DOI:
10.1039/D3TC04365G
(Paper)
J. Mater. Chem. C, 2024,
12, 2836-2842
Stepwise post-synthetic linker installation in rare-earth metal–organic frameworks†
Received
27th November 2023
, Accepted 17th January 2024
First published on 17th January 2024
Abstract
Metal–organic frameworks based on rare earth elements (i.e. RE-MOFs) represent an important class of porous crystalline materials that have gained increasing attention due to their diverse optical, photophysical, and electronic properties. Developing strategies to enrich their properties is of great interest to expand their applications. Post-synthetic installation of secondary linkers to RE-MOFs, in principle, is an effective approach to incorporate new functionalities of RE-MOFs. However, the lack of synthesis control to generate RE-MOFs that consist of less connected clusters for linker installation limits the utility of this methodology. Herein, a co-modulator strategy was successfully used to synthesize RE-MOFs consisting of 8-connected RE6 clusters with scu topology. These new RE-MOFs allow the precise installation of different secondary linkers including photosensitizers along the a and c crystallographic axes via single-crystal-to-single-crystal transformation, and the resulting RE-MOFs exhibit either enhanced or inhibited energy transfer depending on the energy level of the inserted linker. Our work provides an effective approach for the design and synthesis of multifunctional RE-MOFs.
1. Introduction
During the evolution of reticular chemistry in the past two decades, the employment of polynuclear clusters as secondary building units (SBUs) to construct metal–organic frameworks (MOFs) marks a distinct turning point.1 Compared with the conventional single-metal nodes, SBUs allow for the directional assembly of stable crystalline porous materials, which significantly expands the applications of MOFs.2 One outstanding example is zirconium MOFs (Zr-MOFs) that are mostly based on hexanuclear [Zr6(μ3-O)4(μ3-OH)4] (Zr6) clusters. The exceptional adaptability of Zr6 clusters promotes a diverse connectivity based on the carboxylic ligands with various geometries. To date, Zr6 clusters serving as 4-, 6-, 8-, 9-, 10-, and 12-connected SBUs have been documented.3,4 In particular, the less connected clusters offer an efficient platform to modify Zr-MOFs via postsynthetic linker installation to place multiple functional groups into predetermined positions with atomic level precision.5–7
Recently, MOFs based on rare-earth (RE) elements (i.e., RE-MOFs) have received increasing attention due to their unique optical, magnetic, and electronic properties, in addition to the conventional applications in gas storage and separation.8–15 Compared with the widely studied Zr-MOFs, RE-MOFs share some topological similarities, of which the prominent one being that the fcu topology can be found in both frameworks when linking Zr6 and RE6 clusters with ditopic ligands, respectively.3,16 Nonetheless, some major difference between Zr-MOFs and RE-MOFs does exist because the hard-sphere behavior and relatively large size of the RE cations allow for multiple directionalities of coordinated carboxylic ligands.17 Typically, each RE cation can coordinate with 6 to 12 terminal ligands, while each Zr4+ can only coordinate with eight. The higher coordination number along with the moderate RE–O bond strength gives rise to a large library of unique polynuclear RE clusters that lead to the formation of sophisticated RE-MOFs with fascinating topologies.18–23 Indeed, many highly adaptable RE4, RE6, RE9, and RE18 clusters, some of which are difficult to access with other metals, have been discovered as building blocks for the assembly of RE-MOFs with diverse topologies and high connectivity.24–27 It should be noted, however, the formation and stabilization of several polynuclear clusters in RE-MOFs were recently found to be facilitated by the bridging F− ions that are generated in situ during materials synthesis from the decomposition of modulators such as 2-fluorobenzoic acid.28,29
On the other hand, although post-synthetic linker installation has proven to be an effective strategy to construct multicomponent Zr-MOFs,30–37 it is not straightforward to apply this method to increase the structural complexity and enrich the functionality of RE-MOFs. This is because that it is often challenging to synthesize RE-MOFs consisting of less connected polynuclear clusters, the required framework component to install secondary linkers. For example, the combination of Zr4+ with square-shaped tetratopic linkers usually form ftw or shp nets based on 12-connected Zr6 clusters.38–44 By using rectangular linkers with high aspect ratio, Zr-MOFs with reduced SBU connectivity, such as 8-, 6-, and 4-connected Zr6 clusters, can be constructed with scu/csq/sqc, soc, and lvt topology, respectively.3 Unfortunately, this ligand symmetry reduction strategy often appears ineffective for RE-MOFs,45–48 likely because the less connected RE clusters inevitably destabilize during MOFs growth and lead to the formation of other thermodynamically favored products with fully connected SBUs.49–51
Thus, despite some recent success involving major modification of the main ligand such as desymmetrization of tetracarboxylate linkers, synthetic approaches to construct RE-MOFs consisting less connected clusters are not widely available.52–54 Therefore, developing simple strategies to reduce the connectivity to allow for multifunctionality installation in RE-MOFs is highly desirable. The unsaturated metal sites within less connected clusters can offer the binding site to install secondary linkers that can not only increase the stability but also introduce new functions into the pristine RE-MOFs. Herein, we report the design and synthesis of RE-MOFs with 4,8-connected scu topology, NPF-320-RE (RE = Eu, Tb; NPF = Nebraska Porous Framework). This is achieved via a straightforward co-modulator method for the MOFs synthesis, and the resulting framework materials NPF-320-RE are indeed capable to incorporate different secondary linkers into distinct location. Furthermore, we show that two photosensitizer-based ditopic secondary linkers can be installed in a stepwise fashion in the framework along different crystallographic axes via single-crystal-to-single-crystal transformation. Overall, our work offers another approach to enhance and control the luminescent properties of RE-MOFs.
