DOI:
10.1039/D3TB02674D
(Perspective)
J. Mater. Chem. B, 2024,
12, 2720-2736
Trends in bioactivity: inducing and detecting mineralization of regenerative polymeric scaffolds
Received
10th November 2023
, Accepted 14th February 2024
First published on 16th February 2024
Abstract
Due to limitations of biological and alloplastic grafts, regenerative engineering has emerged as a promising alternative to treat bone defects. Bioactive polymeric scaffolds are an integral part of such an approach. Bioactivity importantly induces hydroxyapatite mineralization that promotes osteoinductivity and osseointegration with surrounding bone tissue. Strategies to confer bioactivity to polymeric scaffolds utilize bioceramic fillers, coatings and surface treatments, and additives. These approaches can also favorably impact mechanical and degradation properties. A variety of fabrication methods are utilized to prepare scaffolds with requisite morphological features. The bioactivity of scaffolds may be evaluated with a broad set of techniques, including in vitro (acellular and cellular) and in vivo methods. Herein, we highlight contemporary and emerging approaches to prepare and assess scaffold bioactivity, as well as existing challenges.
1. Introduction
1.1. Current methods to treat bone defects
Bone tissue is critical for mechanical functionality, protection, and hematopoiesis.1,2 These traits stem from its unique combination of carbonated hydroxyapatite (HAp) [Ca10(PO4)6(OH)2] embedded in an extracellular matrix (ECM) comprised of collagen (primarily type I), proteoglycans, and glycoproteins. While capable of regeneration, bone tissue healing is limited for defects beyond a critical size that stem from traumatic injury, surgical excision, or congenital anomalies.3 Bone tissue healing is also hindered by advanced age, osteoarthritis, and radiological treatment. Numerous products have been developed to treat and heal critical-sized bone defects, and are exemplified in Table 1.
Table 1 Examples of commercial biological and alloplastic materials to treat bone defects
Product name |
Bioactive component |
Matrix/carrier component |
AlloFuse® (AlloSource) |
DBM |
Reverse phase medium gel |
Calcigen® S (Zimmer Biomet) |
Calcium sulfate |
Not reported |
Cerament® (BONESUPPORT) |
HAp & calcium sulfate |
Not reported |
CranioSculpt™ (KLS Martin) |
Calcium phosphate |
Not reported |
Cortoss® (Stryker) |
Bioactive glass ceramic |
Acrylate copolymer |
Grafton™ DBM (Medtronic) |
DBM |
Glycerol |
Kinex® (Globus Medical) |
BG |
Collagen, hyaluronic acid |
MinerOss® (BioHorizons) |
Cortical & cancellous bone chips |
None |
NanoFUSE® (Amend Surgical) |
DBM & BG-45S5 coated with gelatin |
None |
Optium® (LifeNet Health) |
DBM |
Glycerol |
OSferion (Arthrex) |
β-TCP |
None |
Osteosponge® (XTANT Medical) |
DBM |
None |
Puros® (ZimVie) |
DBM |
Reverse phase medium gel |
Vestakeep® Fusion (Evonik) |
Biphasic calcium phosphate |
PEEK |
Biological grafting approaches are frequently employed, wherein the living tissue graft becomes incorporated into the surrounding tissue. Autografting remains the ‘gold standard’ with over two million bone autografts performed annually.4 However, autografting is associated with complex surgical harvesting (e.g., from tibia or iliac crest), donor site morbidity, and limited availability, as well as premature resorption stemming from poor contact with adjacent tissue. Cadaveric allografts are also associated with limited availability and premature resorption, as well as immune rejection.4 Xenografts, particular bovine grafts, have been explored but pose a risk for disease transmission, a greater chance of host immune response, highly variable resorption rates, and reduction in osteoinductive properties due to strict manufacturing and processing requirements.5,6 Alloplastic bone substitutes have been leveraged as an alternative to biological grafts. For example, demineralized bone matrix (DBM) is prepared via decalcification (i.e., removal of HAp) of cortical bone allografts with an acidic solution that leaves behind a composite of collagens, non-collagenous proteins, growth factors, residual calcium phosphate mineral (1–6%), and trace cellular debris.7 Alloplastic grafts have also been prepared based on one or more synthetic bioactive “bioceramics”, including: bioactive glasses (BGs),8,9 HAp10–12 β-tricalcium phosphate [β-Ca3(PO4)2; β-TCP],13,14 and calcium sulfate.15 Silicate BGs are widely used for their capacity to bond to bone, and represent certain compositions of SiO2–Na2O–CaO–P2O5 (e.g., 45S5-BG). In the granular form, DBM and bioceramics provide advantageous microporosity and complete resorption. Still, these are also often combined with a polymer coating or matrix to afford injectability or moldability within irregular shapes, as well as to circumvent brittleness in certain cases.16 The use of poly(methyl methacrylate) (PMMA) is associated with exothermic cures, post-cure shrinkage, lack of porosity, and non-degradability, as well as brittle mechanical properties.17,18 To mitigate brittleness and afford replacement by neotissue, numerous degradable synthetic and natural polymers have been employed.19–22 Synthetic polyesters and copolymers thereof have been widely utilized given the tunability of properties.20 More recently, polyether ether ketone (PEEK) 3D printed alloplastic devices have been created in complex, patient-specific geometries,23 including as composites with bioactive fillers.24,25
1.2. Regenerative engineering approaches with bioactive scaffolds
Owing to the limitations of biological and alloplastic grafts, regenerative engineering approaches have emerged to heal bone defects.26 The scaffold compositions play an instrumental role, and must fulfill a demanding set of criteria to maximize bone tissue healing.27,28 Bioactivity is of significant importance as it leads to the formation of HAp that promotes osteogenic differentiation (i.e., osteoinductivity), as well as osseointegration with surrounding bone tissue (Fig. 1).29–31
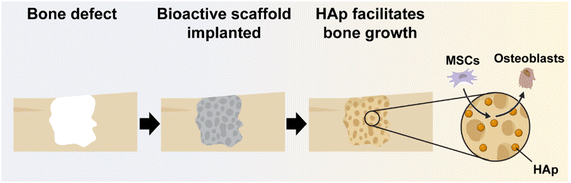 |
| Fig. 1 Bioactive scaffolds lead to HAp mineralization, and subsequently promote osteogenesis and osseointegration. | |
While bioactive HAp is innately present in biological grafts, for alloplastic grafts, bioactivity is traditionally afforded by the inclusion of DBM or bioceramics. Scaffolds that are potently bioactive may reduce or even eliminate the necessity of exogeneous growth factors (e.g., bone morphogenic protein 2, BMP-2), which risk off-target responses.32 Beyond bioactivity, the scaffold must also be osteoconductive (i.e., permitting cell migration and neotissue infiltration).33,34 This is achieved through porosity that may be afforded through a variety of fabrication methods (e.g., 3D printing, solvent cast particulate leaching [SCPL], gas foaming, freeze drying, and electrospinning) with polymers forming the regenerative bone scaffold.35 Degradation of the scaffold also facilitates osteoconductivity, making the rate of scaffold resorption important to healing. To enable osseointegration, the scaffold must form close contact with adjacent bone tissue.29 This has been particularly addressed with injectable scaffolds,36 3D-printed scaffolds,37,38 and shape memory polymer (SMP) scaffolds.39
2. Imparting bioactivity to scaffolds
Given the importance of bioactivity to bone regeneration, bioactive polymeric scaffolds continue to be developed (Fig. 2). Approaches include bioactive composite scaffolds based on combining polymers with bioactive fillers (e.g., DBM and bioceramics), bioactive coatings and surface treatments, as well as surface modification of scaffolds. Recent reports are exemplified herein.
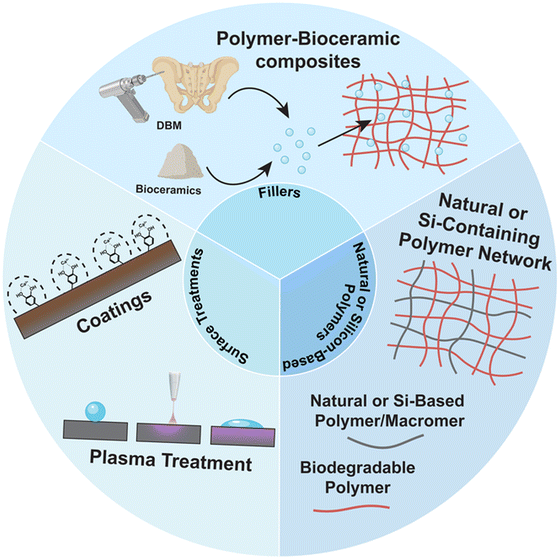 |
| Fig. 2 Methods to prepare bioactive polymeric scaffolds for bone regeneration. | |
2.1. Bioactive composite scaffolds
Bioactive composite scaffolds, comprised of one or more bioceramics embedded in a polymer matrix,40–42 remain prolific in regenerative bone engineering. Versus bioceramic-only scaffolds, these composites can improve processibility, increase rigidity and strength, and mitigate brittleness that contribute to post-surgical fracture. Furthermore, owing to the hydrophilicity and susceptibility to hydrolysis, bioceramic–polymer composites also degrade at favorably faster rates compared to polymer-only scaffolds. A variety of bioactive composite scaffolds have recently been reported (Table 2). Both biodegradable synthetic and natural polymers have been utilized. A variety of bioceramics and glasses have been leveraged, ranging from historically used types (e.g., BG, HAp, and β-TCP), to newer types (e.g., nanosilicates, and eggshell particles). Their mineralization activity and mechanism vary, with some capable of acellular mineralization in physiological environments including simulated body fluid (SBF) (Fig. 3). For instance, 45S5-BG [45 wt% SiO2, 24.5 wt% Na2O, 24.5 wt% CaO, and 6 wt% P2O5] is known to promote rapid HAp formation on its surface within hours.43 The mechanism involves a series of sequential steps: (1) exchange of Na+ ions with solution H+, (2) hydrolysis of Si–O–Si bonds to form SiOH bonds, and the release of Si(OH)4, (3) polycondensation to form a hydrated silica gel, (4) formation of an amorphous calcium phosphate phase via absorption of Ca2+, PO43−, and CO32− ions, and (5) crystallization to carbonated HAp. Synthetic HAp is also capable of inducing the formation of a HAp layer in SBF, and at greater levels versus BG-45S5.44 HAp forms through several steps: (1) adsorption of Ca2+ ions to the surface, (2) formation of Ca-rich amorphous calcium phosphate at surface, (3) transition to a Ca-poor surface due to adsorption of solution PO43− as well as CO32− ions, and (4) crystallization into carbonated HAp.45 In addition to bone bonding, the formed HAp layer facilitates bone formation via the stimulation of mesenchymal stem cell (MSC) osteogenesis, especially via increased expression of growth factors (e.g., BMP) and enhanced alkaline phosphatase (ALP) activity. In contrast, β-TCP does not mineralize with SBF exposure, but rather is osteoconductive and osteoinductive.14 β-TCP leads to osteoclast-mediated resorption and osteoconduction that is associated with rapid bone formation and high bone bonding strengths.
