DOI:
10.1039/D0RA05217E
(Paper)
RSC Adv., 2020,
10, 25165-25169
Iodine-catalyzed efficient synthesis of xanthene/thioxanthene-indole derivatives under mild conditions†
Received
13th June 2020
, Accepted 26th June 2020
First published on 2nd July 2020
Abstract
An iodine-catalyzed nucleophilic substitution reaction of xanthen-9-ol and thioxanthen-9-ol with indoles has been developed, providing an efficient procedure for the synthesis of xanthene/thioxanthene-indole derivatives with good to excellent yields. This protocol offers several advantages, such as short reaction times, green solvent, operational simplicity, easily available catalyst and mild reaction conditions. Moreover, this method showed good tolerance of functional groups and a wide range of substrates.
Introduction
Xanthenes and their analogues including thioxanthenes are important biologically active heterocycles.1 Natural and synthetic products possessing xanthene moieties exhibit a wide range of biological activities such as antitumor,2 antibacterial,3 antifungal,4 analgesic and antiinflammatory5 activities. Presently, a number of methods for the functionalized xanthene derivatives have been investigated, some methods particularly for the synthesis of 9-substituted xanthene derivatives.6 Notably, substitution of the xanthenyl-9-position with an indolyl substituent has received much attention due to the widespread biological and pharmacological activities of indole derivatives.7 Recently, xanthene-indole derivatives were explored by the nucleophilic substitution of xanthen-9-ol in the presence of catalysts such as AcOH,8 DDQ,9 CAN,10 In(DS)3,11 BmimBF4,12 gluconic acid13 and 1,3-dichloro-tetra-n-butyl-distannoxane.14 Cozzi also reported the direct substitution of xanthen-9-ol ‘on water’ without Brønsted or Lewis acid or surfactants, but this reaction must be carried out at 80 °C for long time.15 However, many of these procedures suffer from certain drawbacks including strong acidic conditions, long reaction times, low yields of products and harsh reaction conditions. In addition, Tsuchimoto reported an indium-catalyzed reductive alkylation of indoles by using carbonyl compounds to obtain alkylindoles with structural diversity.7d However, this method was not used in the formation of xanthene-indole derivatives. Therefore, there is considerable interest in exploring superior catalyst systems which are cheap, stable, environmentally friendly and easy to access.
In recent years, molecular iodine has drawn considerable attention, and been used frequently in many organic reactions. It is widely available, nontoxic, relatively inexpensive, environmentally friendly and highly stable to air and moisture.16 Iodine catalyzed reactions have resulted in the formation of new C–C,17 C–O,18 C–N (ref. 19) and C–S (ref. 20) bonds, and have been recognized as a powerful tool for the synthesis of pharmacologically important heterocyclic compounds.21 Several methodologies have been reported for the reactions involving the iodine-mediated nucleophilic addition of indole to aldehydes and ketones.22 However, the reactions about iodine-mediated nucleophilic substitution of alcohols with indoles are seldom.
With these in our mind, we report an efficient synthesis of xanthene/thioxanthene-indole derivatives by iodine-catalyzed nucleophilic substitution of alcohols with indoles under mild conditions in a very short time (Scheme 1). Moreover, this protocol was also used in the substitution of secondary alcohols with indoles or other nucleophiles such as pyrrole, furan and 1,3-dicarbonyl compounds. To the best of our knowledge, there are no reports concerning the iodine employed in the substitution of xanthen-9-ol or thioxanthen-9-ol.