2. Experimental
2.1 Materials and methods
All solvents and reagents were purchased from commercial suppliers and, unless otherwise noted, used without further purification. Solution 1H and 13C nuclear magnetic resonance NMR measurements were performed on a Bruker FT-NMR spectrometer (400 MHz) or a Bruker FT-NMR spectrometer (300 MHz). Mass spectra (MS) were performed on a Waters Q-TOF mass spectrometer. Powder X-ray diffraction (PXRD) patterns were taken with either a PANalytical Empyrean diffractometer with a PIXcel 3D detector and a Rigaku Miniflex 6G XRD. The copper target X-ray tube was set to 45 kV and 40 mA.
Scanning Electron Microscope. SEM images and EDS data were collected on a tabletop Phenom ProX equipped with the Element Identification (EID) software package and a specially designed and fully integrated Energy Dispersive Spectrometer (EDS).
Photoluminescence and excitation spectra were measured on a PerkinElmer LS55 spectrometer. UV-vis spectra were measured using an Agilent Cary 300 UV-vis spectrometer. Fluorescence lifetimes were measured on an Edinburgh FLS1000 spectrometer with an EPLED-365 light source. Well suspended samples of NPF-320-RE (∼5 mg) in DMF were used photoluminescence tests.
Acid digestion was performed in 500 μL DMSO-d6 with 25 μL D2SO4 at 60 °C until the solution get clear, then the solution was used for 1H NMR measurement.
2.2 Synthetic procedures
Tetratopic ligand H4L was synthesized via the typical Suzuki couplings followed by saponification in a basic aqueous solution. Detailed synthesis procedure of H4L has been reported in the literature.55 Ditopic secondary linker 9H-carbazole-2,7-dicarboxylic acid (CzDC) was purchased and used without purification. The synthesis of ditopic secondary linker carbazole-substituted terphenyl dicarboxylic acid (CzTPDC) was described in detail in ESI† (Fig. S1).
Synthesis of NPF-320-Eu.
Eu(NO3)3·6H2O (3 mg) and 2-fluorobenzoic acid (2-FBA, 150 mg) were mixed in 1 mL DMF in a glass vial and ultrasonically dissolved. The clear solution was heated in an oven at 80 °C for 1 h. After cooling down to room temperature ligand H4L (3 mg) and 10 μL trifluoroacetic acid (TFA) were added to this solution and the mixture was sonicated for 5 min to dissolve all ligand. Then the yellow solution was put into an oven and the temperature was increased from 30 °C to 115 °C in 2 h and then kept at 115 °C for 48 h. After cooling down to room temperature in 2 h, needle-shaped single crystals were obtained. After activation, the samples were washed with fresh DMF and stay in DMF for further use.
Synthesis of NPF-320-Tb.
NPF-320-Tb was synthesized in the similar procedure as that of NPF-320-Eu, except for the use of Tb(NO3)3·6H2O (3 mg) as the metal source.
Post-synthetic installation of secondary linkers in NPF-320-RE.
NPF-320-RE (RE = Eu or Tb, ∼4 mg) was soaked in 1 mL DMF solution of secondary linker (biphenyl-4,4′-dicarboxylic acid (SL1), 2′,5′-dimethyl-[1,1′:4′,1′′-terphenyl]-4,4′′-dicarboxylic acid (SL2), CzDC, or CzTPDC, 3.3 μmol). The sample was then put in oven and the temperature was increased from 30 °C to 60 °C in 2 h and then kept at 60 °C for 12 h. After cooled down to room temperature, the solvent was exchanged with fresh DMF at least three times within 12 h.
3. Results and discussions
3.1 Synthesis and structure description of NPF-320-RE
Tetratopic ligand H4L was previously synthesized by our group and used for constructing the 4,8-c Zr-based NPF-320 with scu topology.55 This success prompted us to explore the possibility of its use to synthesize RE-MOFs with similar topology. Eddaoudi and co-workers have reported that H4L0, a structure prototype of H4L can be used to produce a RE-MOF (i.e., ftw-MOF-2) with 4,12-c ftw topology (Fig. 1a),45 for which 2-fluorobenzoic acid (2-FBA) was used as the sole modulator. It is known that the nature and composition of the modulator used in Zr-MOF synthesis is critical for producing certain topologies. We thus hypothesized that a similar strategy can be adapted for the synthesis of RE-MOFs. Indeed, a solvothermal reaction between Eu(NO3)3·6H2O and H4L in the presence of 2-FBA for 48 h yielded colorless cubic block-shaped crystals (Fig. S3, ESI†), suggesting the formation of RE-MOFs with 4,12-c ftw topology, which is consistent with Eddaoudi's observation.45 Interestingly, by introducing a co-modulator, trifluoroacetic acid (TFA), some needle-shaped crystals appeared in the product mixture (Fig. S3, ESI†). With careful adjustment of the amount of 2-FBA and TFA during the MOF synthesis, a single phase of needle-shaped crystals was obtained as NPF-320-Eu (Fig. 1b, c and Fig. S3, ESI†).