Table 2 Examples of bioactive composite scaffolds for bone regeneration
Study |
Bioactive component |
Matrix component |
Wt% of Filler |
Fabrication method |
Key findings |
Shuai, 202147 |
HAp |
PLLA, or PGA |
10% |
3D printing (SLS) |
HAp/PLLA/PGA scaffolds displayed tunable degradation and mechanical properties. |
Xu, 201748 |
HAp or β-TCP |
PLGA |
50% |
SCPL |
HAp/PLGA scaffolds exhibited higher strength and faster degradation; TCP/PLGA scaffolds improved bone regeneration in a rabbit model. |
Cheng, 202149 |
β-TCP (with cucurbitacin B) |
PLGA |
25% |
3D printing (low temperature rapid prototyping) |
TCP/PLGA/Cur-B scaffolds increased bone regeneration and angiogenesis in a rat model. |
Nyberg, 201750 |
HAp, β-TCP, DBM, or BO |
PCL |
30% |
3D printing (extrusion) |
DBM/PCL and BO/PCL scaffolds exhibited greater osteoinduction; PCL/HAp displayed higher compressive modulus. |
Shuai, 202251 |
PDLA-grafted HAp (g-HAp) |
PDLA |
0.5, 1, 2 or 4% |
3D printing (SLS) |
g-HAp/PDLA scaffolds exhibited increased modulus and strength. |
Sultan, 202252 |
45S5-BG |
PLA |
5% |
TIPS and 3D printing (extrusion) |
45S5-BG/PLA scaffolds exhibited increased strength. |
Nitschke, 202353 |
45S5-BG |
PCL, PLLA |
2.5 to 30% |
Modified SCPL |
45S5-BG/SMP scaffolds exhibited faster degradation, and retained shape memory behavior. |
Distler, 202054 |
45S5-BG |
PLA |
1–10 wt% |
FDM |
45S5-BG/PLA scaffolds exhibited decreased strength with increased BG wt%; triggered cellular osteogenesis. |
Monfared, 202255 |
45S5-BG and β-TCP |
Gelatin, and PVA |
35–40% (equal parts each) |
3D printing (extrusion) |
45S5-BG/TCP/Gelatin/PVA scaffolds exhibited increased compressive strength and faster degradation. |
Han, 202356 |
BBG |
PCL |
5–40% |
3D printing (SLS) |
BBG/PCL scaffolds with 20 wt% BGG exhibited optimal mechanical properties, and promoting regeneration & integration in a rabbit model. |
Hatton, 201957 |
ICIE16M-BG |
Alginate |
50% |
Freeze-drying |
ICI16M-BG/alginate scaffolds exhibited increased strength. |
Du, 201958 |
MBG |
SF, or PCL |
80% |
3D printing (extrusion) |
MBG/SF exhibited superior compressive strength, and greater heterotopic bone formation in a mouse model. |
Qi, 201759 |
MBG and calcium sulfate |
PCL |
70% |
3D printing (extrusion) |
MBG/CS scaffolds exhibited superior osteogenesis of cultured human bone marrow mesenchymal stem cells (hBMSCs) and greater bone formation in a rat model. |
Carrow, 201960 |
LAPONITE® |
PEOT/PBT |
5, 10, or 15% |
3D printing (extrusion) |
LAPONITE®/PEOT/PBT scaffolds exhibited decreased degradation rates versus copolymer-only scaffolds, as well as increased osteogenesis of hMSCs. |
Wu, 202161 |
Eggshell microparticles (ESP) |
Gel–MA |
ESP combined with 5% prepolymer solution |
Casting/UV cure |
ESP/Gel–MA scaffolds exhibited superior osteogenesis of cultured MC3T3-E1 pre-osteoblasts versus Gel–MA-only scaffold, and greater bone formation in a rat model. |
Huang, 202062 |
MWCNTs &/or nHAp |
PCL |
0.75% MWCNT & 20% nHAp |
3D printing (screw assisted extrusion) |
PCL/MWCNT/nHAp scaffolds exhibited increased modulus versus PCL-only scaffolds, and greater osteogensis of cultured hADSCs. |
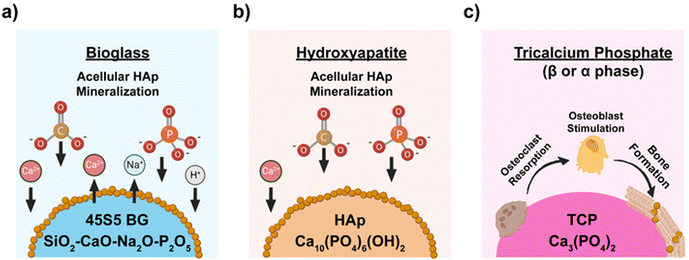 |
| Fig. 3 Simplified mechanisms of mineralization for common bioceramics: (a) BG-45S5, (b) synthetic HAp, and (c) β-TCP. | |
For bioactive composite scaffolds, the level of incorporated bioactive bioceramics is highly variable, ranging from less than 1 wt% to over 50 wt%. Composites prepared with two or more distinct types of bioceramics have also been reported. A number of fabrication methods have been employed (e.g., SCPL, electrospinning). Notably, various forms of 3D printing have been leveraged extensively to impart finer control of microarchitecture as well as to produce patient-specific scaffolds.46 For instance, selective laser sintering (SLS) 3D printing employs a laser to sinter a powder (e.g., a mixture of polymer and bioceramic) and fuse particles together, while unfused particles support the structure. Comparison of composite scaffold bioactivity efficacy is difficult, as in vitro and in vivo evaluations of such scaffolds are highly variable in the literature. For example, time-points selected to confirm HAp mineralization following exposure to SBF vary appreciably (from 1 day to several weeks). Still, some studies directly compare scaffolds prepared with two different bioceramics.
Both HAp and β-TCP continue to be utilized to prepare bioactive composite scaffolds, either alone or in combinations. Shuai et al. utilized SLS 3D printing to fabricate composite scaffolds based on HAp (10 wt%) with poly(L-lactic acid) (PLLA) and poly(glycolic acid) (PGA).47Versus HAp/PLLA composite scaffolds, the faster degradation of HAp/PLLA/PGA composites (50
:
50 wt% PLLA
:
PGA) enhanced the exposure of HAp, leading to superior mineralization and regeneration in a rabbit segmental defect model. Xu et al. reported both HAp- and β-TCP-containing poly(lactic-co-glycolic acid) (PLGA) composite scaffolds via SCPL.48 Compressive tests revealed that HAp-scaffolds had higher strengths and rates of in vitro degradation compared to β-TCP-scaffolds. In a rabbit calvarial defect, while early-stage bone growth was faster for HAp-scaffolds, β-TCP-scaffolds exhibited greater bone mineral densities and higher compressive strengths of repaired bone at 20 weeks. Cheng et al. introduced cucurbitacin B, a plant-derived terpene, to β-TCP/PLGA scaffolds leading to enhanced osteogenesis and neovascularization.49 In an example by Nyberg et al., a series of 3D printed composite poly(ε-caprolactone) (PCL) scaffolds were prepared by altering the bioactive filler: a bioceramic (HAp or β-TCP) or a biologic (decellularized bone matrix [DBM] or Bio-Oss® [BO], a clinically available form of DBM).50 BO/PCL and DBM/PCL scaffolds exhibited enhanced osteoinduction versus HAp/PCL and TCP/PCL scaffolds, while the compressive modulus was highest for HAp/PCL scaffolds. Shuai et al. reported poly(D,L-lactic acid) (PDLLA) grafted onto HAp (g-HAp), combined with PLLA to prepare composite scaffolds via SLS 3D printing.51 As a result of improved interfacial bonding via stereo-complexation, g-HAp/PLLA composite scaffolds displayed significant enhancements in stiffness and strength.
BGs continue to be utilized to prepare bioactive composite scaffolds. Sultan et al. prepared composite scaffolds based on 45S5-BG (5 wt%) and poly(lactic) acid (PLA).52 Thermally induced phase separation (TIPS) was used to create a homogenous distribution of BG, and the resulting composite spheres were subsequently formed into scaffolds via extrusion 3D printing. Due to the homogeneous distribution of BG, scaffold compressive strength increased for printed scaffolds.
We have previously reported ‘self-fitting’ SMP scaffolds based on PCL,63 including PCL/PLLA semi-interpenetrating networks (semi-IPNs).64 Recently, Nitschke et al. reported analogous composite scaffolds that included 45S5-BG.53 A modified SCPL protocol was utilized wherein the fused template was formed from a mixture of BG and salt, resulting in localization of BG on the scaffold pore walls. At just 5 and 10 wt% BG, 45S5-BG/PCL scaffolds induced HAp mineralization after 1 day in SBF (1X), and degraded faster versus corresponding polymer-only scaffolds, all while maintaining shape memory behavior. Distler et al. described 45S5-BG/PLA scaffolds with 1–10 wt% BG formed by fused deposition modeling (FDM) of 45S5-BG/PLA filaments.54 Monfared et al. combined 45S5-BG with β-TCP (50
:
50 wt% ratio; 35–45 wt% total) to prepare composite scaffolds based on gelatin, poly(vinyl alcohol) (PVA), and Tween® 60 using extrusion-based 3D printing.55 The printed scaffolds achieved higher moduli versus analogous scaffolds prepared by foam casting.
Newer types of bioceramics have also been developed and used to prepare bioactive composite scaffolds. For example, borate-containing BGs (BBGs), wherein borate (B2O3) is partially or completely substituted for silica (SiO2), are associated with faster rates of HAp formation compared to silicate bioactive glasses (e.g., 45S5).65 Thus, BBG composite scaffolds have been formed, as in Han et al. wherein BBG/PCL scaffolds were prepared by SLS 3D printing.56 Furthermore, ICIE16-BG, a potassium-containing BG (48.0 SiO2, 6.6 Na2O, 32.9 CaO, 2.5 P2O5, 10.0 K2O [wt%]), was developed as an alternative to 45S5-BG.57 For instance, Hatton et al. combined ICIE16-BG with alginate to form composite scaffolds via freeze-drying.66 Still, different types of bioactive silicate bioceramics have been leveraged to form bioactive composite scaffolds. Mesoporous bioactive glasses (MBG) based on silicates have well-defined pores with diameters around 5 to 20 nm, presenting a large surface area.67 Du et al. 3D printed composite scaffolds from MBG (80 wt%) combined with silk fibroin (SF) and PCL, with MBG/SF scaffolds exhibiting superior strength and bioactivity versus MBG/PCL scaffolds.58 While relatively low cost, calcium sulfate exhibits particularly rapid resorption as well as limited bioactivity.68 Thus, Qi et al. prepared 3D printed scaffolds wherein MBG and calcium sulfate were combined with PCL.59 In addition to MBG, other nanosized bioactive fillers have also been utilized to form bioactive scaffolds,69 including HAp nanoparticles,70 and nanosilicates (e.g., LAPONITE®).71,72 Carrow et al. reported 3D printed scaffolds using LAPONITE® in combination with a poly(ethylene oxide terephthalate) (PEOT)/poly(butylene terephthalate) (PBT) (PEOT/PBT) copolymer.60 Wu et al. reported the use of chicken eggshell microparticles (ESP), possessing high levels of calcium and representing a sustainable alternative to BGs, to form bioactive composite scaffolds in combination with gelatin methacrylate (Gel–MA).61 To achieve biomimetic scaffolds in terms of not only bioactivity but structure, Huang et al. prepared PCL scaffolds with multi-walled carbon nanotubes (MWCNTs) and nano-HAp (nHAp), using screw-assisted extrusion based 3D printing to align the MWCNTs.62 Piezoelectric perovskites such as barium titanate (BTO) have also shown to be capable of HAp mineralization.73 In the case of BTO, when exposed to an electric field or mechanical stress, the titanium and oxygen ions switch locations, leading to a concentration of negatively charged O2− on the material surface. In turn, positively charged Ca2+ ions from the physiologic fluid are attracted to the surface, leading to the formation of an apatite layer.74
2.2. Bioactive coatings and surface treatments
Various bioactive coatings have historically been applied to metal implants.75 To achieve bioactivity of polymeric scaffolds, coatings have been likewise applied.76,77 Such coatings can enhance the bioactivity of composite scaffolds, or be used in lieu of fillers to avoid brittleness. Bioceramic coatings may also be directly applied to scaffolds. Recently, several bioactive coatings and surface treatments have been applied to scaffolds to promote bioactivity (Table 3). For instance, Fazeli et al. reported the deposition of HAp and BG onto 3D printed PCL scaffolds via an immersion method with a HAp/BG solution.78 Zhang et al. reported PCL scaffolds coated with HAp via pulsed laser deposition (PLD).79 Li et al. described HAp deposition onto PVA/PLA scaffolds via electrodeposition.80 Coatings based on a combination of bioceramics and polymers have also been reported. For instance, based on the bioactivity of chitosan,81 Shaltooki et al. applied a chitosan/BG coating to PCL/BG composite scaffolds by exposing scaffolds to homogenized solutions.82 Alternatively, a bioactive polymeric coating may be applied to scaffolds. Collagen type I, a natural component of bone tissue, is a frequently used material for bone regeneration.83 Thus, Tabatabaei et al. reported PCL/β-TCP scaffolds coated by collagen using an immersion method that included homogenization.84 While developed for the purpose of assessment of biomaterial bioactivity, SBF exposure has been used to deposit bioactive mineral coatings onto polymeric scaffolds.76,85 Polydopamine (PDA) was established to readily form adherent coatings onto substrates via base-catalyzed autooxidation of dopamine.86,87 PDA was confirmed to induce HAp mineralization in SBF,88 leading to its use as a coating to create bioactive scaffolds for bone regeneration. Numerous studies have been noted in a recent review by Tolabi et al., with the scaffold polymer component frequently being a biodegradable polyester.89 We likewise applied a PDA coating to polyester SMP scaffolds, which resulted in enhanced osteogenic differentiation of hMSCs as well as HAp mineralization after SBF exposure.90