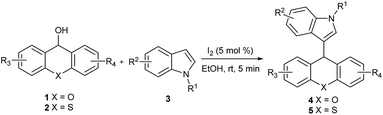 |
| Scheme 1 I2-catalyzed synthesis of xanthene/thioxanthene-indole derivatives. | |
Results and discussion
The reaction of 9H-xanthen-9-ol 1a and indole 3a was chosen as a model reaction to optimize the reaction conditions. The results were listed in the Table 1. Initially, we investigated the reaction at room temperature under different amounts of iodine in CH3CN. In the absence of iodine, the reaction could not proceed in CH3CN at room temperature even prolonging reaction time to 1 h (entry 1). To our delight, the reaction proceeded rapidly in the presence of iodine to afford the corresponding substituted product in good yield (entries 2–4), and the investigation indicated that the optimal amount of catalyst was 5 mol% in 85% yield (entry 2). Next, different solvents were also examined in the presence of 5 mol% I2. Interestingly, EtOH and CH2Cl2 are tolerated in this reaction (entries 5 and 6). Only H2O is turned out to be incompatible for the poor solubility of 1a in H2O (entry 7). Different oxidizing agents including NBS and tBuOOH were also examined, but did not show any improvement in the generation of 4aa. Therefore, the reaction with 5 mol% I2 in EtOH at room temperature for 5 minutes was found to be the optimal condition.
Table 1 Optimization of the reaction conditionsa
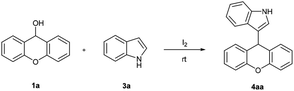
|
Entry |
Catalyst (mol%) |
Solvent |
Time (min) |
Yieldb (%) |
Reaction conditions: 1a (1.0 mmol) and 3a (1.0 mmol) in solvent (5 mL) at rt under air. Isolated yield. Not detected. Reaction temperature was 50 °C. |
1 |
I2 (0) |
CH3CN |
60 |
n.d.c |
2 |
I2 (5) |
CH3CN |
5 |
85 |
3 |
I2 (10) |
CH3CN |
5 |
81 |
4 |
I2 (15) |
CH3CN |
5 |
80 |
5 |
I2 (5) |
EtOH |
5 |
87 |
6 |
I2 (5) |
CH2Cl2 |
5 |
77 |
7 |
I2 (5) |
H2O |
5 |
n.d. |
8 |
I2 (5) |
EtOH |
30 |
86 |
9d |
I2 (5) |
EtOH |
5 |
84 |
10 |
NBS (5) |
EtOH |
5 |
84 |
11 |
tBuOOH (5) |
EtOH |
60 |
82 |
Under the above optimum reaction conditions, we investigated the substrate scope of the iodine-catalyzed nucleophilic substitution of xanthen-9-ol. Firstly, xanthen-9-ol 1 with different indoles were investigated, and the representative results were listed in Table 2. All substituted indoles with electronic-withdrawing groups or electronic-donating groups proceeded smoothly to afford the desired xanthene-indole derivatives with high or excellent yield. Surprisingly, not only 1- or 2-substituted indoles but also 3-, 4-, 5- or 6-substituted indoles showed high reactivity in our methodology. Moreover, for methyl 1H-indole-2-carboxylate, the desired product could be afforded with 90% yield. Substitution of xanthen-9-ol with different substituents such as halogen atoms, CH3, CH3O, CH3CH2O and NO2 groups was conducted, and the targeted products were obtained in good yields.
Table 2 Scope of the reaction of indoles 3 with xanthen-9-ol 1a,b
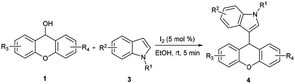
|
Reaction conditions: 1 (1.0 mmol) and 3 (1.0 mmol) in EtOH (5 mL) at rt under air. Isolated yield. The product was recrystallized from petroleum ether and ethyl acetate. |
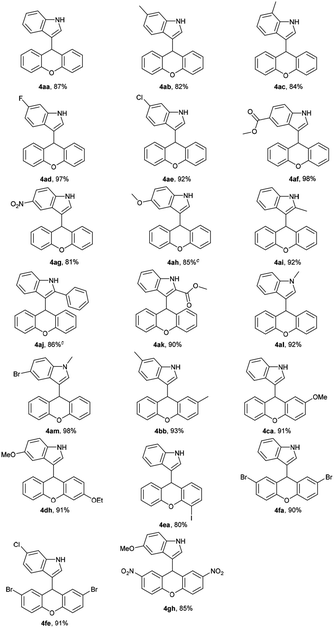 |
Subsequently, the reactivity of 9H-thioxanthen-9-ol 2 with indoles was also tested for synthesis of thioxanthene-indole derivatives. As shown in Table 3, the reaction proceeded smoothly and afforded corresponding substituted product with high yield.
