DOI:
10.1039/D4TB00050A
(Paper)
J. Mater. Chem. B, 2024,
12, 3694-3702
Star-PCL shape memory polymer (SMP) scaffolds with tunable transition temperatures for enhanced utility†
Received
8th January 2024
, Accepted 13th March 2024
First published on 26th March 2024
Abstract
Thermoresponsive shape memory polymers (SMPs) prepared from UV-curable poly(ε-caprolactone) (PCL) macromers have the potential to create self-fitting bone scaffolds, self-expanding vaginal stents, and other shape-shifting devices. To ensure tissue safety during deployment, the shape actuation temperature (i.e., the melt transition temperature or Tm of PCL) must be reduced from ∼55 °C that is observed for scaffolds prepared from linear-PCL-DA (Mn ∼ 10 kg mol−1). Moreover, increasing the rate of biodegradation would be advantageous, facilitating bone tissue healing and potentially eliminating the need for stent retrieval. Herein, a series of six UV-curable PCL macromers were prepared with linear or 4-arm star architectures and with Mns of 10, 7.5, and 5 kg mol−1, and subsequently fabricated into six porous scaffold compositions (10k
, 7.5k
, 5k
, 10k★, 7.5k★, and 5k★) via solvent casting particulate leaching (SCPL). Scaffolds produced from star-PCL-tetraacrylate (star-PCL-TA) macromers produced pronounced reductions in Tm with decreased Mnversus those formed with the corresponding linear-PCL-diacrylate (linear-PCL-DA) macromers. Scaffolds were produced with the desired reduced Tm profiles: 37 °C < Tm < 55 °C (self-fitting bone scaffold), and Tm ≤ 37 °C (self-expanding stent). As macromer Mn decreased, crosslink density increased while % crystallinity decreased, particularly for scaffolds prepared from star-PCL-TA macromers. While shape memory behavior was retained and radial expansion pressure increased, this imparted a reduction in modulus but with an increase in the rate of degradation.
Introduction
Thermoresponsive shape memory polymers (SMPs) have been widely explored for biomedical applications.1,2 Biodegradable, semi-crystalline poly(ε-caprolactone) (PCL) SMPs3,4 have been proposed for numerous biomedical applications including ‘self-closing sutures’, and ‘self-expanding’ cardiovascular stents.5,6 We developed ‘self-fitting’ PCL-based scaffolds for bone regeneration.7 UV-curable PCL macromers afford convenient fabrication of such devices. For instance, we utilized linear-PCL-diacrylate (linear-PCL-DA, Mn ∼ 10 kg mol−1) to prepare porous scaffolds via the UV cure of a solvent-based solution over a fused salt template followed by aqueous extraction of the template (i.e., solvent-casting/particulate leaching, SCPL).7,8 In the resulting PCL networks, the covalent crosslinks act as net points while the crystalline lamellae act as switching segments. These scaffolds exhibited a melt transition (Tm) of ∼55 °C, such that when heated (T > Tm), the crystalline lamellae melt causing the scaffold to become soft and malleable. This allows the scaffold to press-fit into irregular defects, with shape recovery driving expansion to the perimeter. Shape fixity occurs when the scaffold is subsequently cooled to body temperature (∼37 °C) (T < Tm). These conformal, self-fitting PCL SMP scaffolds have demonstrated excellent osseointegration and healing in a preclinical study,9 and so represent a potential alternative to existing biological and alloplastic grafts.
The Tm of PCL SMPs is vital as it serves as the actuation temperature for shape recovery during implantation. Due to the risk of thermal damage to tissues,10–13 systematically reducing the Tm of PCL SMP scaffolds is necessary. In the case of bone scaffolds prepared from linear-PCL-DA (Mn ∼ 10 kg mol−1), a Tm of 55 °C prohibits surgical site irrigation to extend the working time. Ideally, such a scaffold would exhibit a 37 °C < Tm < 55 °C so that, once fitted, would return to the rigid state to support load. We also recently proposed porous, self-expanding vaginal stents that could be inserted in a fixed, compressed state, and then shape recovered in situ to provide patency.14,15 Such a device would require a Tm ≤ 37 °C. Other devices would also ideally shape recovery at body temperature (e.g., vascular stents, surgical sutures, etc.). Overall, systematic reductions in the Tm values of PCL SMPs would represent a significant enhancement in tissue safety and hence utility.
The % crystallinity and Tm values of polymers is known to be influenced by architecture as well as molecular weight (Mn).16,17Versus analogous linear polymers of the same Mn, the lower steric mobility and shorter chain length of multi-arm polymers reduce crystallization.18–20 This effect has been observed for specifically star homopolymers (i.e., 4-arm extending from a central core).19–24 We have reported that star-PCL-tetracrylate (star-PCL-TA, Mn ∼ 10 kg mol−1) exhibited a significantly lower Tm (∼45 °C) versus its linear analogue (linear-PCL-DA, Mn ∼ 10 kg mol−1; ∼55 °C).25 Because of the concomitant deduction in % crystallinity, star- polyesters have been utilized to tailor rates of degradation.26–28 Indeed, a faster rate of degradation was noted for scaffolds prepared from star-PCL-TA (Mn ∼ 10 kg mol−1) versus linear-PCL-DA (Mn ∼ 10 kg mol−1). Lastly, star- polymers are well known for having relatively reduced dilute solution viscosities, due to their reduced hydrodynamic volumes and fewer chain entanglements,19,20,29,30 which can be helpful in solvent-based fabrication processes.
Herein, towards tuning the Tm of PCL-based SMP scaffolds to afford greater utility and tissue safety, the architecture and Mn of UV curable macromers were systematically tuned (Fig. 1). PCL macromers were prepared with linear- and star- architectures, and Mn was modulated in increments of 2500 g mol−1. From this, the following six scaffold compositions were prepared from the designated macromer: 10k
(linear-PCL-DA, Mn ∼ 10 kg mol−1), 7.5k
(linear-PCL-DA, Mn ∼ 7.5 kg mol−1), 5k
(linear-PCL-DA, Mn ∼ 5 kg mol−1), 10k★ (star-PCL-TA, Mn ∼ 10 kg mol−1), 7.5k★ (star-PCL-TA, Mn ∼ 7.5 kg mol−1), and 5k★ (star-PCL-TA, Mn ∼ 5 kg mol−1). All porous scaffolds were prepared via SCPL to generate similar pore sizes as well as pore interconnectivity. These six SMP scaffold compositions were then assessed in terms of rheological, thermal, shape memory, degradative, and mechanical properties towards advancing their use as tissue-safe self-fitting bone scaffolds, self-expanding vaginal stents, as well as potentially other SMP devices.
