, C. Chang, H. Tang, Z. Li, W. Chen, C. Jia, T. Yao, S. Wei, Y. Wu and Y. Li, J. Am. Chem. Soc., 2017, 139, 17281–17284 CrossRef CAS PubMed
.
- G. Yang, J. Zhu, P. Yuan, Y. Hu, G. Qu, B. A. Lu, X. Xue, H. Yin, W. Cheng, J. Cheng, W. Xu, J. Li, J. Hu, S. Mu and J. N. Zhang, Nat. Commun., 2021, 12, 1734 CrossRef CAS PubMed
.
- R. Gao, J. Wang, Z.-F. Huang, R. Zhang, W. Wang, L. Pan, J. Zhang, W. Zhu, X. Zhang, C. Shi, J. Lim and J.-J. Zou, Nat. Energy, 2021, 6, 614–623 CrossRef CAS
.
- Z. Lu, B. Wang, Y. Hu, W. Liu, Y. Zhao, R. Yang, Z. Li, J. Luo, B. Chi, Z. Jiang, M. Li, S. Mu, S. Liao, J. Zhang and X. Sun, Angew. Chem., Int. Ed., 2019, 58, 2622–2626 CrossRef CAS PubMed
.
- A. Han, X. Wang, K. Tang, Z. Zhang, C. Ye, K. Kong, H. Hu, L. Zheng, P. Jiang, C. Zhao, Q. Zhang, D. Wang and Y. Li, Angew. Chem., Int. Ed., 2021, 60, 19262–19271 CrossRef CAS PubMed
.
- T. He, Y. Chen, Q. Liu, B. Lu, X. Song, H. Liu, M. Liu, Y. N. Liu, Y. Zhang, X. Ouyang and S. Chen, Angew. Chem., Int. Ed., 2022, 61, e202201007 CAS
.
- L. Lin, H. Li, C. Yan, H. Li, R. Si, M. Li, J. Xiao, G. Wang and X. Bao, Adv. Mater., 2019, 31, e1903470 CrossRef PubMed
.
- J. Jiao, R. Lin, S. Liu, W. C. Cheong, C. Zhang, Z. Chen, Y. Pan, J. Tang, K. Wu, S. F. Hung, H. M. Chen, L. Zheng, Q. Lu, X. Yang, B. Xu, H. Xiao, J. Li, D. Wang, Q. Peng, C. Chen and Y. Li, Nat. Chem., 2019, 11, 222–228 CrossRef CAS PubMed
.
- Y. Li, W. Shan, M. J. Zachman, M. Wang, S. Hwang, H. Tabassum, J. Yang, X. Yang, S. Karakalos, Z. Feng, G. Wang and G. Wu, Angew. Chem., Int. Ed., 2022, 61, e202205632 CAS
.
- Q. Hao, H.-x Zhong, J.-z Wang, K.-h Liu, J.-m Yan, Z.-h Ren, N. Zhou, X. Zhao, H. Zhang, D.-x Liu, X. Liu, L.-w Chen, J. Luo and X.-b Zhang, Nat. Synth., 2022, 1, 719–728 CrossRef
.
- L. Jiao, J. Zhu, Y. Zhang, W. Yang, S. Zhou, A. Li, C. Xie, X. Zheng, W. Zhou, S. H. Yu and H. L. Jiang, J. Am. Chem. Soc., 2021, 143, 19417–19424 CrossRef CAS PubMed
.
- Z. Chen, Y. Xu, D. Ding, G. Song, X. Gan, H. Li, W. Wei, J. Chen, Z. Li, Z. Gong, X. Dong, C. Zhu, N. Yang, J. Ma, R. Gao, D. Luo, S. Cong, L. Wang, Z. Zhao and Y. Cui, Nat. Commun., 2022, 13, 763 CrossRef CAS PubMed
.