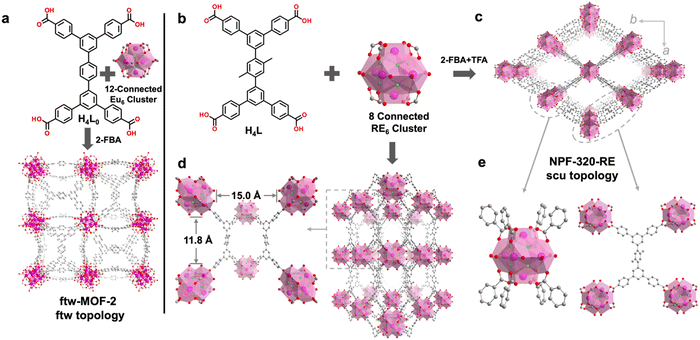 |
| Fig. 1 (a) Formation of ftw-MOF-2 from the assembly of 12-connected Eu6 cluster with ligand H4L0. (b) Primary ligand H4L and 8-connected RE6 cluster. (b) Topological representation of NPF-320-RE (C, gray; O, red; F, green; RE, pink polyhedron). (c) Simplified structure and (d) the two open pockets of NPF-320-Eu. (e) Connectivity of RE6 cluster and ligand H4L in NPF-320-RE. | |
Single-crystal X-ray diffraction (sc-XRD) study reveals that NPF-320-Eu crystalizes in the orthorhombic crystal system with the Cmmm space group (Table S1, ESI†). In the framework of NPF-320-Eu, each ligand is coordinated to four Eu6 clusters and each Eu6 cluster is coordinated to eight ligands with four above and four below the equatorial plane, and eight terminal H2O/OH− groups in the equatorial plane (Fig. S4, ESI†), with the with the overall formula of Eu6(μ3-F)8(H2O/OH−)8(L)2. It should be noted that the presence of F−-containing clusters was further confirmed by, in addition to the better crystallographic refinement compared with OH- group, SEM/EDX mapping (Fig. S5, ESI†) and the 19F NMR spectrum of an acid-digested sample (Fig. S6, ESI†). The connectivity of NPF-320-Eu belongs to a 4,8-c net, with the similar scu topology as NPF-300 and NPF-320.7,55 It should be noted that the 4,8-c scu topology is rarely reported in RE-MOFs.54,56 Due to the steric hindrance caused by the two methyl groups, the three phenyl rings on the backbone of the primary ligand are not coplanar, with the dihedral angle of 60.3 °C (Fig. S7, ESI†). NPF-320-Eu features four large 1D open channels: three rhombic channels with the size of 27 × 15 Å along the c axis, 7 × 12 Å along the b axis, and 5 × 9 Å along the a axis, and one hexagonal channel with a diameter of 12 Å along a axis (Fig. S9, ESI†). NPF-320-Tb was synthesized using the same co-modulator strategy in an isostructural form as NPF-320-Eu, which is confirmed by sc-XRD study (Table S1, ESI†). Powder X-ray diffraction (PXRD) patterns confirm the bulk phase purity of the as-synthesized NPF-320-RE when compared to the simulated patterns from the corresponding single-crystal structure (Fig. S13, ESI†).
3.2 Linkers installation in NPF-320-RE
We expect that NPF-320-RE MOFs that consist of 8-connected RE6 clusters are ideal platforms for secondary linker installation via the replacement of H2O/OH− groups on the clusters according to the size matching principle.55 In NPF-320-Eu, the pocket size along the c axis, defined as the O–O distance between the opposing H2O/OH− groups on the Eu6 clusters, is 11.8 Å, and the pocket size along the a axis is 15.0 Å (Fig. 1d). These values are similar as those found in NPF-320,55 which fit favorably with secondary linkers SL1 (11.3 Å) and SL2 (15.4 Å), respectively (Fig. 2).