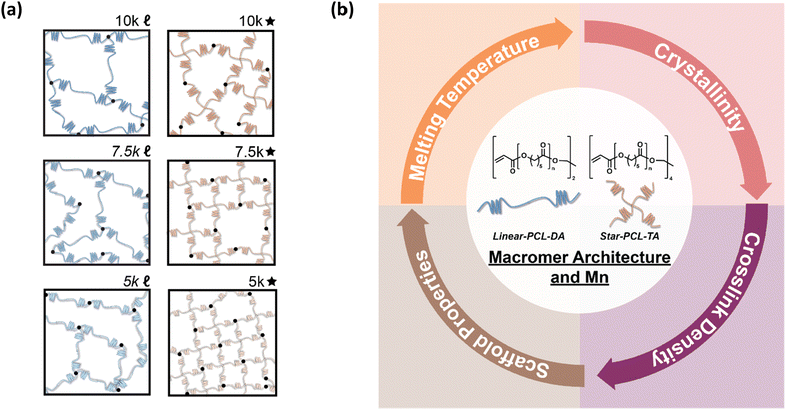 |
| Fig. 1 To achieve porous PCL SMP scaffolds with systematically lower, “tissue safe” shape transition temperatures (Tm), (a) linear-PCL-DA and star-PCL-TA UV-curable macromers of varying Mns (10, 7.5, and 5 kg mol−1) were synthesized and used to prepare a series of 6 scaffolds: 10k , 7.5k , 5k , 10k★, 7.5k★, and 5k★. (b) Alternations to macromer architecture and Mn produced changes in Tm, % crystallinity, and crosslink density that in turn produced changes in scaffold properties (e.g., mechanical and degradation). | |
Experimental
Materials
Linear-PCL-diol (Mn = 10 kg mol−1 per manufacturer), ε-caprolactone, pentaerythritol, tin(II) 2-ethylhexanoate (Sn(Oct)2), ethylene glycol, dichloromethane (DCM), 4-(dimethylamino)pyridine (DMAP), triethylamine (Et3N), acryloyl chloride, ethyl acetate, potassium carbonate (K2CO3), anhydrous magnesium sulfate (MgSO4), sodium chloride (NaCl) salt, 2,2-dimethoxy-2-phenyl acetophenone (DMP), 1-vinyl-2-pyrrolidinone (NVP), sodium hydroxide (NaOH), deuterated chloroform (CDCl3), phosphate-buffered saline (PBS), and solvents were obtained from Sigma-Aldrich. All solvents were dried over 4 Å molecular sieves, all reagents were vacuum dried overnight (ON), and all glassware and stir bars were dried at 120 °C ON prior to use. Salt was sieved to an average size of 460 ± 70 μm (per scanning electron microscopy [SEM] and ImageJ) using an ASTM E-11 no. 40 and no. 35 sieves with 425 μm and 500 μm openings, respectively.
Methods
Syntheses.
All reactions were run under a nitrogen (N2) atmosphere with a Teflon-covered stir bar. After purification, molecular structures (including % acrylation, architecture, and Mn) were determined with 1H NMR spectroscopy (Avance Neo 400 MHz spectrometer) operating in the FT-mode with CDCl3 as the standard (Fig. S1 and S2, ESI†). The target Mn and architecture of linear-PCL-DA and star-PCL-TA macromers were verified by comparing the repeat unit CH2δ = 4.1 ppm versus the terminal CH2δ = 3.7 ppm. Differential scanning calorimetry (DSC, TA Instruments Q100) protocols described below were used to determine thermal properties.
Linear-PCL-diol (10 kg mol−1) was purchased while linear-PCL-diols (7.5 and 5 kg mol−1) were synthesized via ring-opening polymerization (ROP) as follows. ε-Caprolactone (20.0 g), a difunctional initiator (ethylene glycol), and Sn(Oct)2 were combined in a round bottom (rb) flask and maintained at 120 °C ON. The targeted Mns were achieved by adjusting the monomer to initiator molar ratio: 88
:
1 [10 kg mol−1], 66
:
1 [7.5 kg mol−1], and 44
:
1 [5 kg mol−1]. The crude product was dissolved in DCM, precipitated into methanol, vacuum filtered, and dried (room temperature [RT], overnight [ON], 30 in. Hg) to obtain the purified product. Star-PCL-tetrols (10, 7.5, and 5 kg mol−1) were likewise prepared via the Sn(Oct)2-catalized ROP of ε-caprolactone (20.0 g), but in the presence of a tetrafunctional initiator (pentaerythritol), and at the designated monomer to initiator molar ratios: 88
:
1 [10 kg mol−1], 66
:
1 [7.5 kg mol−1], and 44
:
1 [5 kg mol−1]. The final products were likewise isolated.
Linear-PCL-diols and star-PCL-tetrols were acrylated to form UV-curable linear-PCL-DA and star-PCL-TA macromers, respectively, using established acrylation protocols.8 Briefly, each diol (20.0 g) was combined with DMAP (6.6 mg for linear-PCL-diols; 13.2 mg for star-PCL-tetrols) and dissolved in DCM (120 mL). Et3N and acryloyl chloride (4.0 mmol & 8.0 mmol for linear-PCL-diols; 8.0 mmol & 16.0 mmol for star-PCL-tetrols) were each added dropwise to the flask and stirred under positive N2 pressure for 30 min at RT. Established work-up procedures8 were followed to obtain linear-PCL-DA and star-PCL-TA. The final products were isolated as above.
Fabrication
Scaffolds.