- A. Kumar, V. Q. Bui, J. Lee, L. Wang, A. R. Jadhav, X. Liu, X. Shao, Y. Liu, J. Yu, Y. Hwang, H. T. D. Bui, S. Ajmal, M. G. Kim, S. G. Kim, G. S. Park, Y. Kawazoe and H. Lee, Nat. Commun., 2021, 12, 6766 CrossRef CAS PubMed
.
- L. Zhang, Y. Jia, H. Liu, L. Zhuang, X. Yan, C. Lang, X. Wang, D. Yang, K. Huang, S. Feng and X. Yao, Angew. Chem., Int. Ed., 2019, 58, 9404–9408 CrossRef CAS PubMed
.
- Y. Zhou, E. Song, W. Chen, C. U. Segre, J. Zhou, Y. C. Lin, C. Zhu, R. Ma, P. Liu, S. Chu, T. Thomas, M. Yang, Q. Liu, K. Suenaga, Z. Liu, J. Liu and J. Wang, Adv. Mater., 2020, 32, e2003484 CrossRef PubMed
.
- T. Chao, X. Luo, W. Chen, B. Jiang, J. Ge, Y. Lin, G. Wu, X. Wang, Y. Hu, Z. Zhuang, Y. Wu, X. Hong and Y. Li, Angew. Chem., Int. Ed., 2017, 56, 16047–16051 CrossRef CAS PubMed
.
- Y. Yang, Y. Qian, H. Li, Z. Zhang, Y. Mu, D. Do, B. Zhou, J. Dong, W. Yan, Y. Qin, L. Fang, R. Feng, J. Zhou, P. Zhang, J. Dong, G. Yu, Y. Liu, X. Zhang and X. Fan, Sci. Adv., 2020, 6, eaba6586 CrossRef CAS PubMed
.
- W. Xie, H. Li, G. Cui, J. Li, Y. Song, S. Li, X. Zhang, J. Y. Lee, M. Shao and M. Wei, Angew. Chem., Int. Ed., 2021, 60, 7382–7388 CrossRef CAS PubMed
.
- D. Zhang, W. Chen, Z. Li, Y. Chen, L. Zheng, Y. Gong, Q. Li, R. Shen, Y. Han, W. C. Cheong, L. Gu and Y. Li, Chem. Commun., 2018, 54, 4274–4277 RSC
.
- D. Liu, B. Wang, H. Li, S. Huang, M. Liu, J. Wang, Q. Wang, J. Zhang and Y. Zhao, Nano Energy, 2019, 58, 277–283 CrossRef CAS
.
- N. Zhang, X. Zhang, Y. Kang, C. Ye, R. Jin, H. Yan, R. Lin, J. Yang, Q. Xu, Y. Wang, Q. Zhang, L. Gu, L. Liu, W. Song, J. Liu, D. Wang and Y. Li, Angew. Chem., Int. Ed., 2021, 60, 13388–13393 CrossRef CAS PubMed
.
- S. Tian, B. Wang, W. Gong, Z. He, Q. Xu, W. Chen, Q. Zhang, Y. Zhu, J. Yang, Q. Fu, C. Chen, Y. Bu, L. Gu, X. Sun, H. Zhao, D. Wang and Y. Li, Nat. Commun., 2021, 12, 3181 CrossRef CAS PubMed
.
- L. Zhang, R. Si, H. Liu, N. Chen, Q. Wang, K. Adair, Z. Wang, J. Chen, Z. Song, J. Li, M. N. Banis, R. Li, T. K. Sham, M. Gu, L. M. Liu, G. A. Botton and X. Sun, Nat. Commun., 2019, 10, 4936 CrossRef PubMed
.
- H. Yan, Y. Lin, H. Wu, W. Zhang, Z. Sun, H. Cheng, W. Liu, C. Wang, J. Li, X. Huang, T. Yao, J. Yang, S. Wei and J. Lu, Nat. Commun., 2017, 8, 1070 CrossRef PubMed
.