Table 3 Scope of the reaction of indoles 3 with 9H-thioxanthen-9-ol 2a,b
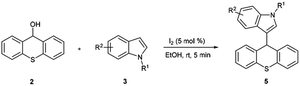
|
Reaction conditions: freshly prepared 2 (1.0 mmol) and 3 (1.0 mmol) in EtOH (5 mL) at rt under air. Isolated yield. |
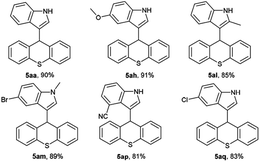 |
Furthermore, other nucleophiles such as pyrrole, furan and 1,3-dicarbonyl compounds were examined (Table 4). Interestingly, the use of 0.5 equiv. of pyrrole or furan gave 2,5-disubstituted pyrrole or 2,5-disubstituted furan in good yields. However, the use of 8 equiv. of pyrrole or furan afforded 2-substituted pyrrole (83%) or 2-substituted furan (81%). 1,3-dicarbonyl compounds such as 2,4-pentanedione, dimedone, 1,3-indanedione, 1,3-dimethylbarbituric acid and 4-hydroxycoumarin were found to be compatible with the reaction conditions, giving the desired products with good to excellent yields.
Table 4 Scope of the reaction of other nucleophiles with xanthen-9-ol 1a,b
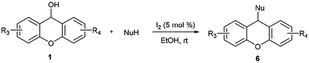
|
Reaction conditions: 1 (1.0 mmol) and NuH (1.0 mmol) in EtOH (5 mL) at rt under air. Isolated yield. The reaction was performed with 0.5 equiv. of pyrrole or furan. The reaction was performed with 8 equiv. of pyrrole or furan. The product was recrystallized from petroleum ether and ethyl acetate. |
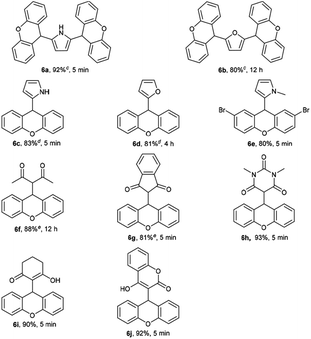 |
In addition, the reactivity of other secondary alcohols including benzhydrols and ferrocenyl alcohols were also investigated (Table 5). Diphenylmethanol was not reactive even prolonging reaction time to 12 h. Bis(4-methoxyphenyl)methanol and bis(4-(dimethylamino)phenyl)methanol were suitable substrates and the corresponding products 8b and 8d could be obtained in 99% and 84% respectively. The substitution of bis(4-(dimethylamino)phenyl)methanol with pyrrole (0.5 equiv.) also proceeded smoothly to give 2,5-disubstituted pyrrole in 80% yield. To our delight, the heterocyclic derived substrates such as di(benzofuran-2-yl)methanol, benzofuran-2-yl(4-methoxyphenyl)methanol and (9-ethyl-9H-carbazol-3-yl)(phenyl)methanol could proceed smoothly in this reaction, affording the desired products with excellent yields. Ferrocenyl alcohols were also good candidate in this transformation to give the desired products 8j (92%) and 8k (98%).