Porous scaffolds (10k
, 7.5k
, 5k
, 10k★, 7.5k★, and 5k★) were fabricated using a SCPL protocol per previous reports.7,8,25 Briefly, sieved NaCl (10 g; 460 ± 70 μm) was placed into a 20 mL scintillation vial (I.D. = 25 mm). DI water (7.5 wt%) was added in four parts followed by manual stirring after each addition. The wet salt was then compacted using a glass stir rod and the vials centrifuged (15 min, 3220 rpm). The open vials were then air-dried (∼1 h) and vacuum dried (RT, ON, 30 in. Hg).
Macromer solutions were prepared by dissolving the designated acrylated macromer in DCM (0.15 g total per mL DCM). Next, photoinitiator solution (10 wt% DMP in NVP) was added at 15 vol%. To each salt template, 5 mL of the resulting solution were added to a vial containing the fused salt template and subsequently centrifuged (10 min, 1260 rpm). Crosslinking of the acrylated macromers was afforded by exposing open vials to UV light for 6 min (UV-Transilluminator, 6 mW cm2, 365 nm). Opened vials were then left in the fume hood to air dry ON. Next, to remove the salt template, vials were placed in a 1
:
1 ratio of water to ethanol for ∼5 days with daily solution changes. Scaffolds were dried in the fume hood ON, and then heat-treated (85 °C, 1 h, 30 in. Hg). The resulting cylindrical scaffolds (d ∼ 12 mm) were sequentially sliced into three specimens (t ∼ 2 mm) (Vibratome, Leica VT 1000 S) and biopsy punched (Integra Miltex, d ∼ 6 mm). The final dimension of a scaffold disc specimen was d ∼ 6 mm × t ∼ 2 mm.
Films.
Analogous solid films (10k
, 7.5k
, 5k
, 10k★, 7.5k★, and 5k★) were prepared for crosslink density and % porosity calculations. Macromer solutions were prepared (0.43 g total per mL DCM), and a designated solution added to a circular silicone mold (d ∼ 45 mm × t ∼ 2 mm; McMaster-Carr) secured between two glass slides. The mold was then exposed to UV-light (UV Transilluminator, 6 mW cm2, 365 nm) for 3 min on each side. The swollen films were sequentially air-dried (RT, ON), dried in vacuo (RT, 4 h, 30 in. Hg), soaked in ethanol atop a shaker table (150 rpm, 4 h), air dried (RT, ON), and heat-treated in vacuo (85 °C, 1 h, 30 in. Hg). Films were biopsy punched to form final disc specimens (d ∼ 4 mm × t ∼ 1.5 mm).
Crosslink density
Crosslink density was analyzed via a swelling test on solid film discs (N = 3). Each disc was submerged in 10 mL of DCM for 24 h in a sealed vial. Solvent-swollen discs were removed, diameters immediately determined with an electronic caliper, and specimens photographed. Solvent-swollen discs were subsequently dried in vacuo (RT, ON, 30 in. Hg.), diameters likewise recorded, and photographed.
Macromer solution viscosity and diffusion through salt template
The reduced viscosities (ηred) of macromer (i.e., linear-PCL-DA and star-PCL-TA) solutions were determined using a Cannon-Fenske viscometer (size 200). Macromer solutions were created at incremental concentrations (0.075, 0.150, 0.225, and 0.300 g mL−1 in DCM), and tested in triplicate per concentration (N = 3). Solutions (10 mL) were poured into the viscometer and maintained at 25 °C using a surrounding water bath. A stopwatch was used to measure the efflux time of each solution through the designated region. The ηred of each solution may be expressed and calculated per the following equation: | 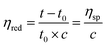 | (1) |
where ηsp is the specific viscosity, c is the concentration of the macromer solution, t is the efflux time, and t0 is the efflux time of the solvent.
Dye-containing macromer solutions were used to analyze the relative differences in diffusion rates through a salt template during a SCPL fabrication process. For improved visual observation, taller salt molds were prepared as above but with increased amount of sieved NaCl (∼15 g). A macromer solution (∼7.5 mL) containing the photoinitiator was prepared as above, to which a few drops of blue food coloring was added. Two salt templates were placed side-by-side while the linear- and star- macromer solutions of the same Mn were simultaneously poured over the template, and diffusion recorded by video.
Sol content
Scaffold disc specimens (N = 3) were each submerged into 10 mL of DCM in a scintillation vial. Vials were sealed and placed atop a shaker plate (48 h, 150 rpm). Next, scaffolds were removed, rinsed with DCM, air dried (∼2 h), and dried in vacuo (RT, ON, 30 in. Hg). Initial and final masses were used to calculate the % sol (i.e., % mass loss).
Morphology
Pore size and interconnectivity.
SEM (Tescan Vega 3, Au–Pt sputter coating (∼14 nm), accelerating voltage ∼10 kV) was used to analyze pore size and pore interconnectivity. Scaffold images (N = 3) were analyzed using ImageJ, with measurements (N = 12) taken from pores along the diagonal midline to determine the average pore size.
% Porosity.
Scaffold % porosity was gravimetrically determined (N = 3) from the densities of solid films (ρsolid
film) and corresponding porous scaffolds (ρporous
scaffold) per eqn (2): |  | (2) |
% Pore interconnectivity.
Scaffold specimens (N = 3) were evaluated to determine their pore interconnectivity using a water wicking protocol.31 Specimens were individually submerged in 10 mL DI water in a sealed vial, and placed on a shaker plate (24 h, 150 rpm) to expel any air bubbles within pores. Specimens were then removed, and weighed on a Petri dish (Masstotal). To wick away the water within the interconnected pore volume, a folded Kimwipe was gently pressed onto the surface of the specimen for 1 min, and the specimen was weighed (Massinterconnected). The % pore interconnectivity was calculated viaeqn (3): |  | (3) |
T
m and % crystallinity
DSC (TA Instruments Q100) was used to characterize Tm and % crystallinity of diols and tetrols, acrylated macromers, and scaffolds. Each specimen (∼10 mg; N = 3) was sealed in a hermetic pan, and heated/cooled (5 °C min−1) over two cycles. The endothermic melt peak was characterized in terms of the onset (Tm,onset) and midpoint (Tm) values (TA Universal Analysis Software). Reported values were determined using the second cycle to account for removal of thermal history. Eqn (4) was used to quantify PCL % crystallinity: | 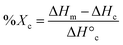 | (4) |
where ΔHm is the enthalpy of fusion calculated from the integral of the endothermic melt peak, ΔHc is the enthalpy of crystallization from the exothermic cold crystallization peak, and ΔH°c is the theoretical value for 100% crystalline PCL (139.5 J g−1).32
Shape memory properties
Shape fixity and recovery.