- L. Bai, C. S. Hsu, D. T. L. Alexander, H. M. Chen and X. Hu, J. Am. Chem. Soc., 2019, 141, 14190–14199 CrossRef CAS PubMed
.
- L. Zhang, J. Fischer, Y. Jia, X. Yan, W. Xu, X. Wang, J. Chen, D. Yang, H. Liu, L. Zhuang, M. Hankel, D. J. Searles, K. Huang, S. Feng, C. L. Brown and X. Yao, J. Am. Chem. Soc., 2018, 140, 10757–10763 CrossRef CAS PubMed
.
- Y. Zhao, X. Yan, K. R. Yang, S. Cao, Q. Dong, J. E. Thorne, K. L. Materna, S. Zhu, X. Pan, M. Flytzani-Stephanopoulos, G. W. Brudvig, V. S. Batista and D. Wang, ACS Cent. Sci., 2018, 4, 1166–1172 CrossRef CAS PubMed
.
- Y. Zhao, K. R. Yang, Z. Wang, X. Yan, S. Cao, Y. Ye, Q. Dong, X. Zhang, J. E. Thorne, L. Jin, K. L. Materna, A. Trimpalis, H. Bai, S. C. Fakra, X. Zhong, P. Wang, X. Pan, J. Guo, M. Flytzani-Stephanopoulos, G. W. Brudvig, V. S. Batista and D. Wang, Proc. Natl. Acad. Sci. U. S. A., 2018, 115, 2902–2907 CrossRef CAS PubMed
.
- Z. Li, Y. Chen, S. Ji, Y. Tang, W. Chen, A. Li, J. Zhao, Y. Xiong, Y. Wu, Y. Gong, T. Yao, W. Liu, L. Zheng, J. Dong, Y. Wang, Z. Zhuang, W. Xing, C.-T. He, C. Peng, W.-C. Cheong, Q. Li, M. Zhang, Z. Chen, N. Fu, X. Gao, W. Zhu, J. Wan, J. Zhang, L. Gu, S. Wei, P. Hu, J. Luo, J. Li, C. Chen, Q. Peng, X. Duan, Y. Huang, X.-M. Chen, D. Wang and Y. Li, Nat. Chem., 2020, 12, 764–772 CrossRef CAS PubMed
.
- Y. Xiong, J. Dong, Z. Q. Huang, P. Xin, W. Chen, Y. Wang, Z. Li, Z. Jin, W. Xing, Z. Zhuang, J. Ye, X. Wei, R. Cao, L. Gu, S. Sun, L. Zhuang, X. Chen, H. Yang, C. Chen, Q. Peng, C. R. Chang, D. Wang and Y. Li, Nat. Nanotechnol., 2020, 15, 390–397 CrossRef CAS PubMed
.
- T. Cui, Y. P. Wang, T. Ye, J. Wu, Z. Chen, J. Li, Y. Lei, D. Wang and Y. Li, Angew. Chem., Int. Ed., 2022, 61, e202115219 CAS
.
- M. Liu, N. Li, S. Cao, X. Wang, X. Lu, L. Kong, Y. Xu and X. H. Bu, Adv. Mater., 2022, 34, e2107421 CrossRef PubMed
.
- D. Yu, Y. Ma, F. Hu, C. C. Lin, L. Li, H. Y. Chen, X. Han and S. Peng, Adv. Energy Mater., 2021, 11, 2101242 CrossRef CAS
.
- Y. S. Wei, L. Sun, M. Wang, J. Hong, L. Zou, H. Liu, Y. Wang, M. Zhang, Z. Liu, Y. Li, S. Horike, K. Suenaga and Q. Xu, Angew. Chem., Int. Ed., 2020, 59, 16013–16022 CrossRef CAS PubMed
.
- X. B. Zheng, B. B. Li, Q. S. Wang, D. S. Wang and Y. D. Li, Nano Res., 2022, 15, 7806–7839 CrossRef CAS
.