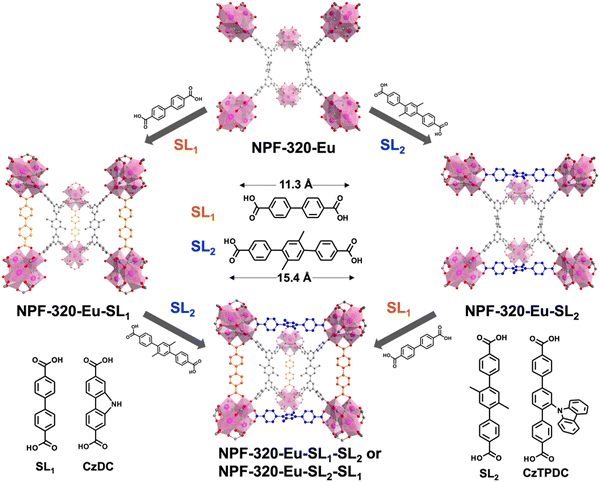 |
| Fig. 2 Stepwise post-synthetic installation of secondary linkers in NPF-320-Eu. | |
We first tested the possible installation of SL2. Briefly, as synthesized NPF-320-Eu single crystals were placed in the solution of SL2 in DMF at 60 °C for 12 h, and the resulting single crystalline material, termed NPF-320-Eu-SL2, belongs to the same Cmmm space group as NPF-320-Eu with the overall formula of Eu6(μ3-F)8(H2O/OH−)4(L)2(SL2) (Table S1, ESI†). As expected, in NPF-320-Eu-SL2, each Eu6 cluster is 10-connected to eight primary ligand L and two secondary linker SL2 along the a axis. According to the crystal structure data, the molar ratio between primary ligand L and SL2 is 2
:
1, which is further confirmed by the 1H NMR spectrum of an acid digested sample (Fig. S14, ESI†). Upon the successful installation of SL2, the O-O distance between the opposing H2O/OH− groups on the Eu6 clusters is 11.6 Å and does not change significantly. Thus, we next attempted to install SL1 into the open pockets along the c axis of NPF-320-Eu-SL2, and the resulting crystalline materials, named NPF-320-Eu-SL2–SL1, crystallized in the same Cmmm space group and the presence of SL1 was observed along the c axis (Fig. 2 and Table S1, ESI†). In NPF-320-Eu-SL2–SL1 each Eu6 cluster is now 12-connected to eight primary ligand L, two SL1, and two SL2, which gives an overall formula of Eu6(μ3-F)8(L)2(SL2)(SL1). Similarly, the molar ratio of L:SL2:SL1 of 2
:
1:1 was also confirmed by 1H NMR (Fig. S15, ESI†). The success of the linker installation on the bulk scale was further examined by PXRD patterns, which exhibit the similar peaks to the simulation (Fig. S16, ESI†).
We next attempted to install the secondary linkers into the open pockets of NPF-320-Eu via the alternate route, i.e., first SL1 followed by SL2. Indeed, single crystals of NPF-320-Eu-SL1 were obtained by heating NPF-320-Eu in a solution of SL1 in DMF (Fig. 2 and Table S1, ESI†), and the expected molar ratio of L:SL1 was supported by 1H NMR spectrum of the acid digested sample (Fig. S17, ESI†). The subsequent installation of SL2 was also successful, resulting in NPF-320-Eu-SL1–SL2 that has essentially the same structure of NPF-320-Eu-SL2–SL1, confirmed by PXRD (Fig. S16, ESI†). It should be noted that we also obtained a single crystalline material by installing 2,2′-bipyridine-5,5′-dicarboxylic acid (bpy) into NPF-320-Eu (Table S1 and Fig. S18, ESI†). The versatile metal-binding ability of ligand bpy offers a vast future opportunity enrich the photophysical, catalytic, optical properties of NPF-320-RE materials.
3.3 Photosensitizer installation in NPF-320-RE
Having confirmed the success of the installation of two secondary linkers with different length into NPF-320-Eu, we next set out to use this strategy to enrich the functionalities of the RE-MOFs. One objective is to enhance the luminescent properties of NPF-320-RE by lowering the excitation energy. Since the primary ligand H4L only absorb light in the UVB region (280–315 nm) (Fig. S19, ESI†), it is beneficial to red shift the excitation wavelength. In fact, many common MOF ligand do not strongly absorb light in the UVA (315–400 nm) or visible region (>400 nm), which limits their wider applications. Carbazole is a well-known electron donor and its derivatives have been widely used in optoelectronic and photocatalysis applications.57 Thus, we envisioned that carbazole-containing ligand such as 9H-carbazole-2,7-dicarboxylic acid (CzDC) and carbazole-substituted terphenyl dicarboxylic acid (CzTPDC) (Fig. 2) should serve as good photosensitizers. More importantly, CzDC and CzTPDC have a similar length of SL1 and SL2 to be installed into the pockets along the c axis and a axis, respectively.
Indeed, upon excitation at 365 nm, CzDC and CzTPDC exhibit strong blue emissions with the λmax at 400 nm and 460 nm, respectively (Fig. S20 and S21, ESI†), suggesting likely good sensitizing capabilities to RE cations with different efficiencies. Installation of CzDC and CzTPDC into NPF-320-Eu and NPF-320-Tb were achieved by similar method described above. The successful linker installation was confirmed by 1H NMR spectra of the corresponding acid digested NPF-320-Eu-CzDC and NPF-320-Eu-CzTPDC (Fig. S22 and S23, ESI†). PXRD patterns also indicate that the crystallinity of the materials retains after linker installation, and more importantly matches well with NPF-320-SL1 and NPF-320-SL2, respectively (Fig. S24, ESI†).
After linker installation, both NPF-320-Eu-CzDC and NPF-320-Eu-CzTPDC display three relatively intense emissions at 589, 620, and 700 nm which are originated from the 5D0 → 7FJ (J = 1, 2, and 4) transitions of Eu3+ ions (Fig. 3a–c). No apparent fluorescent emission based on the installed linker was observed, indicating a highly efficient energy transfer from the sensitizer to Eu. Moreover, to our delight, compared to the parent NPF-320-Eu, both ternary frameworks appear much brighter upon excitation at 365 nm (Fig. 3a–c, inset), with a slightly decrease of the luminescence lifetime from 1.62 ms in NPF-320-Eu to 1.52 ms and 1.47 ms in NPF-320-Eu-CzDC and NPF-320-Eu-CzTPDC, respectively (Fig. S25, ESI†).