Table 5 Scope of the reaction of other secondary alcoholsa,b
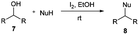
|
Reaction conditions: 7 (1.0 mmol) and NuH (1.0 mmol) in EtOH (5 mL) at rt under air. Isolated yield. The reaction was performed with 0.5 equiv. of pyrrole. The product was recrystallized from petroleum ether and ethyl acetate. |
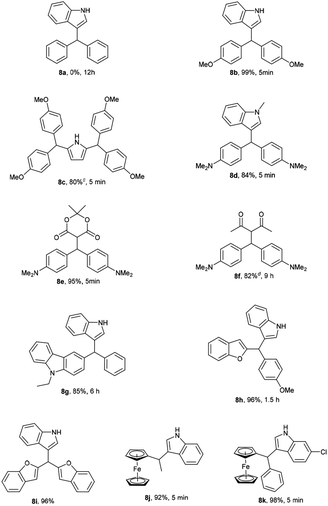 |
The structures of synthesized compounds were confirmed on the basis of IR, 1H, 13C NMR spectra as well as by comparison with previous reports. For example, compound 4aa was obtained as white solid. Its molecular formula was determined to be C21H15NO from the HR-MS at m/z 320.1046 [M + Na]+ (calcd 320.1047). Absorption bands at 3425 cm−1 in the IR spectrum of 4aa were assigned to N–H of indolyl unit. The 1H NMR data of 4aa indicated the presence of a 3-substituted indolyl unit based on the characteristic signals at δH 7.92 (s, 1H), 7.35 (d, J = 8.0 Hz, 1H), 7.30 (d, J = 8.0 Hz, 1H), 7.18 (dd, J = 8.8, 1.6 Hz, 1H), 7.03 (d, J = 2.4 Hz, 1H) and 6.97–6.94 (m, 1H). The 13C NMR data of 4aa showed 15 carbon signals. δC 151.33 (2 C), 129.50 (2 CH), 127.73 (2 CH), 124.44 (2 C), 123.13 (2 CH), 116.35 (2 CH) and 35.53 (CH) suggested the existence of 9-substituted xanthenyl moiety.
In order to probe the mechanism of this reaction, control experiments were further conducted (Scheme 2). A 9-ethoxy-9H-xanthene 6k was obtained when 9H-xanthen-9-ol 1a was treated solely under the optimized reaction conditions. Subsequently, 9-ethoxy-9H-xanthene was reacted with indole 3a under the standard conditions; 86% of 4aa was obtained (Scheme 2b). However, when 9H-xanthen-9-ol 1a was treated solely under I2 (5% mmol) in CH3CN, 9H-xanthen-9-one and 9H-xanthene formed immediately (Scheme 2c). Meanwhile, the reaction of bis(3-methoxyphenyl)methanol with indole could not proceed under the optimized reaction conditions from room temperature to reflux even prolonging reaction time to 12 h (Scheme 2d).
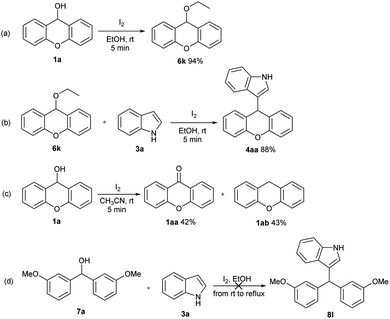 |
| Scheme 2 Control experiments. | |
On the basis of the above experimental outcomes and the previous literature reports,11,22 a plausible reaction mechanism is presented in Scheme 3. The first step of the process is the coordination of iodine to the hydroxyl function of compound A, thus causing its elimination to give the pyrylium ion B. And the I2 is transformed to HIO and I−. Then, nucleophile attacks the pyrylium ion to generate the corresponding product. On the other hand, EtOH as nucleophile can react with pyrylium ion B to give C, which can transform to pyrylium ion under HI. Importantly, the formation of C can prevent the redox reaction between A and pyrylium ion B. Liberated HI is further reacted with HIO to regenerate I2 and complete the catalytic cycle by releasing H2O. For other secondary alcohols, the results could be explained by the higher stabilization of the generated carbocation.