- X. Zheng, P. Li, S. Dou, W. Sun, H. Pan, D. Wang and Y. Li, Energy Environ. Sci., 2021, 14, 2809 RSC
.
- J. Yang, W. Li, D. Wang and Y. Li, Adv. Mater., 2020, 32, e2003300 CrossRef PubMed
.
- J. Gu, M. Jian, L. Huang, Z. Sun, A. Li, Y. Pan, J. Yang, W. Wen, W. Zhou, Y. Lin, H.-J. Wang, X. Liu, L. Wang, X. Shi, X. Huang, L. Cao, S. Chen, X. Zheng, H. Pan, J. Zhu, S. Wei, W.-X. Li and J. Lu, Nat. Nanotechnol., 2021, 16, 1141–1149 CrossRef CAS PubMed
.
- D. Zhao, Z. Zhuang, X. Cao, C. Zhang, Q. Peng, C. Chen and Y. Li, Chem. Soc. Rev., 2020, 49, 2215–2264 RSC
.
- L. Wu, T. Guo and T. Li, Adv. Funct. Mater., 2022, 32, 2203439 CrossRef CAS
.
- H. Sun, C. W. Tung, Y. Qiu, W. Zhang, Q. Wang, Z. Li, J. Tang, H. C. Chen, C. Wang and H. M. Chen, J. Am. Chem. Soc., 2022, 144, 1174–1186 CrossRef CAS PubMed
.
- Y. Yang, S. Louisia, S. Yu, J. Jin, I. Roh, C. Chen, M. V. Fonseca Guzman, J. Feijoo, P. C. Chen, H. Wang, C. J. Pollock, X. Huang, Y. T. Shao, C. Wang, D. A. Muller, H. D. Abruna and P. Yang, Nature, 2023, 614, 262–269 CrossRef CAS PubMed
.
- W. Wan, Y. Zhao, S. Wei, C. A. Triana, J. Li, A. Arcifa, C. S. Allen, R. Cao and G. R. Patzke, Nat. Commun., 2021, 12, 5589 CrossRef CAS PubMed
.
- H. Jing, P. Zhu, X. Zheng, Z. Zhang, D. Wang and Y. Li, Adv. Powder Mater., 2022, 1, 100013 CrossRef
.
- H. Y. Zhuo, X. Zhang, J. X. Liang, Q. Yu, H. Xiao and J. Li, Chem. Rev., 2020, 120, 12315–12341 CrossRef CAS PubMed
.
- X. Zhou, J. Gao, Y. Hu, Z. Jin, K. Hu, K. M. Reddy, Q. Yuan, X. Lin and H. J. Qiu, Nano Lett., 2022, 22, 3392–3399 CrossRef CAS PubMed
.
- X. Zhu, J. Yan, M. Gu, T. Liu, Y. Dai, Y. Gu and Y. Li, J. Phys. Chem. Lett., 2019, 10, 7760–7766 CrossRef CAS PubMed
.
- H. N. Nong, L. J. Falling, A. Bergmann, M. Klingenhof, H. P. Tran, C. Spori, R. Mom, J. Timoshenko, G. Zichittella, A. Knop-Gericke, S. Piccinin, J. Perez-Ramirez, B. R. Cuenya, R. Schlogl, P. Strasser, D. Teschner and T. E. Jones, Nature, 2020, 587, 408–413 CrossRef CAS PubMed
.
- J. T. Mefford, A. R. Akbashev, M. Kang, C. L. Bentley, W. E. Gent, H. D. Deng, D. H. Alsem, Y. S. Yu, N. J. Salmon, D. A. Shapiro, P. R. Unwin and W. C. Chueh, Nature, 2021, 593, 67–73 CrossRef CAS PubMed
.