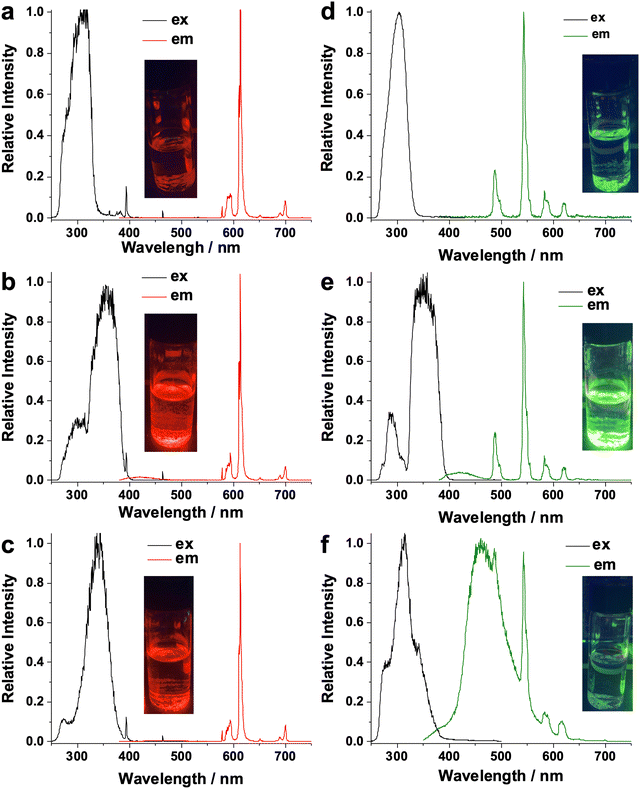 |
| Fig. 3 The excitation and emission spectra of (a) NPF-320-Eu, (b) NPF-320-Eu-CzDC, (c) NPF-320-Eu-CzTPDC, (d) NPF-320-Tb, (e) NPF-320-Tb-CzDC, (f) NPF-320-Tb-CzTPDC. Inset: The photograph under UV light (λ = 365 nm). | |
In sharp contrast, the luminescence enhancement of NPF-320-Tb was only observed upon the installation of CzDC (Fig. 3e), which displays the emission peaks at 492, 542, 587, and 621 nm assigned to the 5D4 → 7FJ (J = 6, 5, 4, and 3) transitions of Tb3+ ions. In fact, the green emission from Tb3+ in NPF-320-Tb-CzTPDC was significantly quenched after the post-synthetic installation (Fig. 3f). At the same time, a weak, broad emission band around 460 nm attributed to CzTPDC is also detected, indicating the energy transfer is inefficient. This is likely due to the lower energy of the triplet state of CzTPDC compared to CzDC. Indeed, the phosphorescence spectrum of CzTPDC indicates a triplet state energy of 20
964 cm−1 (Fig. S26a, ESI†), which is lower than that of CzDC (Fig. S26b, ESI†) and only slightly higher than the 5D4 excited state of Tb3+ (20
408 cm−1), less than the empirical optimum gap of 1850 cm−1 for the efficient energy transfer.58 In fact, the decreased Tb3+ lifetime (0.74 ms) in NPF-320-Tb-CzTPDC compared to 1.09 ms of NPF-320-Tb-CzDC also suggested a back energy transfer pathway from Tb3+ to ligand triplet state. This energy relaxation route further deteriorates the energy transfer process from the primary ligand to Tb, indicated by a weaker green luminescence in NPF-320-Tb-CzTPDC compared to NPF-320-Tb (Fig. 3d and f). Overall, our work exemplifies the power of the stepwise linker installation strategy to diversify the photoluminescent properties of RE-MOFs. A promotion or inhibition of energy transfer process with RE ions, secondary linker, as well as the primary ligand can be precisely controlled by judicious choice the photosensitizers.
4. Conclusion
In conclusion, we report a new synthesis strategy for preparing RE-MOFs consisting of less connected RE6 clusters for post-synthetic linker installation. Previous synthesis of RE-MOFs using square or rectangular tetratopic ligands usually results in the formation of 12-connected RE6 clusters with underlying ftw or shp topology which inhibit further linker installation and functionality incorporation. Herein, by using a co-modulator strategy, namely, a mixture of TFA as a second modulator combined with a commonly used 2-FBA, we have successfully used a rectangular linker to prepare RE-MOFs that consist of 8-connected RE6 clusters with underlying scu topology. More interestingly, the eight connected RE6 clusters in the scu network enable the precise installation of secondary linkers along the a and c axis via single-crystal-to-single-crystal transformation. Moreover, two photosensitizer-based secondary linkers (CzDC and CzTPDC) were successfully installed in NPF-320-RE and govern the energy transfer processes between the primary/secondary ligands with the RE ions. To the best of our knowledge, this is the first example of the installation of two distinct secondary linkers within a RE-MOF, which paves a versatile way for the future design multifunctional RE-MOFs.
Conflicts of interest
There are no conflicts to declare.