Shape memory properties of scaffold specimens (N = 3) were evaluated using a model defect as previously described.25 A circular defect (d ∼ 5 mm) was created from an UHMWPE sheet (t ∼ 2 mm) using a drill press (Grizzly G7948). A hot plate equipped with a digital temperature probe (Heidolph, MR HEI-TEC) was used to pre-heat saline to the designated Tm. Each scaffold specimen was subjected to the following protocol, and the diameter recorded as noted with an electronic calipers to quantify scaffold strain (ε): (1) record diameter; (2) submerge into the water bath at the designated Tm (∼1 min); (3) remove from the water bath and immediately press fit into the model defect (at RT); (4) maintain in the defect for ∼2 min to allow for shape fixity; (5) remove from the defect, and allowed to sit for ∼2 min (at RT); (6) record diameter; (7) re-submerge into the water bath at designated Tm (∼1 min) to allow for shape recovery; (8) remove from the water bath and cool for ∼2 min (at RT); (9) record diameter. This procedure was repeated twice to determine shape fixity (Rf) and shape recovery (Rr) over for the first (N = 1) and second (N = 2) cycles according to their respective equations. | 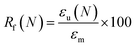 | (5) |
| 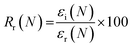 | (6) |
where εu(N) is the strain in the stress-free state, εm is the maximum strain, εi(N) is the initial strain is a stress-free environment, and εr(N) is the recovered strain in a stress-free environment.
Radial pressure during shape recovery.
Scaffold discs (N = 5) were subjected to radial mechanical testing (Instron 5965 equipped with a clockwise RJA62 J-crimp Radial Compression Station) per a prior reported.25 This protocol was utilized to determine the radial pressure exerted by a scaffold during shape recovery at its designated Tm to mimic press-fitting into a defect (d ∼ 4.5 mm). Each specimen was loaded into the bore (d ∼ 6.5 mm), heated to the Tm, and maintained for 5 min. The bore then compressed to d ∼ 4.5 mm at a rate of 1 mm min−1. At this point, force was recorded, total radial force (TRF) calculated, and TRF converted to radial pressure based on scaffold dimensions.33
Degradation
Degradation studies under accelerated conditions were performed according to ASTM F1635. Scaffold specimens (N = 3 per time point) were each submerged in 10 mL of 0.1 M NaOH in a 20 mL glass scintillation vials. The sealed vials were placed in an incubator (VWR Benchtop Shaking Incubator, Model 1570), and maintained at 37 °C and 60 rpm. At the designated time points (1, 2, 3, 4, 5, 6, 7, 8, 9, 10, 12, and 15 days), specimens were removed, rinsed well with DI water, and dried in vacuo (RT; ON; 36 in. Hg). The final dried mass was compared to the initial mass to determine the mass loss. SEM images of specimens at 7 days were obtained as described above.
Compressive mechanical properties
Scaffold specimens (N = 5) underwent static compression testing (Instron 5944) at RT with a constant rate of strain (1.5 mm min−1) up to 85% strain (ε). Due to their non-brittle nature, no specimen fractured. The compressive modulus (E), strength (CS), and toughness were determined. From the resulting stress (σ) versus ε curve, and E was calculated from the initial linear region (≤10% ε). CS was determined at 85% ε. Toughness values were calculated from the areas under the σ vs. ε curves.
Statistical analysis
All data was reported as the average ± the standard deviation. Statistical analysis was performed using one-way analysis of variance (ANOVA) between compositions of similar architecture followed by Welches t-test to make direct comparisons between
vs. ★ compositions at the same Mn. 10k
and 10k★ were used as the respective controls during the ANOVA tests. A p-value of * < 0.05 was considered statistically significant.
Results and discussion
Macromer synthesis
Linear-PCL-diols, star-PCL-tetrols, linear-PCL-DAs, and star-PCL-TAs were characterized via1H NMR to confirm molecular structure and Mns as well as by DSC to evaluate thermal properties (Fig. S1 and S2, ESI†). The targeted Mns (10, 7.5, and 5 kg mol−1) were achieved by modulating the ratio of monomer to di- or tetrafunctional initiator. For a given Mn, end group analysis verified linear versus star architecture due to the doubling of end group protons for the latter. Spectra also confirmed that diols and tetrols were successfully acrylated (>85%). For linear-PCL-diols and linear-PCL-DAs, Tm [∼53–54 °C] and % crystallinity [∼46–53%] were not substantially impacted by Mn. This was also the case for the Tm [∼44–49 °C] and % crystallinity [∼42–50%] values of star-PCL-diols and star-PCL-TAs.
Crosslink density
Prior to fabrication of porous scaffolds, solid films were produced by acrylated macromers to determine relative crosslink density. Diminished swelling (i.e., decreased % diameter change) was expected as crosslink density increased. As macromer Mn decreased, crosslink density would increase. Also, for a given Mn, crosslink density would increase for star- versus linear- macromers. These expected trends were observed (Fig. S3 and Table S1, ESI†). Notably, for a given Mn, the films based on star- macromers exhibited significantly less swelling. In this way, differences in crosslink density produced by macromers can be used to subsequently explain properties of crosslinked scaffolds.
Solution viscosity and diffusion
Polymers with a star architecture are known to have a lower hydrodynamic volume and hence lower solution viscosity versus a linear analogue of the same Mn.19,20,29,30 Thus, the ηred values of acrylated macromer solutions were determined to further verify architecture (Fig. S4 and Table S2, ESI†). This expected trends were generally observed, with star-PCL-TAs (10 and 7.5 kg mol−1) having lower ηredversus the corresponding linear-PCL-DAs. The reduced viscosities of star-PCL-TA (5 kg mol−1) and linear-PCL-TA (5 kg mol−1) were quite similar, perhaps due to their low Mn and hence short arm lengths. As expected, ηred values decreased as macromer Mn decreased.