- X. Wang, S. Xi, P. Huang, Y. Du, H. Zhong, Q. Wang, A. Borgna, Y. W. Zhang, Z. Wang, H. Wang, Z. G. Yu, W. S. V. Lee and J. Xue, Nature, 2022, 611, 702–708 CrossRef CAS PubMed
.
- X. Zheng, P. Cui, Y. Qian, G. Zhao, X. Zheng, X. Xu, Z. Cheng, Y. Liu, S. X. Dou and W. Sun, Angew. Chem., Int. Ed., 2020, 59, 14533–14540 CrossRef CAS PubMed
.
- X. Zheng, Y. Chen, X. Zheng, G. Zhao, K. Rui, P. Li, X. Xu, Z. Cheng, S. X. Dou and W. Sun, Adv. Energy Mater., 2019, 9, 1803482 CrossRef
.
- X. Zheng, Y. Chen, W. Lai, P. Li, C. Ye, N. Liu, S. X. Dou, H. Pan and W. Sun, Adv. Funct. Mater., 2022, 32, 2200663 CrossRef CAS
.
- G. Zhao, Z. Luo, B. Zhang, Y. Chen, X. Cui, J. Chen, Y. Liu, M. Gao, H. Pan and W. Sun, Nano Res., 2023, 16, 4767–4774 CrossRef CAS
.
- B. Hu, A. Huang, X. Zhang, Z. Chen, R. Tu, W. Zhu, Z. Zhuang, C. Chen, Q. Peng and Y. Li, Nano Res., 2021, 14, 3482–3488 CrossRef CAS
.
- C. Wu, X. Zhang, Z. Xia, M. Shu, H. Li, X. Xu, R. Si, A. I. Rykov, J. Wang, S. Yu, S. Wang and G. Sun, J. Mater. Chem. A, 2019, 7, 14001–14010 RSC
.
- Z. Pei, X. F. Lu, H. Zhang, Y. Li, D. Luan and X. W. D. Lou, Angew. Chem., Int. Ed., 2022, 61, e202207537 CAS
.
- X. Zheng, J. Yang, Z. Xu, Q. Wang, J. Wu, E. Zhang, S. Dou, W. Sun, D. Wang and Y. Li, Angew. Chem., Int. Ed., 2022, 61, e202205946 CAS
.
- S. Li, B. Chen, Y. Wang, M. Y. Ye, P. A. van Aken, C. Cheng and A. Thomas, Nat. Mater., 2021, 20, 1240–1247 CrossRef CAS PubMed
.
- Z. Li, H. He, H. Cao, S. Sun, W. Diao, D. Gao, P. Lu, S. Zhang, Z. Guo, M. Li, R. Liu, D. Ren, C. Liu, Y. Zhang, Z. Yang, J. Jiang and G. Zhang, Appl. Catal., B, 2019, 240, 112–121 CrossRef CAS
.
- J. Chen, H. Li, C. Fan, Q. Meng, Y. Tang, X. Qiu, G. Fu and T. Ma, Adv. Mater., 2020, 32, e2003134 CrossRef PubMed
.
- X. Zhu, D. Zhang, C.-J. Chen, Q. Zhang, R.-S. Liu, Z. Xia, L. Dai, R. Amal and X. Lu, Nano Energy, 2020, 71, 104597 CrossRef CAS
.
- Y. Wang, Z. Li, P. Zhang, Y. Pan, Y. Zhang, Q. Cai, S. R. P. Silva, J. Liu, G. Zhang, X. Sun and Z. Yan, Nano Energy, 2021, 87, 106147 CrossRef CAS
.
- Z. Zeng, L. Y. Gan, H. Bin Yang, X. Su, J. Gao, W. Liu, H. Matsumoto, J. Gong, J. Zhang, W. Cai, Z. Zhang, Y. Yan, B. Liu and P. Chen, Nat. Commun., 2021, 12, 4088 CrossRef CAS PubMed
.
|
This journal is © The Royal Society of Chemistry 2023 |
Click here to see how this site uses Cookies. View our privacy policy here.