Acknowledgements
Work at the Molecular Foundry and Advanced Light Source was supported by the Office of Science, Office of Basic Energy Sciences, of the U.S. Department of Energy under Contract No. DE-AC02-05CH11231. This work is also partially supported by NSF/CBET-1706632.
References
- M. Eddaoudi, D. B. Moler, H. Li, B. Chen, T. M. Reineke, M. O'Keeffe and O. M. Yaghi, Acc. Chem. Res., 2001, 34, 319–330 CrossRef CAS PubMed.
- M. J. Kalmutzki, N. Hanikel and O. M. Yaghi, Sci. Adv., 2018, 4, eaat9180 CrossRef CAS PubMed.
- Y. Bai, Y. Dou, L. H. Xie, W. Rutledge, J. R. Li and H. C. Zhou, Chem. Soc. Rev., 2016, 45, 2327–2367 RSC.
- S. Yuan, L. Feng, K. Wang, J. Pang, M. Bosch, C. Lollar, Y. Sun, J. Qin, X. Yang, P. Zhang, Q. Wang, L. Zou, Y. Zhang, L. Zhang, Y. Fang, J. Li and H. C. Zhou, Adv. Mater., 2018, 30, 1704303 CrossRef PubMed.
- S. Yuan, W. Lu, Y. P. Chen, Q. Zhang, T. F. Liu, D. Feng, X. Wang, J. Qin and H. C. Zhou, J. Am. Chem. Soc., 2015, 137, 3177–3180 CrossRef CAS PubMed.
- C. X. Chen, Z. Wei, J. J. Jiang, Y. Z. Fan, S. P. Zheng, C. C. Cao, Y. H. Li, D. Fenske and C. Y. Su, Angew. Chem., Int. Ed., 2016, 55, 9932–9936 CrossRef CAS PubMed.
- X. Zhang, B. L. Frey, Y. S. Chen and J. Zhang, J. Am. Chem. Soc., 2018, 140, 7710–7715 CrossRef CAS PubMed.
- T. M. Reineke, M. Eddaoudi, M. O'Keeffe and O. M. Yaghi, Angew. Chem., Int. Ed., 1999, 38, 2590–2594 CrossRef CAS PubMed.
- T. M. Reineke, M. Eddaoudi, M. Fehr, D. Kelley and O. M. Yaghi, J. Am. Chem. Soc., 1999, 121, 1651–1657 CrossRef CAS.
- J. Rocha, L. D. Carlos, F. A. Paz and D. Ananias, Chem. Soc. Rev., 2011, 40, 926–940 RSC.
- Y. Cui, Y. Yue, G. Qian and B. Chen, Chem. Rev., 2012, 112, 1126–1162 CrossRef CAS PubMed.
- C. Pagis, M. Ferbinteanu, G. Rothenberg and S. Tanase, ACS Catal., 2016, 6, 6063–6072 CrossRef CAS.
- B. Yan, Acc. Chem. Res., 2017, 50, 2789–2798 CrossRef CAS PubMed.
- E. A. Dolgopolova, A. M. Rice, C. R. Martin and N. B. Shustova, Chem. Soc. Rev., 2018, 47, 4710–4728 RSC.
- S. Wu, H. Min, W. Shi and P. Cheng, Adv. Mater., 2020, 32, 1805871 CrossRef CAS PubMed.
- F. Saraci, V. Quezada-Novoa, P. R. Donnarumma and A. J. Howarth, Chem. Soc. Rev., 2020, 49, 7949–7977 RSC.
- J. C. Bunzli and C. Piguet, Chem. Rev., 2002, 102, 1897–1928 CrossRef CAS PubMed.
- D. X. Xue, A. J. Cairns, Y. Belmabkhout, L. Wojtas, Y. Liu, M. H. Alkordi and M. Eddaoudi, J. Am. Chem. Soc., 2013, 135, 7660–7667 CrossRef CAS PubMed.
- D. X. Xue, Y. Belmabkhout, O. Shekhah, H. Jiang, K. Adil, A. J. Cairns and M. Eddaoudi, J. Am. Chem. Soc., 2015, 137, 5034–5040 CrossRef CAS PubMed.
- Z. Chen, L. J. Weselinski, K. Adil, Y. Belmabkhout, A. Shkurenko, H. Jiang, P. M. Bhatt, V. Guillerm, E. Dauzon, D. X. Xue, M. O'Keeffe and M. Eddaoudi, J. Am. Chem. Soc., 2017, 139, 3265–3274 CrossRef CAS PubMed.
- T. Y. Luo, C. Liu, S. V. Eliseeva, P. F. Muldoon, S. Petoud and N. L. Rosi, J. Am. Chem. Soc., 2017, 139, 9333–9340 CrossRef CAS PubMed.
- D. Alezi, A. M. Peedikakkal, L. J. Weselinski, V. Guillerm, Y. Belmabkhout, A. J. Cairns, Z. Chen, L. Wojtas and M. Eddaoudi, J. Am. Chem. Soc., 2015, 137, 5421–5430 CrossRef CAS PubMed.
- L. Zhang, S. Yuan, L. Feng, B. Guo, J. S. Qin, B. Xu, C. Lollar, D. Sun and H. C. Zhou, Angew. Chem., Int. Ed., 2018, 57, 5095–5099 CrossRef CAS PubMed.