A lower ηred was expected to facilitate scaffold fabrication by enhancing the rate of diffusion of the macromer solution through the fused salt template during SCPL. To observe this, macromer solutions containing a dye were cast over templates to assess relative diffusion rates (Fig. S5, ESI†). As anticipated, macromer solutions based on star-PCL-TAs exhibited faster diffusion versus those based on linear-PCL-TAs of the same Mn with rates increasing as Mn decreased. While the scaffold fabrication protocol used herein utilized centrifugation to ensure that macromer solutions diffused completed through a salt template, the ηred exhibited by star macromers could be advantageous for preparing larger scaffolds that cannot be subjected to centrifugation.
Scaffold fabrication and porosity
Scaffolds were fabricated via SCPL from linear-PCL-DA and star-PCL-TA macromers to produce six compositions based on architecture and Mn: 10k
, 7.5k
, 5k
, 10k★, 7.5k★, and 5k★. Sol content studies confirmed successful crosslinking of macromers, with all compositions displaying low sol content (< ∼5%) (Fig. S6 and Table S3, ESI†). Analysis of SEM images (Fig. S7a, ESI†) revealed that scaffold average pore sizes (∼190 μm) were largely similar (Fig. S7b and Table S4, ESI†). This pore size range is within that associated with osseointegration and subsequent vascularization between the host bone and scaffold,34 but could be modulated via salt size if preferred for other applications. Scaffolds exhibited ∼64–77% porosity (Fig. S7c and Table S4, ESI†). The use of the fused salt template during SCPL fabrication resulted in scaffolds with similar average pore interconnectivity (∼51%) (Fig. S8 and Table S4, ESI†). In the case of bone regeneration, this feature is anticipated to enhance cellular infiltration and neotissue formation throughout the scaffold. For vaginal stents, pore interconnectivity should facilitate egress.
Scaffold Tm and % crystallinity
T
m is a vital aspect of shape memory PCL scaffolds as it represents the temperature required for self-fitting (e.g., within bone defects) and self-expansion (e.g., as a stent within the vaginal canal). As noted earlier, a self-fitting scaffold to treat bone detects would ideally exhibit 37 °C < Tm < 55 °C, thus permitting continued irrigation at the defect site without necrosis (to prolong the fitting time), but subsequently returning to a rigid state to support load. In the case of a self-expanding vaginal stent, a Tm < 37 °C would permit insertion in a fixed, compressed state followed by expansion. A reduction in PCL % crystallinity is expected to give rise to lower Tm values. The Tm and % crystallinity of scaffolds were obtained from DSC thermograms (Fig. 2 and Table S5, ESI†). A reduction in Mn of linear-PCL-DA did not lead to a significant decrease in scaffold Tm (∼51–56 °C) despite the decrease in % crystallinity: 10k
(∼46%) versus 7.5k
(∼37%) and 5k
(∼35%). Thus, no linear- scaffold exhibited the desirable thermal profile. In contrast, scaffolds prepared from star-PCL-TAs of decreasing Mn exhibited a pronounced and systematic decrease in Tm and % crystallinity: 10k★ (∼46 °C/∼27%), 7.5k★ (∼40 °C/∼22%), and 5k★(∼29 °C/∼19%). Furthermore, for a given Mn, these values were lower for star- scaffolds versus linear- scaffolds. This reduction is attributed to the shorter length of star “arms” (i.e., higher crosslink density) that limits the potential to form crystalline lamellae, a phenomena observed in other semi-crystalline polymers.19–24 Based on achieved Tm values, specific compositions emerged as candidates for the aforementioned tissue-safe applications: 10k★ and 7.5k★ (self-expanding bone tissue scaffold), and 5k★ (self-expanding stent). Still, the differences in scaffold % crystallinity and crosslink density was expected to impact other key properties as detailed below.
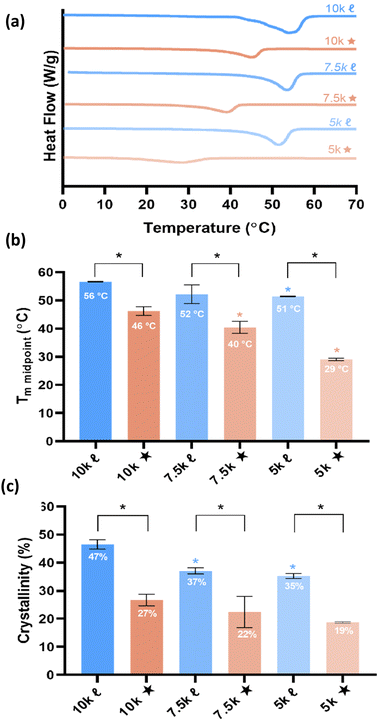 |
| Fig. 2 (a) Representative DSC thermograph of scaffolds. (b) Tm values. (c) % Crystallinity values. “Black *” p < 0.05 comparing linear- and star- scaffolds of same Mn. “Blue *” p < 0.05 versus 10k . “Pink *” p < 0.05 versus 10k★. | |
Shape memory behavior and radial expansion pressure
Given the role of crystalline lamellae as switching segments in shape memory behavior, it is paramount that scaffolds retain sufficient % crystallinity. Shape memory tests were performed using a circular model defect (d ∼ 5 mm). Slightly oversized scaffold specimens (d ∼ 6 mm) were used to ensure contact with the defect perimeter. The model defect diameter also coincides with the dimensions of a rat calvarial defect model often used in preliminary assessment of bone regeneration.35,36 Thus, the protocol mimics how scaffolds would undergo self-fitting in such defects, with the scaffold's Tm (midpoint) used as the temperature of the saline bath. As detailed in the methods, shape fixity (Rf) was related to retention of new diameter following fitting into and removal from defect while shape recovery (Rr) was related to subsequent recovery of original diameter. Rf and Rr were measured over 2 cycles. While the % crystallinity of linear- scaffolds (∼35–47%) was greater than that of star-scaffolds (∼19–27%), shape memory behavior was retained with Rf and Rr values near ∼100% for all compositions (Table S6, ESI†). Based on visual observations, the 5k★ had slightly diminished Rf values, attributed to its low % crystallinity.