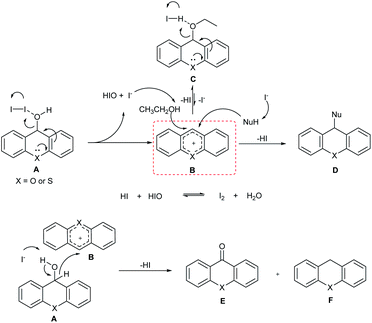 |
| Scheme 3 Proposed mechanisms. | |
Conclusions
In conclusion, I2 in ethanol was found to be effective and green catalyst for the electrophilic substitution reactions of xanthen-9-ol and thioxanthen-9-ol with indoles giving xanthene/thioxanthene-indole derivatives. Using 5 mol% I2, the reactions could proceed efficiently at room temperature in five minutes and afford xanthene/thioxanthene-indole derivatives with good to excellent yield. Moreover, the reactions of xanthen-9-ol with other nucleophilic reagents such as pyrrole, furan and 1,3-dicarbonyl compounds could proceed smoothly. The scope of substrate was also extended to other secondary alcohols such as benzhydrols and ferrocenyl alcohols.
Conflicts of interest
There are no conflicts to declare.
Acknowledgements
This work was supported by the National Natural Science Foundation of China (No. 21602050), Applied Basic Research Project of Yunnan (2017FD154), and Yunnan Province Department of Education Fund (2016ZZX218).
Notes and references
-
(a) M. R. Taghartapeh, N. N. Pesyan, H. Rashidnejad, H. R. Khavasi and A. Soltani, J. Mol. Struct., 2017, 1149, 862 CrossRef CAS;
(b) L. Jeyaseeli, A. D. Gupta, K. A. Kumar, K. Mazumdar, N. K. Dutta and S. G. Dastidar, Int. J. Antimicrob. Agents, 2006, 27, 58 CrossRef CAS PubMed;
(c) T. Yıldız and H. B. Kücük, RSC Adv., 2017, 7, 16644 RSC.
- S. Y. Wu, Y. H. Fu, G. Y. Chen, X. B. Li, Q. Zhou, C. R. Han, X. P. Song, X. J. Du, M. L. Xie and G. G. Yao, Phytochem. Lett., 2015, 11, 236 CrossRef CAS.
-
(a) S. Naseem, M. Khalid, M. N. Tahir, M. A. Halim, A. A. C. Braga, M. M. Naseer and Z. Shafia, J. Mol. Struct., 2017, 1143, 235 CrossRef CAS;
(b) A. Barmak, K. Niknam, G. Mohebbi and H. Pournabi, Microb. Pathog., 2019, 130, 95 CrossRef CAS PubMed;
(c) A. Akbari and A. Hosseini-Nia, J. Saudi Chem. Soc., 2017, 21, S7 CrossRef CAS.
-
(a) J. M. Khurana, D. Magoo, K. Aggarwal, N. Aggarwal, R. Kumar and C. Srivastava, Eur. J. Med. Chem., 2012, 58, 470 CrossRef CAS PubMed;
(b) U. Kusampally, R. Pagadala and C. R. Kamatala, Tetrahedron Lett., 2017, 58, 3316 CrossRef CAS.
-
(a) H. N. Hafez, M. I. Hegab, I. S. Ahmed-Farag and A. B. A. El-Gazzar, Bioorg. Med. Chem. Lett., 2008, 18, 4538 CrossRef CAS PubMed;
(b) A. G. Banerjee, L. P. Kothapalli, P. A. Sharma, A. B. Thomas, R. K. Nanda, S. K. Shrivastava and V. V. Khatanglekar, Arabian J. Chem., 2016, 9, S480 CrossRef CAS.