- L. Feng, J. Pang, P. She, J. L. Li, J. S. Qin, D. Y. Du and H. C. Zhou, Adv. Mater., 2020, 32, 2004414 CrossRef PubMed.
- Y. Wang, L. Feng, W. Fan, K. Y. Wang, X. Wang, X. Wang, K. Zhang, X. Zhang, F. Dai, D. Sun and H. C. Zhou, J. Am. Chem. Soc., 2019, 141, 6967–6975 CrossRef CAS PubMed.
- B. Guo, H. Liu, J. Pang, Q. Lyu, Y. Wang, W. Fan, X. Lu and D. Sun, J. Hazard. Mater., 2022, 436, 129094 CrossRef CAS PubMed.
- F. Li, K. Y. Wang, Z. Liu, Z. Han, D. Kuai, W. Fan, L. Feng, Y. Wang, X. Wang, Y. Li, Z. Yang, R. Wang, D. Sun and H. C. Zhou, JACS Au, 2023, 3, 1337–1347 CrossRef CAS PubMed.
- J. P. Vizuet, M. L. Mortensen, A. L. Lewis, M. A. Wunch, H. R. Firouzi, G. T. McCandless and K. J. Balkus, Jr., J. Am. Chem. Soc., 2021, 143, 17995–18000 CrossRef CAS PubMed.
- M. S. Christian, K. J. Fritzsching, J. A. Harvey, D. F. Sava Gallis, T. M. Nenoff and J. M. Rimsza, JACS Au, 2022, 2, 1889–1898 CrossRef CAS PubMed.
- S. Yuan, Y. P. Chen, J. S. Qin, W. Lu, L. Zou, Q. Zhang, X. Wang, X. Sun and H. C. Zhou, J. Am. Chem. Soc., 2016, 138, 8912–8919 CrossRef CAS PubMed.
- S. Yuan, L. Zou, H. Li, Y. P. Chen, J. Qin, Q. Zhang, W. Lu, M. B. Hall and H. C. Zhou, Angew. Chem., Int. Ed., 2016, 55, 10776–10780 CrossRef CAS PubMed.
- C. X. Chen, Z. W. Wei, J. J. Jiang, S. P. Zheng, H. P. Wang, Q. F. Qiu, C. C. Cao, D. Fenske and C. Y. Su, J. Am. Chem. Soc., 2017, 139, 6034–6037 CrossRef CAS PubMed.
- C. X. Chen, Q. F. Qiu, C. C. Cao, M. Pan, H. P. Wang, J. J. Jiang, Z. W. Wei, K. Zhu, G. Li and C. Y. Su, Chem. Commun., 2017, 53, 11403–11406 RSC.
- S. Yuan, P. Zhang, L. Zhang, A. T. Garcia-Esparza, D. Sokaras, J. S. Qin, L. Feng, G. S. Day, W. Chen, H. F. Drake, P. Elumalai, S. T. Madrahimov, D. Sun and H. C. Zhou, J. Am. Chem. Soc., 2018, 140, 10814–10819 CrossRef CAS PubMed.
- C. X. Chen, Q. F. Qiu, M. Pan, C. C. Cao, N. X. Zhu, H. P. Wang, J. J. Jiang, Z. W. Wei and C. Y. Su, Chem. Commun., 2018, 54, 13666–13669 RSC.
- J. Pang, S. Yuan, J. Qin, M. Wu, C. T. Lollar, J. Li, N. Huang, B. Li, P. Zhang and H. C. Zhou, J. Am. Chem. Soc., 2018, 140, 12328–12332 CrossRef CAS PubMed.
- C. C. Cao, C. X. Chen, Z. W. Wei, Q. F. Qiu, N. X. Zhu, Y. Y. Xiong, J. J. Jiang, D. Wang and C. Y. Su, J. Am. Chem. Soc., 2019, 141, 2589–2593 CrossRef CAS PubMed.
- W. Morris, B. Volosskiy, S. Demir, F. Gándara, P. L. McGrier, H. Furukawa, D. Cascio, J. F. Stoddart and O. M. Yaghi, Inorg. Chem., 2012, 51, 6443–6445 CrossRef CAS PubMed.
- O. V. Gutov, W. Bury, D. A. Gomez-Gualdron, V. Krungleviciute, D. Fairen-Jimenez, J. E. Mondloch, A. A. Sarjeant, S. S. Al-Juaid, R. Q. Snurr, J. T. Hupp, T. Yildirim and O. K. Farha, Chem. – Eur. J., 2014, 20, 12389–12393 CrossRef CAS PubMed.
- Z. Wei, Z. Y. Gu, R. K. Arvapally, Y. P. Chen, R. N. McDougald, Jr., J. F. Ivy, A. A. Yakovenko, D. Feng, M. A. Omary and H. C. Zhou, J. Am. Chem. Soc., 2014, 136, 8269–8276 CrossRef CAS PubMed.
- D. Feng, Z. Y. Gu, Y. P. Chen, J. Park, Z. Wei, Y. Sun, M. Bosch, S. Yuan and H. C. Zhou, J. Am. Chem. Soc., 2014, 136, 17714–17717 CrossRef CAS PubMed.