Table 3 Recent studies in bioactive coatings, surface treatment, and polymers
|
Study |
Bioactive coating |
Matrix component |
Coating method |
Key findings |
Coatings |
Fazeli, 202378 |
HAp |
PCL |
Immersion in HAp solution. |
HAp-coated scaffolds displayed increased osteogenesis in rat calvarial defect models. |
Zhang, 202279 |
HAp |
PCL |
PLD |
Increased osteogenic potential of HAp-coated scaffolds cultured with rat BMSCs, and superior bone regeneration in critical rat calvarial defects. |
Li, 201980 |
HAp |
PVA/PLA |
Electrodeposition |
Longer electrodeposition times resulted in increased surface roughness and higher crystallinity of HAp. |
Shaltooki, 201982 |
Chitosan/BG |
PCL |
Immersion of scaffolds in chitosan/BG solution |
Chitosan/BG-coated scaffolds displayed increased hydrophilicity, degradation, and osteogenic properties. |
Tabatabaei, 202284 |
Collagen |
PCL/β-TCP |
Immersion in homogenized collagen solution |
Collagen-coated scaffolds displayed enhanced hydration and osteogenic potential. |
Arabiyat, 202190 |
PDA |
PCL, PCL/PLLA |
Submersion in PDA solution |
PDA-coated PCL/PLLA scaffolds showed acellular mineralization in SBF, and higher osteogenesis with cultured h-MSCs. |
|
|
Bioactive surface treatment |
Matrix component |
|
Surface treatment |
Kim, 201495 |
O2 plasma |
PCL |
Increased hydrophilicity and surface roughness, acellular mineralization in SBF, and increased osteogenesis with cultured mouse pre-osteoblast cells (MC3T3-E1). |
Murab, 202096 |
O2 plasma |
PCL/TCP |
O2 plasma treated scaffolds presented –COOH groups as nucleation sites for HAp formation. |
Yamada, 202197 |
O2 plasma |
PLA-co-TMC |
O2 plasma scaffolds had increased surface roughness and hydrophilicity, and improved osteogenesis of cultured rat BMSCs. |
Terriza, 201498 |
PECVD |
PLGA |
15 nm SiO2 film deposition onto PLGA films produced changes to osteoblast morphology. |
Song, 202399 |
iCVD |
HAp |
iCVD treatment coated scaffolds with a polyelectrolyte induced mineralization and enhanced osteogenesis of cultured MC3T3-E1s. |
|
|
Bioactive additive |
Matrix component |
Scaffold fabrication method |
|
Bioactive additives |
Jose, 2009100 |
Collagen |
PLGA |
Electrospinning |
Increased elastic modulus for crosslinked collagen, especially for PLGA/collagen 80/20. |
Wang, 2021101 |
Gelatin |
PCL |
MEW and SE |
Increased hydrophilicity and osteogenic potential in vitro compared to PCL-only nanofibers. |
Amiryaghoubi, 2022102 |
Chitosan |
PCL/PU |
Freeze-drying method |
PCL/PU/chitosan scaffolds exhibited increased hydrophilicity, surface roughness, and promoted osteogenic differentiation of hBMSCs. |
Ren, 2021103 |
Fibrin/alginate |
PCL |
MEW of PCL followed by coating with FA foam |
PCL/FA scaffolds exhibited superior angiogenesis in an in vitro model and increased bone regeneration in rat cranial defects. |
Jang, 2020104 |
Hyaluronic acid |
PCL |
Spray-precipitation of microspheres |
PCL/HA microspheres exhibited increased osteogenic potential in vitro and bone regeneration after implantation in critically sized calvarial defect in a rat model. |
Frassica, 2019105 |
PDMSstar–MA |
PEG–DA |
Solvent induced phase separation (SIPS) |
PDMSstar/PEG hydrogels displayed acellular mineralization in SBF, and osteogenesis of hBMSCs. |
Frassica, 2020106 |
PPMS–DA |
PEG–DA |
SIPS |
PPMS/PCL scaffolds exhibited superior osteogenic potential in vitro compared to PEG and PEG/PDMS scaffolds. |
Beltran, 2021107 |
PDMS–DMA |
PCL–DA |
SCPL |
PDMS-containing scaffolds mineralized in 1X SBF and presented accelerated degradation rates. |
Beltran, 2023108 |
PMHS–DMA |
PCL–DA |
SCPL |
PMHS-containing scaffolds had a faster onset of acellular mineralization in SBF, and expedited acceleration profiles compared to analogous PDMS scaffolds. |
Direct surface treatment of polymeric scaffolds has also been utilized to invoke bioactivity.76,91 Plasma treatment, wherein an electric current passes through a gas (e.g., oxygen, argon, and ammonia), is a popular method.92 This process can be used to produce surface functionalization, etching, or film deposition, while maintaining scaffold bulk properties.93 Oxygen plasma treatment has been extensively utilized, including for PCL-based scaffolds, to enhance surface hydrophilicity and surface energy for improved cellular adhesion.94 Depending on plasma parameters and polymer type, oxygen plasma treatment can result in both surface functionalization and etching.93 For instance, Kim et al. reported oxygen plasma treatment of PCL scaffolds resulting in enhanced hydrophilicity and surface roughening.95 These scaffolds exhibited in vitro mineralization by cultured cells. To confirm that such surfaces also give rise to acellular mineralization, Murab et al. used oxygen plasma treated PCL/TCP scaffolds exposed to SBF to demonstrate that resulting –COOH groups act as nucleation sites for amorphous calcium phosphate to form HAp crystals.96 A significant challenge of plasma generated polymeric surfaces is their age-instability, as surfaces hydrophobically recover to the untreated state within as little as hours.109 However, some examples display improved stability. For instance, Yamada et al. reported oxygen plasma treated PLA-co-trimethylene carbonate (PLA-co-TMC) scaffolds whose surfaces were stable for over 2 weeks.97 Plasma-enhanced chemical vapor deposition (PECVD) may be utilized to apply thin films, including inorganic–organic composite films.110 However, recent reports on its use to enhance polymeric bioactivity appear scarce. Terriza et al. used deposition by PECVD of SiO2 onto the surfaces of PLGA membranes, resulting in morphological changes to osteoblasts.98 Another form of CVD, initiated CVD (iCVD), employs a combination of a volatile initiator as well as monomer(s) to produce thin films.110 This process avoids fragmentation of organic precursors as with PECVD. Song et al. reported formation of a polyelectrolyte coating via iCVD onto HAp scaffolds that was then exposed to supersaturated HAp, resulting in mineralized scaffolds that promoted osteogenesis.99
2.3. Bioactive polymers as additives
As previously noted, a variety of synthetic and natural polymers are utilized to form bone tissue scaffolds. Most of these are considered “nearly inert”, or lacking in bioactivity to promote bone regeneration.111 Yet, several natural polymers (e.g., collagen,112 gelatin,113 chitosan,81 alginate,114 and hyaluronic acid115) display bioactivity, and have thus been formed into regenerative bone scaffolds (Table 3). In addition to being used as coatings, these bioactive polymers may be used as an additive in combination with a nearly inert polymer to form the scaffold bulk or a discrete structure within the inert polymer. In some cases, bioactivity is demonstrated in the absence of bioactive fillers. For instance, collagen has been blended with polyesters to form electrospun scaffolds,116 including for bone regeneration per Jose et al.100 Wang et al. combined gelatin with PCL to produce scaffolds with hierarchal morphological structures using melt electrospinning writing (MEW) and solution electrospinning (SE).101 Amiryaghoubi et al. introduced chitosan to PCL/polyurethane (PU) to form scaffolds via freeze-drying.102 Ren et al. prepared PCL scaffolds via MEW and was subsequently impregnated with fibrin/alginate (FA).103 Jang et al. reported PCL/hyaluronic acid microspheres that were embedding into a tissue defect using an in situ gelling alginate hydrogel.104
Inspired by bioactive silicates, we have utilized silicon-based synthetic polymers as bioactive additives to form bone tissue scaffolds. While poly(ethylene glycol) (PEG) hydrogels have been evaluated for bone regeneration, they lack innate bioactivity.117 Thus, star-polydimethylsiloxane methacrylate (PDMSstar–MA) and PEG–diacrylate (PEG–DA) macromers were combined to form templated PDMS–PEG hydrogels that exhibited acellular mineralization when exposed to SBF, as well as enhanced osteogenesis of cultured hBMSCs.105 Greater bioactivity was observed for phosphonated-siloxane PEG hydrogels prepared with a poly(diethyl(2-(propylthio)-ethyl)phosphonate methylsiloxane)–diacrylate (PPMS–DA) macromer.106 Finally, we sought to induce bioactivity to our previously reported PCL SMP scaffolds.39 In the first study, PDMS–dimethacrylate (DMA) was combined with PCL–DA at varying wt% ratios (90
:
10, 75
:
25, and 60
:
40), giving rise to PCL–PDMS scaffolds.107 These maintained shape memory behavior, but displayed acellular mineralization with SBF exposure, as well as enhanced degradation rates due to phase separation effects. In a subsequent study, towards the goal of enhancing bioactivity, polymethylhydrosiloxane–dimethacrylate (PMHS–DMA) was utilized to form analogous PCL–PMHS scaffolds.108 The increased hydrophilicity of PMHS versus PDMS, stemming from the capacity of silane (Si–H) groups to form dihydrogen bonding with hydroxyl (–OH) groups of water, was expected to better parallel hydrophilic bioactive silicates. Indeed, PCL–PMHS scaffolds exhibited enhanced rates of HAp mineralization, as well as in vitro degradation rates. However, incorporation of a PEG-tethered cell adhesive peptide to PCL–PMHS scaffolds resulted in less presentation at the surface, reducing cellular adhesion.
For the future development of bioactive materials, the combination of high throughput screening (HTS)118,119 along with artificial intelligence (AI),120 specifically machine learning (ML),121 has great potential. ML used to analyze data from HTS assays can be critical in predicting properties of regenerative scaffolds, with different material combinations. For HTS and ML to be effective toward generating and predicting attributes of bioactive scaffolds, there must be standardized protocols for testing, which is currently lacking.122 In addition to selecting optimal material combinations, AI can be helpful in determining suitable 3D printing fabrication techniques and scaffold structure depending on the patient and location of the bone defect. Integrating AI with computer-aided design (CAD) to 3D print patient-specific scaffolds, especially with complex geometries, can reduce the time to generate scaffold structures and facilitate the selection of scaffold parameters (e.g., pore size, percent porosity, strut size) to mimic the adjacent trabecular or cortical bone. For example, after an imaging modality (e.g., CT scan) is used to identify the exact geometry of the defect, the AI integrated CAD system could generate a 3D scaffold that perfectly fits into the bone defect to enable proper osseointegration and angiogenesis through the scaffold.123 Furthermore, the use of AI techniques to determine optimal scaffold parameters during fabrication can pave the way for more thorough in vitro and in vivo assessments of the most promising scaffold compositions.
3. Assessment of scaffold of bioactivity
A number of analyses have been used to assess scaffold bioactivity, namely via acellular HAp formation, in vitro cellular behavior, and in vivo bone formation (Fig. 4). Assessment of acellular bioactivity should also consider scaffold sterilization and any pre-treatments (e.g., pre-wetting with ethanol graded baths) to be used for subsequent in vitro cell culture or in vivo assessment.
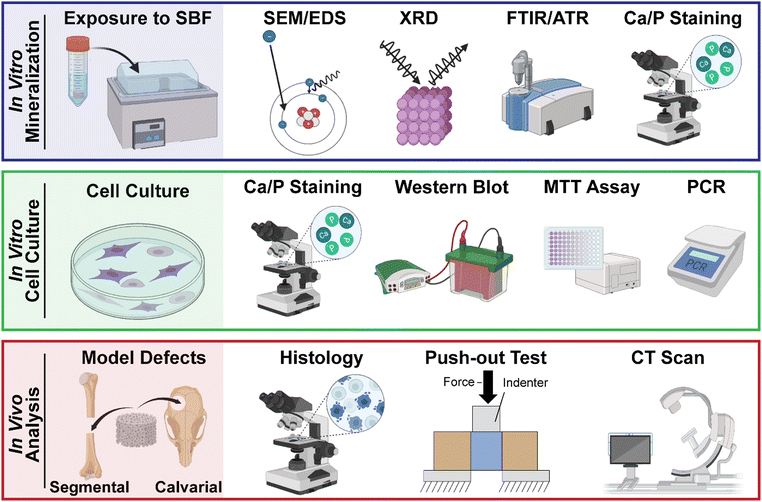 |
| Fig. 4 Generalized methods for assessing scaffold bioactivity: (top) acellular mineralization via exposure to SBF, characterization of HAp mineralization, (middle) cell culture, and (bottom) in vivo analyses using bone defect models. | |
3.1.
In vitro (acellular) assessment of scaffold bioactivity
Immersion of scaffolds to induce mineralization.