-
(a) C. G. Piscopo, S. Bühler, G. Sartori and R. Maggi, Catal. Sci. Technol., 2012, 2, 2449 RSC;
(b) H. Jian, K. Liu, W. H. Wang, Z. J. Li, B. Dai and L. He, Tetrahedron Lett., 2017, 58, 1137 CrossRef CAS;
(c) Q. Chen, G. D. Yu, X. F. Wang, Y. C. Ou and Y. P. Huo, Green Chem., 2019, 21, 798 RSC;
(d) Subodh, N. K. Mogha, K. Chaudhary, G. Kumar and D. T. Masram, ACS Omega, 2018, 3, 16377 CrossRef CAS PubMed;
(e) Q. Chen, X. F. Wang, G. D. Yu, C. X. Wen and Y. P. Huo, Org. Chem. Front., 2018, 5, 2652 RSC.
-
(a) F. Peng, S. Y. Hou, T. Y. Zhang, Y. Y. Wu, M. Y. Zhang, X. M. Yan, M. Y. Xia and Y. X. Zhang, RSC Adv., 2019, 9, 28754 RSC;
(b) A. Kumari and R. K. Singh, Bioorg. Chem., 2019, 89, 103021 CrossRef CAS PubMed;
(c) P. V. Thanikachalam, R. K. Maurya, V. Garg and V. Monga, Eur. J. Med. Chem., 2019, 180, 562 CrossRef CAS PubMed;
(d) S. Nomiyama, T. Hondo and T. Tsuchimoto, Adv. Synth. Catal., 2016, 358, 1136 CrossRef CAS.
- R. Deschner and U. Pindur, J. Heterocycl. Chem., 1984, 21, 1485 CrossRef CAS.
- J. Bergman, R. Carlsson and S. Misztal, Acta Chem. Scand., Ser. B, 1976, 30, 853 CrossRef.
- S. Y. Wang and S. J. Ji, Synlett, 2007, 14, 2222 Search PubMed.
- S. Y. Wang and S. J. Ji, Synth. Commun., 2008, 38, 465 CrossRef CAS.
- L. Y. Liu, B. Wang, H. M. Yang, W. X. Chang and J. Li, Tetrahedron Lett., 2011, 52, 5636 CrossRef CAS.
- B. H. Zhou, J. Yang, M. H. Li and Y. L. Gu, Green Chem., 2011, 13, 2204 RSC.
- L. Y. Liu, Y. Zhang, K. M. Huang, W. X. Chang and J. Li, Appl. Organomet. Chem., 2012, 26, 9 CrossRef CAS.
- P. G. Cozzi and L. Zoli, Angew. Chem., Int. Ed., 2008, 47, 4162 CrossRef CAS PubMed.
-
(a) M. S. Yusubov and V. V. Zhdankin, Resour.-Effic. Technol., 2015, 1, 49 Search PubMed;
(b) S. K. Samanta and M. K. Bera, Org. Biomol. Chem., 2019, 17, 6441 RSC;
(c) K. M. Saini, R. K. Saunthwal, S. Kumar and A. K. Verma, Org. Biomol. Chem., 2019, 17, 2657 RSC;
(d) J. S. Yadav, B. V. S. Reddy, C. V. Rao, P. K. Chand and A. R. Prasad, Synlett, 2001, 1638 CrossRef CAS;
(e) J. S. Yadav, B. V. S. Reddy, K. Premalatha and T. Swamy, Tetrahedron Lett., 2005, 46, 2687 CrossRef CAS.
-
(a) S. Akbar, V. J. Tamilarasan and K. Srinivasan, RSC Adv., 2019, 9, 23652 RSC;
(b) J. J. Koenig, T. Arndt, N. Gildemeister, J. M. Neudörfl and M. Breugst, J. Org. Chem., 2019, 84, 7587 CrossRef CAS PubMed.