The radial pressure exerted by such a scaffold specimen (d ∼ 6 mm) during shape recovery was evaluated upon placing into a bore, equilibrating at its Tm (midpoint), and reducing the diameter (d ∼ 4.5 mm) (Fig. 3a). It was expected that as crosslink density increased (i.e., macromer Mn decreased), radial pressure would increase.37 However, linear- scaffolds exhibited statistically similar radial pressures (∼100 kPa) (Fig. 3b and Table S7, ESI†). At a given Mn, star- scaffolds did exhibit greater radial pressures (∼156–273 kPa) versus linear- scaffolds, which may be attributed to more rapid melting of lamellae. Radial pressures of 7.5k★ and 5k★ were lower versus that of 10k★ due to the reduction in % crystallinity.
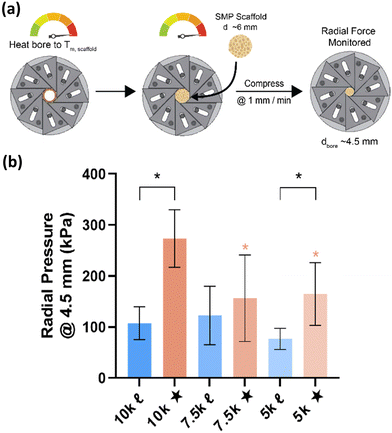 |
| Fig. 3 (a) Protocol to measure, and (b) radial pressure exerted by scaffold specimens at designated Tm. “Black *” p < 0.05 comparing linear- and star- scaffolds of same Mn. “Blue *” p < 0.05 versus 10k . “Pink *” p < 0.05 versus 10k★. | |
Degradation behavior
The slow degradation of PCL in vivo (∼2 years)38 is generally limiting to resorbable device development, such that faster as well as tunable rates of degradation would enhance its utility. In bone tissue regeneration, a scaffold should not impede neotissue formation (∼8–12 weeks),39,40 whereas gynecological stents that are resorbed (∼1–3 months) may eliminate post-operative removal.14,41 Degradation rates of scaffolds were evaluated under base-catalyzed conditions via gravimetric (Fig. 4a–d, and Table S8, ESI†), visual inspection (Fig. 4e and f), and SEM imaging (Fig. S9, ESI†) assessments. As expected, % crystallinity and crosslink density were linked to the observed relative degradation rates. For a given Mn, star- scaffolds degraded faster versus linear- scaffolds due to the reduction in % crystallinity, despite a higher crosslink density. This was particularly true of 7.5k★ and 5k★. As Mn was decreased from 10 to 7.5 kg mol−1, 7.5k
degraded somewhat faster versus 10k
, likely stemming from the former's lower crystallinity. However, the 5k
exhibited the slowest relative degradation rate due its relatively high crosslink density as well as significant % crystallinity. The fastest rate of degradation was observed for 5k★ which, while having the greatest crosslink density, had the lowest % crystallinity.
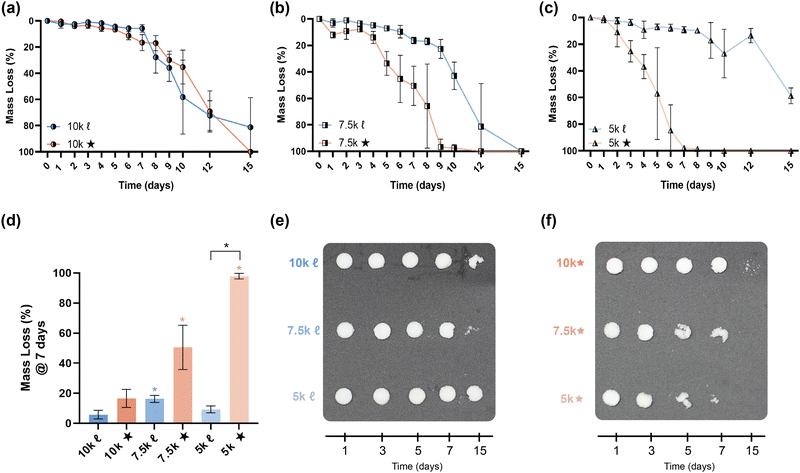 |
| Fig. 4 Base-catalyzed (0.1 M NaOH, 37 °C) degradation studies of scaffolds prepared from (a) 10 kg mol−1, (b) 7.5 kg mol−1, and (c) 5 kg mol−1 macromers. (d) Mass loss at 7 days. (e) and (f) Representative photos of specimens at different timepoints. “Black *” p < 0.05 comparing linear- and star- scaffolds of same Mn. “Blue *” p < 0.05 versus 10k . “Pink *” p < 0.05 versus 10k★. | |
Mechanical properties
The mechanical properties of SMP scaffolds are important to the utility in various shape-actuating device applications. For instance, modulus reflects the stiffness or resistance to deformation. Thus, static compressive testing was performed to determine compressive modulus (E), compressive strength (CS), and toughness (Fig. 5). Non-brittleness was confirmed for all scaffolds due to a lack of fracture at maximum strain (ε = 85%). As with the aforementioned properties, % crystallinity and crosslink density produced differences among scaffolds. Linear- scaffolds provided the highest (and statistically similar) E values (∼4.7–7 MPa), attributed to their highest % crystallinity (∼35–47%). For a given Mn, star- scaffolds exhibited significantly lower E values versus linear- scaffolds: 10k★ (∼2.0 MPa; 26%), 7.5k★ (1.3 MPa; 22%), and 5k★ (∼0.69 MPa; ∼18%). For the star- scaffolds, this general decrease in E with decreasing Mn is also attributed to a reduction in % crystallinity, despite the higher crosslink density. Similar trends were also observed for other mechanical properties. The CS and toughness of linear- scaffolds (∼28–33 MPa; ∼4.3–5.2 MJ m−3) was substantially greater than that of star- scaffolds (∼5.4–10.6 MPa; ∼0.86–1.8 MJ m−3). While star- scaffolds were not as robust, their properties still exceed that of hydrogels that are often explored for implanted medical devices (e.g., poly(ethylene glycol)-DA [3.4 kg mol−1]: E ∼0.2 MPa, CS = ∼0.13 MPa, and toughness = ∼0.015 MJ m−3).42
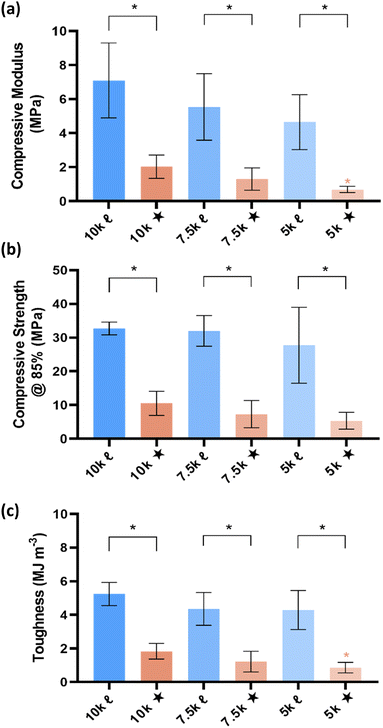 |
| Fig. 5 Compressive (a) modulus, (b) strength (at ε = 85%), and (c) toughness of scaffolds. “Black *” p < 0.05 comparing linear- and star- scaffolds of same Mn. “Blue *” p < 0.05 versus 10k . “Pink *” p < 0.05 versus 10k★. | |
Conclusions