SBF has been widely adopted to measure bioactivity of materials, including per ISO 23317.124 Exposure to SBF, which is acellular and protein-free, is frequently utilized to confirm scaffold bioactivity in terms of HAp formation.14,44,125 Developed by Kokubo in 1991,126 SBF mimics the inorganic composition of human plasma (e.g., Mg2+, Ca2+, Na+, and K+) with a physiological pH (∼7.4), and is used at body temperature (∼37 °C). Kokubo et al. suggested the SBF volume (in mL) be greater than 1/10 the surface area of a porous material (in mm2).44 As a means to accelerate mineralization, SBF of higher concentrations have been utilized.85 However, highly concentrated SBF solutions can produce uneven, localized precipitation onto surfaces, and also exhibit spontaneous precipitation. This may be somewhat mitigated by increasing the temperature127 or decreasing pH of concentrated SBF solution.128 Due to the labor-intensive preparation of SBF, alternatives have been explored. Dulbecco's modified Eagle medium (DMEM), a commercially available cell culture medium, possesses ion concentrations similar to that of human blood plasma.129 α-TCP, β-TCP, and HAp were each incubated in DMEM (37 °C, 5% CO2) for 4 days, leading to CaP precipitate on these surfaces. However, it was noted that the presence of serum can decrease the rate of precipitation.
Scanning electron microscopy (SEM)/energy-dispersive X-ray spectroscopy (EDS).
SEM/EDS may be used in conjunction to evaluate the mineralized surface of bioactive scaffolds, including after immersion in SBF. SEM is frequently utilized to provide images of scaffold morphology and microstructure.130 Coupling with EDS affords determination of elemental composition of the surface.131 Briefly, when the surface is penetrated by the electron beam, element-specific X-rays are emitted and can be quantified by the spectrometer. SEM/EDS can thus determine the Ca to P molar ratio of a surface, which is ∼1.67 in the case of HAp.132
X-ray diffraction (XRD).
Another tool used to confirm HAp mineralization on scaffolds is XRD based on the characteristic diffraction signature.133–136 Briefly, incident X-rays irradiate the surface and the intensities and scattering angles of the emitted X-rays are measured.137 XRD can determine HAp phase composition, degree of crystallinity, and crystallite size.134,138,139
Vibrational spectroscopy.
Attenuated total reflectance-Fourier transform infrared (ATR-FTIR) spectroscopy is also commonly used to evaluate HAp mineralization.140,141 Briefly, molecular functional groups are revealed by nature of their distinct IR absorption band.142 The ATR mode configuration acquires molecular vibrations through a reduced path length of the probing IR beam, allowing evaluation of the surface to a depth of a few micrometers. ATR-FTIR can be used to identify PO43− [∼560 and 600 cm−1 and ∼1000–1100 cm−1], CO32− [between ∼1460 and ∼1530 cm−1], and OH− [∼3570 cm−1] groups present in HAp.138,143 HAp formation, size, and distribution may be assessed with FTIR imaging via micro-ATR-IR.144 Raman spectroscopy has also been used to evaluate HAp deposits onto scaffolds via the inelastic scattering of light, and water produces less interference versus IR spectroscopy.145,146
Staining.
Staining techniques can be used to evaluate acellular HAp mineralization of bioactive scaffolds. These methods generally involve incubation of the mineralized specimen in an aqueous staining solution, followed by fluorescent imaging and analysis (e.g., with ImageJ software) to yield mineral intensity or mineralized area (% coverage).147,148 While advantageously rapid, most stains lack specificity to HAp mineral deposits versus calcium- and phosphate-containing deposits and so cannot be used alone to identify HAp.
Alizarin red S, [3,4-dihydroxy-9,10-dioxo-9,10-dihydroanth-racene-2-sulfonic acid; C14H7NaO7S; “AzHNa”] is a water-soluble sodium salt of Alizarin sulfonic acid that undergoes a reaction with HAp, described as follows:149
Ca10(PO4)6(OH)2 + 10AzHNa →10CaAz↓ + HPO42− + 2H2PO4− + 10Na+ + 2H2O |
The red/orange colored precipitate can be quantified, permitting the relative extent of HAp mineralization to be compared across specimens. Per Gregory et al., the precipitate can be removed via acetic acid extraction, neutralized with ammonium hydroxide, and absorbance intensity evaluated by a scanning spectrometer at 405 nm.150 Alizarin red S staining is not specific to HAp, and will likewise produce such deposits from other sources of Ca2+ ions.151
Von Kossa staining may also be used to evaluate HAp mineralization on scaffolds. Von Kossa staining utilizes a silver nitrate solution to transform calcium phosphate salts to silver phosphate salts, described as follows:152
Ca3(PO4)2 + 6AgNO3 → 3Ca(NO3)2 + ↓2Ag3PO4 |
The grey/black precipitate can be quantified to afford comparison of scaffold specimen HAp mineralization. However, this stain is not specific to phosphates of HAp, and cannot be used to provide absolute identification of HAp.153
Other dyes that can stain HAp mineral deposits on scaffolds include xylenol orange and calcein blue.154,155 Xylenol orange [3,3′-bis[N,N-bis(carboxymethyl)amino-methyl]-o-cresolsulf-onephthalein tetrasodium salt; C31H28N2Na4O13S] forms orange, fluorescent complexes with divalent metal ions (e.g., Ca2+).156 Calcein blue [4-methylumbelliferone-8-methyliminodiacetic acid] also binds to calcium to afford blue staining of the mineral.157
The OsteoImage™ mineralization assay (Lonza) is based on a fluorescent stain that is advantageously specific to HAp mineralization. Thus, OsteoImage™ has been used to stain HAp deposits formed via acellular mineralization158,159 as well as following cell culture.160–162
3.2.
In vitro (cellular) culture to assess scaffold bioactivity
The bioactivity of scaffolds may also be assessed via cell culture.163 A variety of cell sources have been implemented, namely stem cells such as bone marrow mesenchymal stems cells (BMSCs), adipose-derived MSCs (ASCs), perivascular stem cells, and induced pluripotent stem cells (iPSCs).164 Such osteoprogenitor cells undergo osteogenic differentiation into osteoblasts, osteoclasts, and osteocytes, all cells found in native bone tissue.165 Originating from the bone marrow, BMSCs have been particularly utilized in bone regeneration strategies.166 Biomolecules, such as the fibronectin-derived peptide sequence Arg–Gly–Asp–Ser (RGDS), are often incorporated into the scaffold to direct or support desired stem cell adhesion, spreading, and differentiation.91 Exogenous (external) growth factors, particularly BMP-2, have also been incorporated into scaffolds to accelerate osteogenesis, although these strategies often risk off-target effects in vivo.32 A wide range of methods have been used for biomolecule incorporation, as a single method of biomolecule incorporation is not necessarily universally effective for all scaffold types. For instance, phase separation within the scaffold can alter incorporated biomolecule surface presentation.108 Fluorescent imaging can be used to confirm the quality of cellular adhesion and spreading, whereby fixed cells are stained with phalloidin (cellular actin cytoskeleton) and DAPI [4′,6-diamidino-2-phenylindole] (cell nuclei).167 Prior to the aforementioned analyses, the cytocompatibility of the scaffold should be confirmed using a variety of assays (e.g., MTT assay,168 or lactate dehydrogenase [LDH] assay169).
Scaffolds that demonstrate cytocompatibility and the capacity to support cell adhesion and spreading, are often then evaluated for their capacity to support the osteogenic differentiation of adherent stem cells. In these studies, osteogenic medium – typically prepared by supplementing a conventional medium with some combination of L-ascorbic acid, β-glycerophosphate, and dexamethasone – may be utilized to mimic the osteogenic milieu present in bone.170,171 During osteogenesis, stem cells cultured on bioactive scaffolds will produce HAp deposits, the extent to which can be evaluated using histological methods with some of the previously noted stains (e.g., Alizarin red S and von Kossa150,172). The expression of the numerous osteogenic markers involved in osteogenesis,171 and methods for assessment have been recently reviewed by Le et al.173 Briefly, following a defined period of culture, the scaffold homogenates may be subjected to a variety of analyses to detect the expression of mRNA levels of genes (via polymerase chain reaction [PCR]) or expression of proteins (via immunofluorescence staining, western blot, and ELISA assays). Multiple osteogenic markers are typically evaluated as each gives insight into specific aspects of osteogenesis, including stages of progression. These include transcription factors (e.g., RUNX2, Osterix, Msx2), extracellular matrix proteins174 (e.g. secreted protein acidic and rich cysteine [SPARC], osteopontin [OPN], osteocalcin, collagen 1 α1 chain [COL1A1]), and secreted growth factors175 (e.g., vascular endothelial growth factor [VEGF],176 BMP-2,177 and BMP-4178). ALP, an early marker of osteoblast differentiation, is often quantified as an indicator of scaffold bioactivity.179,180 Expression of “off-target” markers can also be assessed to delineate the specificity of scaffold bioactivity for osteogenesis. Off-target evaluation often includes assessment of markers for chondrogenic (e.g., SRY-box transcription factor 9 [SOX9] and collagen 2 α1 chain [COL2A1]) and adipogenic (e.g., CCAAT/enhancer binding protein [α-C/EBP-α], and adipocyte fatty acid binding protein [AFABP]) differentiation.
3.3.
In vivo and ex vivo methods to assess scaffold bioactivity
A number of in vivo and ex vivo models of bone repair are available for the assessment of bioactive scaffolds.181 Animal species that have been utilized include rodent, rabbit, dog, sheep, goat, and pig, with each presenting unique advantages and disadvantages.182 Bone defects are frequently created in calvariae [as confined defects], or in ulnae, tibiae, and femurs [as segmental defects]. The minimum size for a critical defect, wherein spontaneous healing does not occur over a long duration, must be considered.183,184 However, non-critically sized calvarial defects, permitting two rather than one defect per animal, have been used to assess scaffold osseointegration and neotissue infiltration at the perimeter.39 This approach exemplifies a potential way to commit to the 3Rs (reduce, replace, refine) principle of humane animal research.185 Recently, models have also been created to assess osteoporotic defect healing,186,187 and attention has been given to sex-based differences in bone defect healing.188 Several methods to detect in vivo mineralization of scaffold treated defects have been commonly employed as highlighted below. Tissue preservation is required for most ex vivo analyses, and includes methods such as slow freezing, vitrification, hypothermic preservation, and cryopreservation.189
In vivo analyses.
Numerous methods exist for non-invasive, longitudinal monitoring of scaffold-induced bone regeneration, including bone mineral density (BMD).190,191 Micro-computed tomography (micro-CT) is perhaps the most widely utilized, given its relative low cost and efficacy.192,193 Having a spatial resolution of 50–1 μm,194 micro-CT affords 3D evaluation of bone ingrowth and volumetric changes. Micro-CT can also be used to determine BMD (mg HAp cm−3).195 Positron emission tomography (PET) can be used to evaluate longitudinal bone formation using γ-ray emitting tracers, such as a sodium fluoride ([18F]–NaF) that forms fluoroapatite with HAp of new bone tissue.196 Bone regeneration can also be monitored by single-photon emission computed tomography (SPECT) employing positron-emitting tracers, such as 99mtechnetium [99mTc-labelled diphosphonates] that are absorbed by HAp.197 Dual-modality, integrated micro-CT/PET198,199 and micro-CT/SPECT199 images have been used to assess scaffold-induced bone regeneration. Dual-energy X-ray absorptiometry (DEXA) may also be used to assess BMD.200–202 Other methods of non-invasive monitoring have been employed to avoid potential tissue damage associated with X-rays. Magnetic resonance imaging (MRI) based on semi-quantitative methods may be employed to overcome low sensitivity to bone.203 For instance, Ribot et al. developed a 3D anatomic and perfusion MRI protocol to observe scaffold-induced healing of femoral defects in rats, including mineralization and neovascularization.204 Ultrasound imaging, while limited by depth of penetration, may be used to quantify bone regeneration.205 Optical fluorescence imaging (e.g., IVIS) can be performed on fluorescently-labelled scaffolds to monitor resorption in vivo.201
Ex vivo analyses.
Endpoint tissue specimens harvested from experimental models, as well as tissue culture specimens, are frequently evaluated with the aforementioned in vivo methods. Micro-CT is widely used to view morphological features and HAp mineral deposits, wherein longer scan times are permitted for improved spatial resolution.193 Environmental SEM is also useful as it retains the natural state of the specimen by excluding the need for high vacuum conditions, as well as specimens that are clean, dry, and electrically conductive.206 Other examples demonstrate the use of ex vivo MRI,207 DEXA,208 and ultrasound209 in the evaluation of endpoint tissue specimens. Raman spectroscopy is also useful to evaluate the chemical properties of regenerated bone tissue at the nanoscale, including the degree of mineralization.210,211
Histological and histomorphometric analyses.