- T. C. Wang, W. Bury, D. A. Gómez-Gualdrón, N. A. Vermeulen, J. E. Mondloch, P. Deria, K. Zhang, P. Z. Moghadam, A. A. Sarjeant, R. Q. Snurr, J. F. Stoddart, J. T. Hupp and O. K. Farha, J. Am. Chem. Soc., 2015, 137, 3585–3591 CrossRef CAS PubMed.
- T. F. Liu, D. Feng, Y. P. Chen, L. Zou, M. Bosch, S. Yuan, Z. Wei, S. Fordham, K. Wang and H. C. Zhou, J. Am. Chem. Soc., 2015, 137, 413–419 CrossRef CAS PubMed.
- S. B. Kalidindi, S. Nayak, M. E. Briggs, S. Jansat, A. P. Katsoulidis, G. J. Miller, J. E. Warren, D. Antypov, F. Cora, B. Slater, M. R. Prestly, C. Marti-Gastaldo and M. J. Rosseinsky, Angew. Chem., Int. Ed., 2015, 54, 221–226 CrossRef CAS PubMed.
- R. Luebke, Y. Belmabkhout, L. J. Weselinski, A. J. Cairns, M. Alkordi, G. Norton, L. Wojtas, K. Adil and M. Eddaoudi, Chem. Sci., 2015, 6, 4095–4102 RSC.
- R. G. AbdulHalim, P. M. Bhatt, Y. Belmabkhout, A. Shkurenko, K. Adil, L. J. Barbour and M. Eddaoudi, J. Am. Chem. Soc., 2017, 139, 10715–10722 CrossRef CAS PubMed.
- H. Wang, X. Dong, V. Colombo, Q. Wang, Y. Liu, W. Liu, X. L. Wang, X. Y. Huang, D. M. Proserpio, A. Sironi, Y. Han and J. Li, Adv. Mater., 2018, 30, 1805088 CrossRef PubMed.
- D. X. Xue, A. Cadiau, L. J. Weselinski, H. Jiang, P. M. Bhatt, A. Shkurenko, L. Wojtas, Z. Chen, Y. Belmabkhout, K. Adil and M. Eddaoudi, Chem. Commun., 2018, 54, 6404–6407 RSC.
- V. Guillerm, L. J. Weselinski, Y. Belmabkhout, A. J. Cairns, V. D'Elia, L. Wojtas, K. Adil and M. Eddaoudi, Nat. Chem., 2014, 6, 673–680 CrossRef CAS PubMed.
- H. Jiang, J. Jia, A. Shkurenko, Z. Chen, K. Adil, Y. Belmabkhout, L. J. Weselinski, A. H. Assen, D. X. Xue, M. O'Keeffe and M. Eddaoudi, J. Am. Chem. Soc., 2018, 140, 8858–8867 CrossRef CAS PubMed.
- S. Roy, A. Chakraborty and T. K. Maji, Coord. Chem. Rev., 2014, 273–274, 139–164 CrossRef CAS.
- G. K. Angeli, D. Batzavali, K. Mavronasou, C. Tsangarakis, T. Stuerzer, H. Ott and P. N. Trikalitis, J. Am. Chem. Soc., 2020, 142, 15986–15994 CrossRef CAS.
- G. K. Angeli, E. Loukopoulos, K. Kouvidis, A. Bosveli, C. Tsangarakis, E. Tylianakis, G. Froudakis and P. N. Trikalitis, J. Am. Chem. Soc., 2021, 143, 10250–10260 CrossRef CAS PubMed.
- X. L. Lv, L. Feng, L. H. Xie, T. He, W. Wu, K. Y. Wang, G. Si, B. Wang, J. R. Li and H. C. Zhou, J. Am. Chem. Soc., 2021, 143, 2784–2791 CrossRef CAS PubMed.
- Y. Hu, X. Zhang, R. S. H. Khoo, C. Fiankor, X. Zhang and J. Zhang, J. Am. Chem. Soc., 2023, 145, 13929–13937 CrossRef CAS PubMed.
- H. L. Xia, K. Zhou, J. Guo, J. Zhang, X. Huang, D. Luo, X. Y. Liu and J. Li, Chem. Sci., 2022, 13, 9321–9328 RSC.
- A. van Dijken, J. J. Bastiaansen, N. M. Kiggen, B. M. Langeveld, C. Rothe, A. Monkman, I. Bach, P. Stössel and K. Brunner, J. Am. Chem. Soc., 2004, 126, 7718–7727 CrossRef CAS PubMed.
- M. Latva, H. Takalo, V.-M. Mukkala, C. Matachescu, J. C. Rodríguez-Ubis and J. Kankare, J. Lumin., 1997, 75, 149–169 CrossRef CAS.
Footnote |
† Electronic supplementary information (ESI) available: Synthesis, experimental details and procedures, NMR spectra, crystallographic information, SEM and EDX analysis, PXRD patterns, acid digestion experiments, UV-vis absorption and emission spectra, luminescence lifetime trace, phosphorescence spectra are appended. CCDC 2308004–2308010. For ESI and crystallographic data in CIF or other electronic format see DOI: https://doi.org/10.1039/d3tc04365g |
|
This journal is © The Royal Society of Chemistry 2024 |