Shape memory implanted devices, readily created from acrylated PCL macromers, hold potential for broad utility stemming from the ability to undergo thermally-triggered shape actuation. For instance, leveraging shape recovery to undergo expansion within spaces, porous PCL SMP scaffolds could be used as self-fitting bone scaffolds or as self-expanding vaginal stents. Utility in such applications necessitates that thermal triggering be accomplished at temperatures safe to biological tissues or at body temperature. The Tm of PCL serves as its shape actuation temperature. Thus, a self-fitting scaffold would ideally exhibit 37 °C < Tm < 55 °C to allow surgical-site irrigation to prolong the fitting time before returning to a rigid state. A self-expanding vaginal stent should exhibit a Tm < 37 °C to enable insertion in a fixed, compressed state followed by in situ expansion. In this study, the targeted systematic decreases in PCL scaffold Tm was achieved via macromers with a star architecture and reductions in Mn. Lower Tm values were expected upon adequately reducing % crystallinity. This was demonstrated with a series of six scaffolds prepared from the designated linear-PCL-DA or star-PCL-TA macromers of varying Mn: 10k
(Mn ∼ 10 kg mol−1), 7.5k
(Mn ∼ 7.5 kg mol−1), 5k
(Mn ∼ 5 kg mol−1), 10k★ (Mn ∼ 10 kg mol−1), 7.5k★ (Mn ∼ 7.5 kg mol−1), and 5k★ (Mn ∼ 5 kg mol−1). As macromer Mn was reduced, linear-scaffolds PCL-DA did not show a reduction in Tm (∼51–56 °C) owing to insufficient decreases in % crystallinity: 10k
(∼46%) versus 7.5k
(∼37%) and 5k
(∼35%). However, star- scaffolds exhibited substantial and progressive decreases in Tm as Mn was decreased, imparted by diminished % crystallinity: 10k★ (∼46 °C/∼27%), 7.5k★ (∼40 °C/∼22%), and 5k★ (∼29 °C/∼19%). While crosslink density increased with decreasing Mn, the corresponding reduction of % crystallinity was the driving factor in the observed trends in material properties. Star- scaffolds exhibited faster rates of degradation that also increased with decreasing Mn, a feature beneficial to the noted device applications. Due to the reduction of crystallinity, the mechanical properties of star- scaffolds were diminished relative to linear- scaffolds, but exceeded that of common biomedical hydrogels explored in such applications. The shape memory behavior of star- scaffolds were largely retained, with a slight decrease for 5k★. The radial expansion forces of star- scaffolds exceeded that of linear- scaffolds wherein shape recovery was facilitated by faster melting of the formers’ crystalline lamellae present at lower percentages. While the utility of star-PCL macromers to impart the desired Tm was demonstrated with porous scaffolds via SCPL, this platform may be used in a variety of fabrication methods and final product forms to achieve thermally-modulated shape memory devices with tissue safety.
Author contributions
The manuscript was written through contributions of all authors. All authors have given approval to the final version of the manuscript.
Conflicts of interest
There are no conflicts to declare.
Acknowledgements
This work was supported by NIH 1R21HD104059-01A1. The use of the Texas A&M Microscopy and Imaging Center (MIC) is acknowledged. SEM acquisition was supported in part by the National Science Foundation under Grant No. DBI-0116835.
References
- M. R. Pfau and M. A. Grunlan, J. Mater. Chem. B, 2021, 9, 4287–4297 RSC.
- J. Delaey, P. Dubruel and S. Van Vlierberghe, Adv. Funct. Mater., 2020, 30, 1909047 CrossRef CAS.
- R. Dwivedi, S. Kumar, R. Pandey, A. Mahajan, D. Nandana, D. S. Katti and D. Mehrotra, J. Oral Biol. Craniofac. Res., 2020, 10, 381–388 CrossRef PubMed.
- M. Labet and W. Thielemans, Chem. Soc. Rev., 2009, 38, 3484–3504 RSC.
- M. C. Serrano and G. A. Ameer, Macromol. Biosci., 2012, 12, 1156–1171 CrossRef CAS PubMed.
- J. Delaey, P. Dubruel and S. Van Vlierberghe, Adv. Funct. Mater., 2020, 30, 1909047 CrossRef CAS.
- D. Zhang, O. J. George, K. M. Petersen, A. C. Jimenez-Vergara, M. S. Hahn and M. A. Grunlan, Acta Biomater., 2014, 10, 4597–4605 CrossRef CAS PubMed.
- L. N. Nail, D. Zhang, J. L. Reinhard and M. A. Grunlan, J. Visualized Exp., 2015, 105, e52981 Search PubMed.
- M. R. Pfau, F. O. Beltran, L. N. Woodard, L. K. Dobson, S. B. Gasson, A. B. Robbins, Z. T. Lawson, W. B. Saunders, M. R. Moreno and M. A. Grunlan, Acta Biomater., 2021, 136, 233–242 CrossRef CAS PubMed.
- M. Can, S. Koluaçik, E. Bahçe, H. Gokce and F. S. Tecellioglu, J. Mech. Behav. Biomed. Mater., 2022, 126, 105030 CrossRef PubMed.
- G. Augustin, T. Zigman, S. Davila, T. Udilljak, T. Staroveski, D. Brezak and S. Babic, Clin. Biomech., 2012, 27, 313–325 CrossRef PubMed.