Histological and histomorphometric analyses of regenerated, mineralized bone tissue is crucial in assessing the bioactivity of scaffolds. Bone histomorphometry provides quantitative evaluation through the use of digitized histological images,212,213 using various image analysis platforms.214,215 Typically, harvested specimens are sequentially fixed, decalcified, dehydrated, embedded (e.g., in paraffin or PMMA), and sectioned. A variety of stains are available for these analyses, including some mentioned previously to detect acellular mineralization of scaffolds. Hematoxylin & eosin (H&E) staining – which stains cell nuclei a dark blue/purple color and basic proteins in the ECM a pink/orange color216,217 – is useful to identify woven bone, an early stage of bone development characterized by random collagen matrix deposition.39,218 Masson's trichrome staining employs acid–base chemistry by using 3 dyes to selectively stain tissue components.219 When staining for bone regeneration, new bone, collagen, and osteoids are stained blue, while mature bone is stained red.220 Von Kossa staining can also be used to differentiate mineralized [stained black] versus unmineralized [stained red] bone matrix produced by bioactive scaffolds.213
Mechanical testing.
A variety of biomechanical tests are utilized to evaluate the efficacy of scaffolds to promote bone regeneration in experimental models.221,222 In macroscopic assessments, harvested constructs are frequently subjected to quasi-static tests wherein stress or strain is applied in different modes (e.g., compression, tension, bending, torsion, and shear). Bulk modulus, strength, and toughness values can then be determined. In some cases, standards are applied such as ISO 604223–225 and ISO 5833.226,227 Push-out tests are also frequently employed to give insight into scaffold osteointegration with surrounding tissues, and efforts continue to be made to refine best practices.228 Microscopic biomechanical analyses are also utilized to give insight into nanoscale mechanical properties.222 Nanoindentation can be utilized to measure enhanced local hardness imparted by mineralized bone tissue.211 Atomic force microscopy (AFM), using contact or tapping modes, can be used to reveal nanoscale features (e.g., collagen fibrils and HAp crystals), while the nanoindentation mode is useful for nanomechanical modulus mapping.229–231 Sub-resonance AFM (e.g., PeakForce Tapping mode) has also been developed for nanomechanical mapping232 and was used by Zhou et al. to evaluate bone tissue submerged in an aqueous environment.233 It was also shown that micro-CT has also been coupled with mechanical testing to measure contact area and 3D full-field strain in bone/dental implant constructs;234 such a method could likewise be highly informative to the assessment of bioactive scaffolds.
4. Conclusions
Bioactive scaffolds remain a contemporary approach to bone regenerative engineering. Emerging in recent years are an array of methods to induce bioactivity to polymeric scaffolds that utilize bioceramic fillers, coatings and surface treatments, and additives. Bioactive composite scaffolds continue to be formed with traditional bioceramic fillers (e.g., DBM and BGs), including combinations of two or more types. Newer bioceramics have also emerged (e.g., LAPONITE® and eggshell microparticles). Bioactive coatings applied to scaffolds include deposited bioceramics, bioceramics embedded in a polymer matrix, and polymer-only types. Surface treatments such as plasma treatment, PECVD, and iCVD have also been leveraged to induce bioactivity. Finally, bioactive additives have been combined with ‘bioinert’ polymers to form bioactive scaffolds. Such additives include primarily certain natural polymers (e.g., chitosan and collagen), as well as silicon- and phosphonated/silicon-based synthetic polymers. Overall, these methods yield bioactivity throughout the scaffold (via fillers and additives) or at the surface of the scaffold (via coatings and surface treatments). For the former types, bulk properties (e.g., stiffness and degradation) are impacted which may or may not be desirable. With surface modification, bulk properties are retained, but bioactivity can be expected to be diminished when the surface is lost. High throughput screening and machine learning will be critical for efficient and successful future development of bioactive scaffolds, but necessitates standardized characterization methods. AI integrated CAD also has potential to play a pivotal role in the treatment of patient-specific complex bone defects. A plethora of in vitro (acellular and cellular), in vivo, and ex vivo methods exist to evaluate scaffold mineralization and other aspects of bone regeneration. Perhaps the most common initial assessment of scaffold bioactivity is the capacity to undergo HAp mineralization when exposed to SBF. HAp can be subsequently assessed with numerous methods (e.g., imaging, spectroscopy, and staining). Mineralization by cultured cells, particularly MSCs, as well as evaluation of other markers of osteogenesis is also commonly used to assess scaffold bioactivity. Such analyses typically rely on staining techniques and other assays. In vivo models of bone repair afford an opportunity to assess scaffold bioactivity in a physiological environment. Several assessment methods (e.g., PET, SPECT, and MRI) afford longitudinal monitoring of mineralization and tissue regeneration, with micro-CT being the most frequently employed. Harvested endpoint tissue specimens typically undergo histological and histomorphometric analyses, and biomechanical assessments are also valuable. While recent studies highlight the breadth of methods to prepare and assess bioactive scaffolds, several primary challenges remain for bioactive scaffold-based approaches to displace the clinical use of standard biological and alloplastic grafting. Overall, comparison of scaffold bioactivity in the literature is difficult. Studies employ different analyses (e.g., methods, test conditions, and selected time points) to assess bioactivity both in vitro and in vivo. Thus, standardized methods would be extremely useful to the field. Controls that could be uniformly included along with experimental scaffolds would also be beneficial to studies, but are currently lacking.235 Explant cultures (a.k.a. organ or ex vivo cultures), wherein explanted tissue-scaffold constructs are maintained in vitro and often with applied mechanical loading, may provide a valuable intermediate step between in vitro cell culture and in vivo experimental models.236 Despite these challenges, bioactive scaffolds hold tremendous promise in the treatment of bone defects.
Author contributions
Brandon Nitschke: writing – conceptualization, visualization, writing – original draft, writing – review & editing; Felipe Beltran: conceptualization, writing – original draft, writing – review & editing; Mariah Hahn: writing – review & editing; Melissa Grunlan: writing – original draft, writing – review & editing.
Conflicts of interest
There are no conflicts to declare.
Acknowledgements
Funding from NIH/NIDCR (1R01DE025886-01A1) is gratefully acknowledged.
References
- R. Florencio-Silva, G. R. Sasso, E. Sasso-Cerri, M. J. Simões and P. S. Cerri, Biomed. Res. Int., 2015, 2015, 421746 CrossRef.
- X. Lin, S. Patil, Y.-G. Gao and A. Qian, Front. Pharmacol., 2020, 11, 757 CrossRef CAS PubMed.
- E. Roddy, M. R. DeBaun, A. Daoud-Gray, Y. P. Yang and M. J. Gardner, Eur. J. Orthop. Surg. Traumatol., 2018, 28, 351–362 CrossRef PubMed.
- W. Wang and K. W. K. Yeung, Bioactive Mater., 2017, 2, 224–247 CrossRef PubMed.
- R. Amid, A. Kheiri, L. Kheiri, M. Kadkhodazadeh and M. Ekhlasmandkermani, Int. Union Biochem. Mol. Biol., 2020, 68, 1432–1452 Search PubMed.
- H. J. Haugen, S. P. Lyngstadaas, F. Rossi and G. Perale, J. Clin. Periodontol., 2019, 46(Suppl. 21), 92–102 CrossRef PubMed.
- H. Zhang, L. Yang, X.-G. Yang, F. Wang, J.-T. Feng, K.-C. Hua, Q. Li and Y.-C. Hu, Orthopaedic Surg., 2019, 11, 725–737 CrossRef PubMed.
- J. R. Jones, D. S. Brauer, L. Hupa and D. C. Greenspan, Int. J. Appl. Glass Sci., 2016, 7, 423–442 CrossRef CAS.
- J. R. Jones, Acta Biomater., 2013, 9, 4457–4486 CrossRef CAS.
- Z. Bal, T. Kaito, F. Korkusuz and H. Yoshikawa, Emergent Mater., 2020, 3, 521–544 CrossRef CAS.
- N. Ramesh, S. C. Moratti and G. J. Dias, J. Biomed. Mater. Res., Part B, 2018, 106, 2046–2057 CrossRef CAS PubMed.
- V. S. Kattimani, S. Kondaka and K. P. Lingamaneni, Bone Tissue Regener. Insights, 2016, 7, 36138 CrossRef.
- H. Lu, Y. Zhou, Y. Ma, L. Xiao, W. Ji, Y. Zhang and X. Wang, Front. Mater., 2021, 15, 698915 CrossRef.
- M. Bohner, B. L. G. Santoni and N. N. Döbelin, Acta Biomater., 2020, 113, 23–41 CrossRef CAS PubMed.
- A. W. Barone, S. Andreana and R. Dziak, Med. Res. Arch., 2020, 8 DOI:10.18103/mra.v8i11.2283.
- D. Liu, C. Cui, W. Chen, J. Shi, B. Li and S. Chen, J. Funct. Biomater., 2023, 14, 134 CrossRef CAS.
- A.-M. Yousefi, J. Appl. Biomater. Funct. Mater., 2019, 17, 2280800019872594 Search PubMed.
- K. A. Cole, G. A. Funk, M. N. Rahaman and T. E. McIff, J. Biomed. Mater. Res., Part B, 2020, 108, 1580–1591 CrossRef CAS PubMed.
- M. R. Senra and M. d F. V. Marques, J. Compos. Sci., 2020, 4, 191 CrossRef CAS.
- M. Alizadeh-Osgouei, Y. Li and C. Wen, Bioactive Mater., 2019, 4, 23–26 Search PubMed.
- L. Guo, Z. Lian, L. Yang, W. Du, T. Yu, H. Tang, C. Li and H. Qiu, J. Controlled Release, 2021, 338, 571–582 CrossRef CAS.
- M. Filippi, G. Born, M. Chaaban and A. Scherberich, Front. Bioeng. Biotechnol., 2020, 8, 474 CrossRef PubMed.
- C. Sun, J. Kang, C. Yang, J. Zheng, Y. Su, E. Dong, Y. Liu, S. Yao, C. Shi and H. Pang, Biomater. Transl., 2022, 3, 116 Search PubMed.
- B. I. Oladapo, S. A. Zahedi, S. O. Ismail and F. T. Omigbodum, Colloids Surf., B, 2021, 203, 111726 CrossRef CAS PubMed.
- H. Wang, P. Chen, Z. Shu, A. Chen, J. Su, H. Wu, Z. Chen, L. Yang, C. Yan and Y. Shi, Compos. Sci. Technol., 2023, 231, 109805 CrossRef CAS.
- A. S. Mao and D. J. Mooney, Proc. Natl. Acad. Sci. U. S. A., 2015, 112, 14452–14459 CrossRef CAS PubMed.
- G. L. Koons, M. Diba and A. G. Mikos, Nat. Rev. Mater., 2020, 5, 584–603 CrossRef CAS.
- K. S. Ogueri, T. Jafari, J. L. E. Ivirico and C. T. Laurencin, Regener. Eng. Transl. Med., 2019, 5, 128–154 CrossRef CAS PubMed.
- T. Albrektsson and C. Johansson, Eur. Spine J., 2001, 10(Suppl. 2), S96–S101 Search PubMed.
- Y. Du, J. L. Guo, J. Wang, A. G. Mikos and S. Zhang, Biomaterials, 2019, 218, 119334 CrossRef CAS PubMed.
- X. Yu, X. Tang, S. V. Gohil and C. T. Laurencin, Adv. Healthcare Mater., 2015, 4, 1268–1285 CrossRef CAS.
- D. Halloran, H. W. Durbano and A. Nohe, J. Dev. Biol., 2020, 8, 19 CrossRef CAS PubMed.
- E. Alsberg, E. E. Hill and D. J. Mooney, Crit. Rev. Oral Biol. Med., 2001, 12, 64–75 CrossRef CAS PubMed.
- T. Blokhuis and J. C. Arts, Injury, 2011, 42, S26–S29 CrossRef PubMed.
- M. N. Collins, G. Ren, K. Young, S. Pina, R. L. Reis and J. M. Oliveira, Adv. Funct. Mater., 2021, 31, 2010609 CrossRef CAS.
- B. Chang, N. Ahuja, C. Ma and X. Liu, Mater. Sci. Eng., R, 2017, 111, 1–26 CrossRef PubMed.
- M. Mirkhalaf, Y. Men, R. Wang, Y. No and H. Zreiqat, Acta Biomater., 2023, 156, 110–124 CrossRef.
- M. Ly, S. Spinelli, S. Hays and D. Zhu, Eng. Regen., 2022, 3, 41–52 Search PubMed.
- M. R. Pfau, F. O. Beltran, L. N. Woodard, L. K. Dobson, S. B. Gasson, A. B. Robbins, Z. T. Lawson, W. B. Saunders, M. R. Moreno and M. A. Grunlan, Acta Biomater., 2021, 136, 233–242 CrossRef CAS.
- D. M. Yunos, O. Bretcan and A. R. Boccaccini, J. Mater. Sci., 2008, 43, 4433–4442 CrossRef.
- F. Baino, G. Novajra and C. Vitale-Brovarone, Front. Bioeng. Biotechnol., 2015, 3, 202 Search PubMed.
- G. Turnbull, J. Clarke, F. Picard, P. Riches, L. Jia, F. Han, B. Li and W. Shu, Bioactive Mater., 2018, 3, 278–314 CrossRef PubMed.