-
(a) T. Duhamel and K. Muñiz, Chem. Commun., 2019, 55, 933 RSC;
(b) C. Chen, W. B. Liu, P. Zhou and H. L. Liu, RSC Adv., 2017, 7, 20394 RSC;
(c) H. M. S. Kumar, B. V. S. Reddy, E. J. Reddy and J. S. Yadav, Chem. Lett., 1999, 28, 857 CrossRef.
-
(a) X. S. Wang, J. Sheng, L. Lu, K. Yang and Y. L. Li, ACS Comb. Sci., 2011, 13, 196 CrossRef CAS PubMed;
(b) Z. G. Zhang, X. Li, Y. H. Li, Y. Guo, X. N. Zhao, Y. Yan, K. Sun and G. S. Zhang, Tetrahedron, 2019, 75, 3628 CrossRef CAS;
(c) S. Wangngae, C. Duangkamol, M. Pattarawarapan and W. Phakhodee, RSC Adv., 2015, 5, 25789 RSC;
(d) H. W. Zhang and K. Muñiz, ACS Catal., 2017, 7, 4122 CrossRef CAS;
(e) R. Varala, S. Nuvula and S. R. Adapa, J. Org. Chem., 2006, 71, 8283 CrossRef CAS PubMed.
-
(a) Y. C. Gao, Z. B. Huang, L. Xu, Z. D. Li, Z. S. Lai and R. Y. Tang, Org. Biomol. Chem., 2019, 17, 2279 RSC;
(b) Y. W. Liu, S. S. Badsara, Y. C. Liu and C. F. Lee, RSC Adv., 2015, 5, 44299 RSC;
(c) H. L. Zhang, X. Z. Bao, Y. M. Song, J. P. Qu and B. M. Wang, Tetrahedron, 2015, 71, 8885 CrossRef CAS.
-
(a) T. Ishikawa, M. Kimura, T. Kumoi and H. Iida, ACS Catal., 2017, 7, 4986 CrossRef CAS;
(b) S. K. Samanta and M. K. Bera, Org. Biomol. Chem., 2019, 17, 6441 RSC;
(c) C. Wang, X. Geng, P. Zhao, Y. Zhou, Y. D. Wu, Y. F. Cui and A. X. Wu, Chem. Commun., 2019, 55, 8134 RSC;
(d) Q. H. Gao, X. Wu, S. Liu and A. X. Wu, Org. Lett., 2014, 16, 1732 CrossRef CAS PubMed;
(e) G. S. Mani, K. Donthiboina, S. P. Shaik, N. Shankaraiah and A. Kamal, RSC Adv., 2019, 9, 27021 RSC;
(f) J. Wu, H. G. Xia and K. Gao, Org. Biomol. Chem., 2006, 4, 126 RSC;
(g) M. J. Mphahlele, Molecules, 2009, 14, 4814 CrossRef CAS PubMed;
(h) N. Mulakayala, P. V. N. S. Murthy, D. Rambabu, M. Aeluri, R. Adepu, G. R. Krishna, C. M. Reddy, K. R. S. Prasad, M. Chaitanya, C. S. Kumar, M. V. B. Rao and M. Pal, Bioorg. Med. Chem. Lett., 2012, 22, 2186 CrossRef CAS PubMed;
(i) G. R. Reddy, T. R. Reddy, S. C. Joseph, K. S. Reddy and M. Pal, RSC Adv., 2012, 2, 3387 RSC.
-
(a) S. J. Ji, S. Y. Wang, Y. Zhang and T. P. Loh, Tetrahedron, 2004, 60, 2051 CrossRef CAS;
(b) B. P. Bandgar and K. A. Shaikh, Tetrahedron Lett., 2003, 44, 1959 CrossRef CAS.
Footnotes |
† Electronic supplementary information (ESI) available: Detailed experimental procedures. 1H NMR and 13C NMR spectra for synthesized compounds or other electronic format. See DOI: 10.1039/d0ra05217e |
‡ The two authors contributed equally to this paper. |
|
This journal is © The Royal Society of Chemistry 2020 |