- K. Kurata, J. Matsushita, A. Furuno, J. Fujino and H. Takamatsu, J. Orthop. Res., 2017, 35, 2799–2807 CrossRef CAS PubMed.
- E. B. Dolan, M. G. Haugh, M. C. Voisin, D. Tallon and L. M. McNamara, PLoS One, 2015, 10, e0119652 CrossRef PubMed.
- M. Wancura, J. M. McCracken, E. Steen, E. Cosgriff-Hernandez, S. Keswani and J. C.-E. Hakim, Curr. Opin. Gynecol. Obstet., 2019, 31, 309–316 CrossRef PubMed.
-
A. Kamatar, A. Robinson, C. Roberts, F. Beltran, J. McCracken, M. Grunlan, J. Hakim and E. Cosgriff-Hernandez, Society for Biomaterials, Baltimore, MD, 26–30, 2022 Search PubMed.
- L. Peponi, I. Navarro-Baena, J. E. Báez, J. M. Kenny and A. Marcos-Fernandez, Polymer, 2012, 53, 4561–4568 CrossRef CAS.
- I. Navarro-Baena, A. Marcos-Fernández, A. Fernández-Torres, J. M. Kenny and L. Peponi, RSC Adv., 2014, 4, 8510–8524 RSC.
- Y. Ren, Z. Wei, X. Leng, T. Wu, Y. Bian and Y. Li, J. Phys. Chem. B, 2016, 120, 4078–4090 CrossRef CAS PubMed.
- S. Corneillie and M. Smet, Polym. Chem., 2015, 6, 850–867 RSC.
- J. M. Ren, T. G. McKenzie, Q. Fu, E. H. H. Wong, J. T. Xu, Z. S. An, S. Shanmugam, T. P. Davis, C. Boyer and G. G. Qiao, Chem. Rev., 2016, 116, 6743–6836 CrossRef CAS PubMed.
- R. J. Mondschein, A. Kanitkar, C. B. Williams, S. S. Verbridge and T. E. Long, Biomaterials, 2017, 140, 170–188 CrossRef CAS PubMed.
- Y. Sakamoto and H. Tsuji, Polymer, 2013, 54, 2422–2434 CrossRef CAS.
- E. S. Kim, B. C. Kim and S. H. Kim, J. Polym. Sci. Polym. Phys., 2004, 42, 939–946 CrossRef CAS.
- X. Y. Zhu, Y. F. Zhou and D. Y. Yan, J. Polym. Sci. Polym. Phys., 2011, 49, 1277–1286 CrossRef CAS.
- M. R. Pfau, K. G. McKinzey, A. A. Roth, L. M. Graul, D. J. Maitland and M. A. Grunlan, J. Mater. Chem. B, 2021, 9, 3826–3837 RSC.
- Y. Li and T. Kissel, Polymer, 1998, 39, 4221–4447 Search PubMed.
- A. Breitenbach, Y. X. Li and T. Kissel, J. Controlled Release, 2000, 64, 167–178 CrossRef CAS PubMed.
- J. Burke, R. Donno, R. d'Arcy, S. Cartmell and N. Tirelli, Biomacromolecules, 2017, 18, 728–739 CrossRef CAS PubMed.
- J. F. Douglas, J. Roovers and K. F. Freed, Macromolecules, 1990, 23, 4168–4180 CrossRef CAS.
- Y. Lu, L. J. An and Z. Wang, Macromolecules, 2013, 46, 5731–5740 CrossRef CAS.
- M. T. Frassica, S. K. Jones, P. Diaz-Rodriguez, M. S. Hahn and M. A. Grunlan, Acta Biomater., 2019, 99, 100–109 CrossRef CAS PubMed.
- C. G. Pitt, F. I. Chasalow, Y. M. Hibionada, D. M. Klimas and A. Schindler, J. Appl. Polym. Sci., 1981, 26, 3779–3787 CrossRef CAS.
- M. A. Wierzbicki, J. Bryant, M. W. Miller, B. Keller and D. J. Maitland, J. Mech. Behav. Biomed. Mater., 2016, 59, 156–167 CrossRef PubMed.
- N. Abbasi, S. Hamlet, R. M. Love and N. T. Nguyen, J. Sci. Adv. Mater. Dev., 2020, 5, 1–9 Search PubMed.
- Y. Shirakata, T. Nakamura, Y. Shinohara, K. Taniyama, K. Sakoda, T. Yoshimoto and K. Noguchi, J. Mater. Sci.: Mater. Med., 2014, 25, 899–908 CrossRef CAS PubMed.
- C. Bosch, B. Melsen and K. Vargervik, J. Craniofac. Surg., 1998, 9, 310–316 CrossRef CAS PubMed.
- A. Bandyopadhyay, P. K. Valavala, T. C. Clancy, K. E. Wise and G. M. Odegard, Polymer, 2011, 52, 2445–2452 CrossRef CAS.
- B. D. Ulery, L. S. Nair and C. T. Laurencin, J. Polym. Sci. Polym. Phys., 2011, 49, 832–864 CrossRef CAS PubMed.
- E. Gomez-Barrena, P. Rosset, D. Lozano, J. Stanovici, C. Ermthaller and F. Gerbhard, Bone, 2015, 70, 93–101 CrossRef PubMed.
- M. S. Ghiasi, J. Chen, A. Vaziri, E. K. Rodriguez and A. Nazarian, Bone Rep., 2017, 6, 87–100 CrossRef PubMed.
- G. M. Grimsby and L. A. Baker, Curr. Urol. Rep., 2014, 15, 428 CrossRef PubMed.
- M. T. Frassica, C. J. Demott, E. M. Ramirez and M. A. Grunlan, ACS Macro Lett., 2020, 9, 1740–1744 CrossRef CAS PubMed.
Footnote |
† Electronic supplementary information (ESI) available: NMR spectra, DSC data, crosslink density tests, macromer solution viscosity and diffusion through salt template, sol content, porosity, thermal properties, shape fixity & recovery, radial expansion pressures, degradation data, and mechanical data. See DOI: https://doi.org/10.1039/d4tb00050a |
|
This journal is © The Royal Society of Chemistry 2024 |