- L. L. Hench, N. Roki and M. B. Fenn, J. Mol. Struct., 2014, 1073, 24–30 CrossRef CAS.
- T. Kokubo and H. Taakadama, Biomaterials, 2006, 27, 2907–2915 CrossRef CAS PubMed.
- H.-M. Kim, T. Himeno, T. Kokubo and T. Nakamura, Biomaterials, 2005, 26, 4366–4373 CrossRef CAS PubMed.
- Q. Zhang, J. Zhou, P. Zhi, L. Liu, C. Liu, A. Fang and Q. Zhang, Med. Nov. Technol., 2023, 17, 10025 Search PubMed.
- C. Shuai, W. Yang, P. Feng, S. Peng and H. Pan, Bioactive Mater., 2021, 6, 490–502 CrossRef CAS PubMed.
- S. Xu, J. Liu, L. Zhang, F. Yang, P. Tang and D. Wu, J. Mater. Chem. B, 2017, 5, 6110–6118 RSC.
- W.-X. Cheng, Y.-Z. Liu, X.-B. Meng, Z.-T. Zheng, L.-L. Li, L.-Q. Ke, L. Ling, C.-S. Huang, G.-Y. Zhu, H.-D. Pan, L. Qing, X.-L. Wang and P. Zhang, J. Orthop. Translat., 2021, 31, 41–51 CrossRef PubMed.
- E. Nyberg, A. Rindone, A. Dorafshar and W. L. Grayson, Tissue Eng., Part A, 2017, 23, 503–514 CrossRef CAS PubMed.
- C. Shuai, L. Yu, P. Feng, S. Peng, H. Pan and X. Bai, J. Mater. Chem. B, 2022, 10, 214–223 RSC.
- S. Sultan, N. Thomas, M. Varghese, Y. Dalvi, S. Joy, S. Hall and A. P. Mathew, Molecules, 2022, 27, 7214 CrossRef CAS PubMed.
-
B. M. Nitschke, A. E. Konz, E. A. Butchko, M. N. Wahby and M. A. Grunlan, 2023 MRS Fall Meeting, 2023.
- T. Distler, N. Fournier, A. Grünewald, C. Polley, H. Seitz, R. Detsch and A. R. Boccaccini, Front. Bioeng. Biotechnol., 2020, 8, 552 CrossRef PubMed.
- M. H. Monfared, F. E. Ranjbar, M. Torbati, S. A. Poursamar, N. Lotfibakhshaiesh, J. Ai, S. Ebrahimi-Barough and M. Azami, J. Non-Cryst. Solids, 2022, 593, 121769 CrossRef CAS.
- J. Han, J. Wu, X. Xiang, L. Xie, R. Chen, L. Li, K. Ma, Q. Sun, R. Yang and T. Huang, Mater. Des., 2023, 225, 111543 CrossRef CAS.
- F. Westhauser, F. Hohenbild, M. Arango-Ospina, S. I. Schmitz, S. Wilkesmann, L. Hupa, A. Moghaddam and A. R. Boccaccini, Int. J. Mol. Sci., 2020, 21, 1639 CrossRef CAS PubMed.
- X. Du, D. Wei, L. Huang, M. Zhu, Y. Zhang and Y. Zhu, Mater. Sci. Eng., C, 2019, 103, 109731 CrossRef CAS PubMed.
- X. Qi, P. Pei, M. Zhu, X. Du, C. Xin, S. Zhao, X. Li and Y. Zhu, Sci. Rep., 2017, 7, 42556 CrossRef CAS PubMed.
- J. K. Carrow, A. Di Luca, A. Dolatshahi-Pirouz, L. Moroni and A. K. Gaharwar, Regener. Biomater., 2019, 6, 29–37 CrossRef CAS PubMed.
- X. Wu, O. Gauntlett, T. Zhang, S. Suvarnapathaki, C. McCarthy, B. Wu and G. Camci-Unal, ACS Appl. Mater. Interfaces, 2021, 13, 60921–60932 CrossRef CAS PubMed.
- B. Huang, C. Vyas, J. J. Byun, M. El-Newehy, Z. Huang and P. Bártolo, Mater. Sci. Eng., C, 2020, 108, 110374 CrossRef CAS PubMed.
- D. Zhang, O. J. George, K. M. Petersen, A. C. Jimenez-Vergara, M. S. Hahn and M. A. Grunlan, Acta Biomater., 2014, 10, 4597–4605 CrossRef CAS PubMed.
- L. N. Woodard, K. T. Kmetz, A. A. Roth, V. M. Page and M. A. Grunlan, Biomacromolecules, 2017, 18, 4075–4083 CrossRef CAS PubMed.
- D. A. Oluwatosin, K. El Mabrouk and M. Bricha, J. Mater. Chem. B, 2023, 11, 955–973 RSC.
- J. Hatton, G. R. Davis, A.-H. I. Mourad, N. Cherupurakal, R. G. Hill and S. Mohsin, J. Funct. Biomater., 2019, 10, 15 CrossRef CAS PubMed.
- M. Schumacher, P. Habibovic and S. van Rijt, Bioact. Mater., 2020, 6, 1921–1931 Search PubMed.
- M. V. Thomas and D. A. Puleo, J. Biomed. Mater. Res., Part B, 2008, 88B, 597–610 CrossRef PubMed.
- Y. Zhao, Z. Zhang, Z. Pan and Y. Liu, Exploration, 2021, 1, 20210089 CrossRef PubMed.
- M. Du, J. Chen, K. Liu, H. Xing and C. Song, Composites, Part B, 2021, 215, 108790 CrossRef CAS.
- A. K. Gaharwar, S. M. Mihaila, A. Swami, A. Patel, S. Sant, R. L. Reis, A. P. Marques, M. E. Gomes and A. Khademhosseini, Adv. Mater., 2013, 25, 3329–3336 CrossRef CAS PubMed.
- J. K. Carrow, L. M. Cross, R. W. Reese, M. K. Jaiswal, C. A. Gregory, R. Kaunas, I. Singh and A. K. Gaharwar, Proc. Natl. Acad. Sci. U. S. A., 2018, 115, E3905–E3913 CrossRef CAS PubMed.
- T. L. Shi, Y. F. Zhang, M. X. Yao, C. Li, H. C. Wang, C. Ren, J. S. Bai, X. Cui and W. Chen, Biomater. Transl., 2023, 4, 131 Search PubMed.
- T. Zheng, Y. Huang, X. Zhang, Q. Cai, X. Deng and X. Yang, J. Mater. Chem. B, 2020, 8, 10221–10256 RSC.
- K. A. Kravanja and M. Finšgar, Mater. Des., 2022, 217, 110653 CrossRef CAS.
- N. R. Richbourg, N. A. Peppas and V. I. Sikavitsas, J. Tissue Eng. Regener. Med., 2019, 13, 1275–1293 CrossRef CAS PubMed.
- H. F. Pereira, I. F. Cengiz, F. S. Silva, R. L. Reis and J. M. Oliveira, J. Mater. Sci.: Mater. Med., 2020, 31, 27 CrossRef CAS PubMed.
- N. Fazeli, E. Arefian, S. Irani, A. Ardeshirylajimi and E. Seyedjafari, Sci. Rep., 2023, 13, 12145 CrossRef CAS PubMed.
- Y. Zhang, J.-I. Jo, L. Chen, S. Hontsu and Y. Hashimoto, Int. J. Mol. Sci., 2022, 23, 9048 CrossRef CAS PubMed.
- T.-T. Li, L. Ling, M.-C. Lin, Q. Jiang, Q. Lin, J.-H. Lin and C.-W. Lou, Nanomaterials, 2019, 9, 679 CrossRef CAS PubMed.
- S. K. L. Levengood and M. Zhang, J. Mater. Chem. B, 2014, 2, 3161–3184 RSC.
- M. Shaltooki, G. Dini and M. Mehdikhani, Mater. Sci. Eng., C, 2019, 105, 110138 CrossRef CAS PubMed.
- L. Fan, Y. Ren, S. Emmert, I. Vuckovic, S. Stojanovic, S. Najman, R. Schnettler, M. Barbeck, K. Schenke-Layland and X. Xiong, Int. J. Mol. Sci., 2023, 24, 3744 CrossRef CAS PubMed.
- F. Tabatabaei, A. Gelin, M. Rasoulianboroujeni and L. Tayebi, Colloids Surf., B, 2022, 217, 112670 CrossRef CAS PubMed.
- B. Yilmaz, A. E. Pazarceviren, A. Tezcaner and Z. Evis, Microchem. J., 2020, 155, 104713 CrossRef CAS.
- H. Lee, J. Rho and P. B. Messersmith, Adv. Mater., 2009, 21, 431–434 CrossRef CAS PubMed.
- H. Lee, S. M. Dellatore, W. M. Miller and P. B. Messersmith, Science, 2007, 318, 426–430 CrossRef CAS PubMed.
- Y. Liu, K. Ai and L. Lu, Chem. Rev., 2014, 114, 5057–5115 CrossRef CAS PubMed.
- H. Tolabi, N. Bakhtiary, S. Sayadi, M. Tamaddon, F. Ghorbani, A. R. Boccaccini and C. Liu, Front. Bioeng. Biotechnol., 2022, 10, 1008360 CrossRef PubMed.
- A. S. Arabiyat, M. R. Pfau, M. A. Grunlan and M. S. Hahn, J. Biomed. Mater. Res., Part A, 2021, 109, 2334–2345 CrossRef CAS PubMed.
- R. Teimouri, K. Abnous, S. M. Taghdisi, M. Ramezani and M. Alibolandi, J. Mater. Res. Technol., 2023, 24, 7938–7973 CrossRef CAS.
- T. Jacobs, R. Morent, N. D. Geyter, P. Dubruel and C. Leys, Plasma Chem. Plasma Process., 2012, 32, 1039–1073 CrossRef CAS.
- A. Morelli and M. J. Hawker, ACS Biomater. Sci. Eng., 2023, 9, 3760–3777 CrossRef CAS PubMed.
- H. Turkoglu Sasmazel, M. Alazzawi and N. Kadim Abid Alsahib, Molecules, 2021, 26, 1665 CrossRef PubMed.
- Y. Kim and G. Kim, Colloids Surf., B, 2014, 125, 181–189 CrossRef PubMed.
- S. Murab, S. M. S. Gruber, C.-Y. J. Lin and P. Whitlock, Mater. Sci. Eng., C, 2020, 109, 110529 CrossRef CAS PubMed.
- S. Yamada, M. A. Yassin, T. Weigel, T. Schmitz, J. Hansmann and K. Mustafa, J. Biomed. Mater. Res., Part A, 2021, 109, 1560–1574 CrossRef CAS PubMed.
- A. Terriza, J. I. Vilches-Pérez, E. de la Orden, F. Yubero, J. L. Gonzalez-Caballero, A. R. González-Elipe, J. Vilches and M. Salido, BioMed Res. Int., 2014, 2014, 253590 Search PubMed.
- Q. Song, M. Zhu, Y. Shi, J. Smay and Y. Mao, ACS Appl. Bio Mater., 2023, 6, 891–898 CrossRef CAS PubMed.
- M. V. Jose, V. Thomas, D. R. Dean and E. Nyairo, Polymer, 2009, 50, 3778–3785 CrossRef CAS.
- Z. Wang, H. Wang, J. Xiong, J. Li, X. Miao, X. Lan, X. liu, W. Wang, N. Cai and Y. Tang, Mater. Sci. Eng., C, 2021, 128, 112287 CrossRef CAS PubMed.
- N. Amiryaghoubi, N. N. Pesyan, M. Fathi and Y. Omidi, Colloids Surf., A, 2022, 634, 127895 CrossRef CAS.
- J. Ren, N. Kohli, V. Sharma, T. Shakouri, Z. Keskin-Erdogan, S. Saifzadeh, G. I. Brierly, J. C. Knowles, M. A. Woodruff and E. García-Gareta, Polymers, 2021, 13, 3999 CrossRef PubMed.
- H. Y. Jang, J. Y. Shin, S. H. Oh, J.-H. Byun and J. H. Lee, ACS Biomater. Sci. Eng., 2020, 6, 5172–5180 CrossRef CAS PubMed.
- M. T. Frassica, S. K. Jones, P. Diaz-Rodriguez, M. S. Hahn and M. A. Grunlan, Acta Biomater., 2019, 99, 100–109 CrossRef CAS PubMed.
- M. T. Frassica, S. K. Jones, J. Suriboot, A. S. Arabiyat, E. M. Ramirez, R. A. Culibrk, M. S. Hahn and M. A. Grunlan, Biomacromolecules, 2020, 21, 5189–5199 CrossRef CAS PubMed.
- F. O. Beltran, C. J. Houk and M. A. Grunlan, ACS Biomater. Sci. Eng., 2021, 7, 1631–1639 CrossRef CAS PubMed.
- F. O. Beltran, A. S. Arabiyat, R. A. Culibrk, D. J. Yeisley, C. J. Houk, A. J. Hicks, J. Negrón Hernández, B. M. Nitschke, M. S. Hahn and M. A. Grunlan, Polymer, 2023, 284, 126291 CrossRef CAS.
- R. Morent, N. D. Geyter, T. Desmet, P. Dubruel and C. Leys, Plasma Processes Polym., 2011, 8, 171–190 CrossRef CAS.
- A. Khlyustova, Y. Cheng and R. Yang, J. Mater. Chem. B, 2020, 8, 6588–6609 RSC.
- D. T.-J. Barone, J.-M. Raquez and P. Dubois, Polym. Adv. Technol., 2011, 22, 463–475 CrossRef CAS.
- G. A. Rico-Llanos, S. Borrego-González, M. Moncayo-Donoso, J. Becerra and R. Visser, Polymers, 2021, 17, 599 CrossRef PubMed.
- E. Roldán, N. D. Reeves, G. Cooper and K. Andrews, Front. Bioeng. Biotechnol., 2023, 26, 100452 Search PubMed.
- J. Sun and H. Tan, Materials, 2013, 6, 1285–1309 CrossRef CAS PubMed.
- P. Zhai, X. Peng, B. Li, Y. Liu, H. Sun and X. Li, Int. J. Biol. Macrmol., 2020, 151, 1224–1239 CrossRef CAS PubMed.
- A. Guzmán-Soria, V. Moreno-Serna, D. A. Canales, C. García-Herrera, P. A. Zapata and P. A. Orihuela, Polymers, 2023, 15, 1079 CrossRef PubMed.
- X. Xue, Y. Han, Y. Deng and J. Su, Adv. Funct. Mater., 2021, 31, 2009432 CrossRef CAS.
- L. Yang, S. Pijuan-Galito, H. S. Rho, A. S. Vasilevich, A. D. Eren, L. Ge, P. Habibovic, M. R. Alexander, J. de Boer and A. Carlier, Chem. Rev., 2021, 121, 4561–4677 CrossRef CAS PubMed.
- A. K. Patel, M. W. Tibbitt, A. D. Celiz, M. C. Davies, R. Langer, C. Denning, M. R. Alexander and D. G. Anderson, Curr. Opin. Solid State Mater. Sci., 2016, 20, 202–211 CrossRef CAS.
- M. M. H. Shandhi and J. P. Dunn, Cell Rep. Med., 2022, 3, 100861 CrossRef PubMed.
- J. L. Guo, M. Januszyk and M. T. Longaker, Tissue Eng., Part A, 2023, 29, 2–19 CrossRef PubMed.
- S. M. McDonald, E. K. Augustine, Q. Lanners, C. Rudin, L. C. Brinson and M. L. Becker, Nat. Commun., 2023, 14, 4838 CrossRef CAS PubMed.
- A. Logeshwaran, R. Elsen and S. Nayak, ACS Biomater. Sci. Eng., 2024, 10, 677–696 CrossRef PubMed.
- ISO 23317:2014 – Implants for Surgery – In Vitro Evaluation for Apatite-Forming
Ability of Implant Materials, 2014.
- F. Baino and S. Yamaguchi, Biomimetics, 2020, 5, 57 CrossRef CAS PubMed.
- T. Kokubo, Biomaterials, 1991, 12, 155–163 CrossRef CAS PubMed.
- P. X. Zhu, Y. Masuda and K. Koumoto, J. Colloid Interface Sci., 2001, 243, 31–36 CrossRef CAS.
- F. Barrere, C. A. van Blitterswijk, K. de Groot and P. Layrolle, Biomaterials, 2002, 23, 1921–1930 CrossRef CAS PubMed.
- J. T. Y. Lee, Y. Leng, K. L. Chow, F. ren, X. Ge, K. Wang and X. Lu, Acta Biomater., 2011, 7, 2615–2622 CrossRef CAS PubMed.
- M. P. Gashti, F. Alimohammadi, J. Hulliger, M. Burgener, H. Oulevey-Aboulfad and G. L. Bowlin, Curr. Microsc. Contrib. Adv. Sci. Tech., 2012, 625–638 Search PubMed.
-
J. Goldstein, D. Newbury, D. Joy, C. Lyman, P. Echlin, E. Lifshin, L. Sawyer and M. Michael, Scanning Electron Microscopy and X-Ray Microanalysis, Springer, 3rd edn, 2007, ch. 1, pp. 1–20 Search PubMed.
- J. Jeong, J. H. Kim, J. H. Shim, N. S. Hwang and C. Y. Heo, Biomater. Res., 2019, 23, 4 CrossRef PubMed.
- R. Kotian, P. P. Rao and P. Madhyastha, Eur. J. Dent., 2017, 11, 438–446 CrossRef PubMed.
- J. G. M. Poralan, J. E. Gambe, E. M. Alcantara and R. M. Vequizo, IOP Conf. Series: Mater. Sci. Eng., 2015, 79, 012828 Search PubMed.
- K. Teshima, S. H. Lee, K. Yubuta, S. Mori, T. Shishido and S. Oishi, CrystEngComm, 2011, 13, 827–830 RSC.
- S. Kim, H.-S. Ryu, H. Shin, H. S. Jung and K. S. Hong, Mater. Chem. Phys., 2005, 91, 500–506 CrossRef CAS.
- H. Stanjek and W. Häusler, Hyperfine Interact., 2004, 154, 107–119 CrossRef CAS.
- M. Mir, F. L. Leiteb, J. P. S. de Paula Herrmann, F. L. Pissettia, A. M. Rossid, E. L. Moreirad and Y. P. Mascarenhase, Mater. Res., 2012, 15, 622–627 CrossRef CAS.
- M. Rabiei, A. Palevicius, A. Monshi, S. Nasiri, A. Vilkauskas and G. Janusas, Nanomaterials, 2010, 10, 1627 CrossRef PubMed.
- M. S. Hossain and S. Ahmed, RSC Adv., 2023, 13, 14625–14630 RSC.
- Y. Cao, M. L. Mei, Q.-L. Li, E. C. M. Lo and C. H. Chu, ACS Appl. Mater. Interfaces, 2014, 6, 410–420 CrossRef CAS PubMed.
-
H. Kaur, B. Rana, D. Tomar, S. Kaur and K. C. Jena, in Modern Techniques of Spectroscopy, ed. D. K. Singh, M. Pradhan and A. Materny, Springer, Singapore, 2021, pp. 3–56 Search PubMed.
- H. Gheisari, E. Karamian and M. Abdellahi, Ceram. Int., 2015, 41, 5957–5975 CrossRef.
- S. G. Kazarian, K. L. A. Chan, V. Maquet and A. R. Boccaccini, Biomaterials, 2004, 25, 3931–3938 CrossRef CAS PubMed.
- L. Borkowski, A. Sroka-Bartnicka, P. Drączkowski, A. Ptak, E. Zięba, A. Ślósarczyk and G. Ginalska, Mater. Sci. Eng., C, 2016, 62, 260–267 CrossRef CAS PubMed.
- K. J. I. Ember, M. A. Hoeve, S. L. McAughtrie, M. S. Bergholt, B. J. Dwyer, M. M. Stevens, K. Faulds, S. J. Forbes and C. J. Campbell, npj Regener. Med., 2017, 2, 12 CrossRef PubMed.
- M. D. Weir and H. H. K. Xu, J. Biomed. Mater. Res., Part B, 2010, 93, 93–105 CrossRef PubMed.
- S. A. Davari, S. Masjedi, Z. Ferdous and D. Mukherjee, J. Biophotonics, 2018, 11, e201600288 CrossRef PubMed.
- D. N. Misra, Colloids Surf., 1992, 66, 181–187 CrossRef CAS.
- C. A. Gregory, W. G. Gunn, A. Peister and D. J. Prockop, Anal. Biochem., 2004, 329, 77–84 CrossRef CAS PubMed.
- M. Lievremont, J. Potus and B. Guillou, Acta Anat., 1982, 114, 268–280 CrossRef CAS PubMed.
- M. R. Schneider, Histochem. Cell Biol., 2021, 156, 523–526 CAS.
- L. F. Bonewald, S. E. Harris, J. Rosser, M. R. Dallas, S. L. Dallas, N. P. Camacho, B. Boyan and A. Boskey, Calcif. Tissue Int., 2003, 72, 537–547 CrossRef CAS PubMed.
- M. D. Weir and H. H. Xu, J. Biomed. Mater. Res., Part B, 2010, 93, 93–105 CrossRef PubMed.
- K. White, R. Chalaby, G. Lowe, J. Berlin, C. Glackin and R. Olabisi, Polymers, 2021, 13, 2274 CrossRef CAS PubMed.
- B. A. Rahn and S. M. Perren, Stain Technol., 1971, 46, 125–129 CrossRef CAS PubMed.
- E. Tambutté, S. Tambutté, N. Segonds, D. Zoccola, A. Venn, J. Erez and D. Allemand, Proc. R. Soc. B, 2012, 279, 19–27 CrossRef PubMed.
- A. K. Nguyen, S. B. Nelson, S. A. Skoog, P. Jaipan, P. E. Petrochenko, A. Kaiser, L. Lo, J. Moreno, R. J. Narayan, P. L. Goering and G. Kumar, J. Biomed. Mater. Res., Part B, 2023, 111, 987–995 CrossRef CAS PubMed.
- Y. Kim, E. J. Lee, A. V. Davydov, S. Frukhtbeyen, J. E. Jonathan, S. Takagi, L. Chow and S. Alimperti, Biomed. Mater., 2021, 16, 045002 CrossRef CAS PubMed.
- D. Lantigua, X. Wu, S. Suvarnapathaki, M. A. Lguyen and G. Camci-Unal, Bioengineering, 2021, 8, 169 CrossRef CAS PubMed.
- Y. Dong, L. Suryani, X. Zhou, P. Muthukumaran, M. Rakshit, F. Yang, F. Wen, A. M. Hassanbhai, K. Parida, D. T. Simon, D. Iandolo, P. S. Lee, K. W. Ng and S. H. Teoh, Int. J. Mol. Sci., 2021, 22, 6438 CrossRef CAS PubMed.
- K. Vuornos, H. Huhtala, M. Kääriäinen, K. Kuismanen, L. Hupa, M. Kellomäki and S. Miettinen, J. Biomed. Mater. Res., Part B, 2019, 108, 1332–1342 CrossRef PubMed.
- B. Safari, A. Aghanejad, L. Roshangar and S. Davaran, Colloids Surf., B, 2021, 198, 111462 CrossRef CAS PubMed.
- Y.-Z. Jin and J. H. Lee, Clin. Orthop. Surg., 2018, 10, 271–278 CrossRef PubMed.
- A. J. Friedenstein, R. K. Chailakhyan and U. V. Gerasimov, Cell Tissue Kinet., 1987, 20, 263–272 CAS.
- A. Arthur and S. Gronthos, Int. J. Mol. Sci., 2020, 21, 9759 CrossRef CAS PubMed.
- M. J. Poellmann, J. B. Estrada, T. Boudou, Z. T. Berent, C. Franck and A. J. Wagoner Johnson, J. Biomech. Eng., 2015, 137, 124503 CrossRef PubMed.
- M. Ghasemi, T. Turnbull, S. Sebastian and I. Kempson, Int. J. Appl. Glass Sci., 2021, 22, 12827 CAS.
- P. Kumar, A. Nagarajan and P. D. Uchil, Cold Spring Harbor Perspect., 2018, 465–468 Search PubMed.
- M. Yuasa, T. Yamada, T. Taniyama, T. Masaoka, W. Xuetao, T. Yoshii, M. Horie, H. Yasuda, T. Uemura, A. Okawa and S. Sotome, PLoS One, 2015, 6, e0116462 CrossRef PubMed.
- J. Mollentze, C. Durandt and M. S. Pepper, Stem Cells Int., 2021, 2021, 9919361 CrossRef PubMed.
- R. Rosales-Ibáñez, A. E. Viera-Ruiz, J. V. Cauich-Rodríguez, H. J. Carrillo-Escalante, A. González-González, J. J. Rodríguez-Martínez and F. Hernández-Sánchez, Polym. Bull., 2023, 80, 2533–2552 CrossRef.
- J. Le, L. Zhongqun, W. Zhaoyan, S. Yijun, W. Yingjin, W. Yaojie, J. Yanan, J. Zhanrong, M. Chunyang and G. Fangli, Bioact. Mater., 2021, 6, 613–626 CAS.
- W. C. W. Chan, Z. Tan, M. K. T. To and D. Chan, Int. J. Mol. Sci., 2021, 22, 5545 CrossRef PubMed.
- É. R. Oliveira, L. Nie, D. Podstawczyk, A. Allahbakhsh, J. Ratnayake, D. L. Brasil and A. Shavandi, Int. J. Mol. Sci., 2021, 22, 903 CrossRef PubMed.
- K. Hu and B. R. Olsen, Bone, 2016, 91, 31–38 CrossRef PubMed.
- S.-Y. Park, K.-H. Kim, S. Kim, Y.-M. Lee and Y.-J. Seol, Pharmaceutics, 2019, 11, 393 CrossRef CAS PubMed.
- H. Cheng, X. Gao, M. Huard, A. Lu, J. J. Ruzbarsky, S. Amra, B. Wang and J. Huard, Stem Cell Res. Ther., 2022, 13, 385 CrossRef CAS PubMed.
- S. Trivedi, K. Srivastava, A. Gupta, T. S. Saluja, S. Kumar, D. Mehrotra and S. K. Singh, J. Oral Biol. Craniofac. Res., 2020, 10, 158–160 CrossRef PubMed.
- N. Rezania, M. Asadi-Eydivand, N. Abolfathi, S. Bonakdar, M. Mehrjoo and M. Solati-Hashjin, J. Mater. Sci.: Mater. Med., 2022, 33, 31 CrossRef CAS PubMed.
- I. L. Tsiklin, A. V. Shabunin, A. V. Kolsanov and L. T. Volova, Polymers, 2022, 14, 3222 CrossRef CAS.
- Y. Li, S.-K. Chen, L. Li, L. Qin, X.-L. Wang and Y.-X. Lai, J. Orthop. Translat., 2015, 3, 95–104 CrossRef.
- D. S. Sparks, S. Saifzadeh, F. M. Savi, C. E. Dlaska, A. Berner, J. Henkel, J. C. Reichert, M. Wullschleger, J. Ren, A. Cpitria, J. A. McGovern, R. Steck, M. Wagels, M. A. Woodruff, M. A. Schuetz and D. W. Hutmacher, Nat. Protoc., 2020, 15, 877–924 CrossRef CAS.
- P. P. Spicer, J. D. Kretlow, S. Young, J. A. Jansen, F. K. Kasper and A. G. Mikos, Nat. Protoc., 2012, 7, 1918–1929 CrossRef CAS.
- J. Zurlo, D. Rudacille and A. M. Goldberg, Altern. Lab Anim., 1996, 23, 298–304 Search PubMed.
- T. El Khassawna, F. Merboth, D. Malhan, W. Böcker, D. E. Daghma, S. Stoetzel, S. Kern, F. Hassan, D. Rosenbaum and J. Langenstein, Am. J. Pathol., 2017, 187, 1686–1699 CrossRef CAS PubMed.
- M. Rupp, C. Biehl, D. Malhan, F. Hassan, S. Attia, S. Rosch, A. B. Schäfer, E. McMahon, M. Kampschulte, C. Heiss and T. El Khassawna, Life, 2021, 11, 254 CrossRef CAS PubMed.
- E. Ortona, M. T. Pagano, L. Capossela and W. Malorni, Biology, 2023, 12, 993 CrossRef CAS.
- S. Freitas-Ribeiro, R. L. Reis and R. P. Pirraco, PNAS Nexus, 2022, 1, 1–15 CrossRef PubMed.
- E. A. Fragogeorgi, M. Rouchota, M. Georgiou, M. Velez, P. Bouziotis and G. Loudos, J. Tissue Eng., 2019, 10, 2041731419854586 Search PubMed.
- M. Ventura, O. C. Boerman, C. de Korte, M. Rijpkema, A. Heerschap, E. Oosterwijk, J. A. Jansen and X. F. Walboomers, Tissue Eng., 2014, 20, 578–595 CrossRef PubMed.
- J. D. Boerckel, D. E. Mason, A. M. McDermott and E. Alsberg, Stem Cell Res. Ther., 2014, 5, 144 CrossRef PubMed.
- M. P. Fernández, F. Witte and G. Tozzi, J. Microscopy, 2020, 277, 179–196 CrossRef PubMed.
- E. L. Ritman, Annu. Rev. Biomed. Eng., 2011, 13, 531–552 CrossRef CAS PubMed.
-
E. F. Morgan, A. d Giacomo and L. C. Gerstenfeld, in Skeletal Development and Repair: Methods and Protocols, Methods in Molecular Biology, ed. M. J. Hilton, Springer Nature, 2021, pp. 17–37 Search PubMed.
- S. Bastawrous, P. Bhargava, F. Behnia, D. S. W. Djang and D. R. Haseley, Nucl. Med., 2014, 34, 1295–1316 Search PubMed.
- K. Horiuchi-Suzuki, A. Konno, M. Udeda, Y. Fukuda, S. Nishio, K. Hashimoto and H. Saji, Eur. J. Nucl. Med. Mol. Imaging, 2004, 31, 388–398 CrossRef PubMed.
- S. Annibali, D. Bellavia, L. Ottolenghi, A. Cicconettie, M. P. Cristalli, R. Quaranta and A. Pilloni, J. Biomed. Mater. Res., Part B, 2014, 102, 815–825 CrossRef PubMed.
- M. Ventura, G. M. Franssen, E. Oosterwijk, O. C. Boerman, J. A. Jansen and X. F. Walboomers, J. Tissue Eng. Regener. Med., 2016, 10, 843–854 CrossRef CAS PubMed.
- H. S. Kim, E. S. Jeong, M. H. Yang and S.-O. Yang, Osteoporosis Sarcopenia, 2018, 4, 79–85 CrossRef PubMed.
- B. Gurumurthy, M. A. Tucci, L.-W. Fan, H. A. Benghuzzi, P. Pal, G. L. Bidwell, S. M. S. Moracho, Z. Cason, D. Gordy and A. V. Jonorkar, Adv. Healthcare Mater., 2020, 9, 1901385 CrossRef CAS PubMed.
- C.-C. Niu, S.-S. Lin, W.-J. Chen, S.-J. Liu, L.-H. Chen, C.-Y. Yang, C.-J. Wang, L.-J. Yuan, P.-H. Chen and H.-Y. Cheng, J. Orthop. Surg. Res., 2015, 10, 111 CrossRef PubMed.
- G. Chang, S. Boones, D. Martel, C. S. Rajapaske, R. S. Hallyburton, M. Valko, S. Honig and R. R. Regatte, J. Magn. Reson. Imaging, 2017, 46, 323–337 CrossRef PubMed.
- E. J. Ribot, C. Tournier, R. Aid-Launais, N. Koonjoo, H. Oliveira, A. J. Trotier, S. Rey, D. Wecker, D. Letourneur, J. A. Vilamitjana and S. Miraux, Sci. Rep., 2017, 7, 6100 CrossRef PubMed.
- S. Tang, P. Shajudeen, E. Tasciotti and R. Righetti, Sci. Rep., 2020, 10, 13646 CrossRef CAS PubMed.
- F. A. Shah, K. Ruscsák and A. Palmquist, Bone Res., 2019, 7, 15 CrossRef PubMed.
- N. Celikkin, S. Mastrogiacomo, W. Dou, A. Heerschap, E. Oosterwijk, X. F. Walboomers and W. Święszkowski, J. Biomed. Mater. Res., 2022, 110, 2133–2145 CrossRef CAS PubMed.
- J. J. Jolly, N. F. M. Fozi, K.-Y. Chin, S. K. Wong, K. H. Chua, E. Alias, N. S. Adnan and S. Ima-Nirwana, Exp. Ther. Med., 2021, 22, 680 CrossRef CAS PubMed.
- K. Oe, M. Miwa, K. Nagamune, Y. Sakai, S. Y. Lee, T. Niikura, T. Iwakura, T. Hasegawa, N. Shibanuma, Y. Hata, R. Kuroda and M. Kurosaka, Tissue Eng., 2010, 16, 347–353 CrossRef CAS PubMed.
- L. Borkowski, A. Sroka-Bartnicka, I. Polkowska, M. Pawlowska, K. Palka, E. Zieba, A. Slosarczyk, K. Jozwiak and G. Ginalska, Anal. Bioanal. Chem., 2017, 409, 5747–5755 CrossRef CAS PubMed.
- M. Fraulob, S. Pang, S. L. Cann, R. Vayron, M. Laurent-Brocq, S. Todatry, J. A. N. T. Soares, I. Jasiuk and G. Haïat, Med. Eng. Phys., 2020, 84, 60–67 CrossRef PubMed.
-
F. Rauch, in Osteogenesis Imperfecta: A Translational Approach to Brittle Bone Disease, ed. J. R. Shapiro, P. H. Byers, F. H. Glorieux and P. D. Sponseller, Academic Press, 2014, pp. 237–242 Search PubMed.
- D. Malhan, M. Muelke, S. Rosch, A. B. Schaefer, F. Merboth, D. Weisweiler, C. Heiss, I. Arganda-Carreras and T. E. Khassawana, Front. Endocrinol., 2018, 9, 666 CrossRef PubMed.
- F. M. Savi, F. Lawrence, D. W. Hutmacher, M. A. Woodruff, L. J. Bray and M. L. Wille, Tissue Eng., Part C, 2019, 25, 732–741 CrossRef PubMed.
- K. P. Egan, T. A. Brennan and R. J. Pignolo, Histopathology, 2012, 61, 1168–1173 CrossRef PubMed.
- A. H. Fischer, K. A. Jacobson, J. Rose and R. Zeller, Cold Spring Harbor Perspect., 2008, 2008, pdb.prot4986 CrossRef PubMed.
-
W. Bloch and Y. Korkmaz, Classical histological staining procedures in cardiovascular research, 2005 Search PubMed.
- F. Shapiro and J. Y. Wu, Eur. Cell. Mater., 2019, 38, 137–167 CrossRef CAS PubMed.
-
S. Wulff, L. Hafer, M. Cheles, D. A. Htl and D. A. Stanforth, Guide to special stains, 2004.
- C. Zhang, B. Yan, Z. Cui, S. Cui, T. Zhang, X. Wang, D. Liu, R. Yang, N. Jiang and Y. Zhou, Sci. Rep., 2017, 7, 10519 CrossRef PubMed.
- N. N. F. N. M. N. Kahar, M. Jaafar, N. Ahmad, A. R. Sulaiman, B. H. Yahaya and Z. A. A. Hamid, Mater. Today: Proc., 2022, 66, 3031–3035 Search PubMed.
- Y. Niu, T. Du and Y. Liu, J. Funct. Biomater., 2023, 14, 212 CrossRef CAS PubMed.
- C. G. Helguero, V. M. Mustahsan, S. Parmar, S. Pentyala, J. L. Pfail, I. Kao, D. E. Komatsu and S. Pentyala, J. Orthop. Surg. Res., 2017, 12, 195 CrossRef PubMed.
- ISO 604:2002 – Plastics – Determination of Compressive Properties, 2002.
- C. G. Helguero, J. L. Amaya, D. E. Komatsu, S. Pentyala, V. Mustahsan, E. A. Ramirez and I. Kao, Proceedia CIRP, 2017, 65, 121–126 CrossRef.
- ISO 5833:2022 – Implants for surgery – Acrylic resin cements, 2022.
- D. Wu, A. Spanou, A. Diez-Escudero and C. Persson, J. Mech. Behav. Biomed. Mater., 2020, 103, 103608 CrossRef CAS PubMed.
- Z. T. Lawson, J. Han, W. B. Saunders, M. A. Grunlan, M. R. Moreno and A. R. Robbins, Methods X, 2021, 8, 101541 CAS.
- P. J. Thurner, Wiley Interdiscip. Rev.: Nanomed. Nanobiotechnol., 2009, 1, 624–649 Search PubMed.
- M. Bontempi, F. Salamnna, R. Capozza, A. Visani, M. Fini and A. Gambardella, Materials, 2022, 15, 7512 CrossRef CAS PubMed.
- Y. Zhou and J. Du, Prog. Biophys. Mol. Biol., 2022, 176, 52–66 CrossRef PubMed.
-
C. Su, J. Shi, Y. Hu, S. Hu and J. Ma, US Pat., US9291640B2, 2014 Search PubMed.
- Y. Zhou, M. Kastner, T. B. Tighe and J. Du, Extreme Mech. Lett., 2020, 41, 101031 CrossRef.
- Y. Zhou, C. Gong, M. Hossaini-Zadeh and J. Du, J. Mech. Behav. Biomed. Mater., 2020, 110, 103858 CrossRef CAS PubMed.
- C. G. Simon, M. J. Yaszmeski, A. Ratcliffe, P. Tomlins, R. Luginbuehl and J. A. Tesk, J. Biomed. Mater. Res., Part B, 2015, 103, 949–959 CrossRef CAS PubMed.
- E. E. A. Cramer, K. Ito and S. Hofmann, Curr. Osteoporos. Rep., 2021, 19, 75–87 CrossRef CAS PubMed.
|
This journal is © The Royal Society of Chemistry 2024 |