DOI:
10.1039/D3SM01001E
(Paper)
Soft Matter, 2024,
20, 133-143
Enhancing the textural and rheological properties of fermentation-induced pea protein emulsion gels with transglutaminase
Received
29th July 2023
, Accepted 28th November 2023
First published on 6th December 2023
Abstract
The aim of this study was to assess how transglutaminase (TG) impacts the microstructure, texture, and rheological properties of fermentation-induced pea protein emulsion gels. Additionally, the study examined the influence of storage time on the functional properties of these gels. Fermentation-induced pea protein gels were produced in the presence or absence of TG and stored for 1, 4, 8, 12, and 16 weeks. Texture analysis, rheological measurements, moisture content and microstructure evaluation with confocal laser scanning microscopy (CLSM) and 3D image analysis were conducted to explore the effects of TG on the structural and rheological properties of the fermented samples. The porosity of the protein networks in the pea gels decreased in the presence of TG, the storage modulus increased and the textural characteristics were significantly improved, resulting in harder and more springy gels. The gel porosity increased in gels with and without TG after storage but the effect of storage on textural and rheological properties was limited, indicating limited structural rearrangement once the fermentation-induced pea protein emulsion gels are formed. Greater coalescence was observed for oil droplets within the gel matrix after 16 weeks of storage in the absence of TG, consistent with these protein structures being weaker than the more structurally stable TG-treated gels. This study shows that TG treatment is a powerful tool to enhance the textural and rheological properties of fermentation-induced pea protein emulsion gels.
1 Introduction
The gelation dynamics and physicochemical properties of protein gels depend on the protein sequence and molecular structure of the proteins involved. Dairy protein gels are characterized by a network of aggregated casein micelle particles that have been previously functionalized through enzymes, acidity and heat to facilitate casein reorganization into three-dimensional networks.1 Plant protein gels are three-dimensional networks that can be induced by heat, acid, enzymatic action, or fermentation. All four methods may transform a protein dispersion in liquid state into a deformable gel structure through the formation of covalent and non-covalent bonds between proteins. For plant proteins, a denaturation step is often required to unfold the globular protein structure, exposing hydrophobic residues, which allows the formation of protein aggregates that establish gels, stabilized mainly by hydrophobic interactions, disulfide bonds and hydrogen bonds.2,3 The textural and rheological properties of these gels can vary with final pH, ionic strength, the presence of reducing agents or protein concentration, among other factors.2 More complex plant protein gels can be formed by adding plant lipid sources to plant protein suspensions to make oil-in-water emulsion gel systems. Once gelled, the protein network traps oil droplets, along with water, within the pores of the network. These colloidal systems can exist as self-supporting gels, with an appearance typical of dairy protein gels and can serve as a base for the development of plant-based cheese.4 The gel strength of fermentation-induced plant protein systems, however, is generally weaker than dairy gels with a similar protein content.5 Plant-based cheeses currently available in the market are mainly based on solid fats and starch for texture development6 and not many are fermented. An alternative approach would be to produce fermentation-induced gels, where the main structuring agents would be plant proteins. Such products would still remain far from consumer expectations, however, as the gel hardness would be much lower than in dairy counterparts. For this reason, strategies to increase plant protein gelation dynamics and gel strength are of interest to increase our understanding and control of food soft matter. This will allow plant-based cheese products to provide the texture and functionality that consumers value in dairy cheeses, such as sliceability, stretchability, or meltability. Increasing the number of protein–protein interactions and the fraction of proteins incorporated in the gel network is one approach commonly used to increase gel strength in the dairy industry, which may also be applied to plant protein gels.7 Other structural factors, such as the size of the protein aggregates and whether the protein network strands are fine or particulated will also affect gel texture and technological functionality.7,8 In the context of protein gelation, it is important to explore various mechanisms that can induce three-dimensional networks and influence gel properties through the establishment of specific chemical and physical bonds. One intriguing avenue is fermentation, as it introduces unique mechanistic elements that can significantly impact the textural and rheological properties of protein gels.
While earlier discussions have mostly focused on the gelation dynamics of proteins induced by enzymes, acidity, heat, and other conventional methods, fermentation offers a distinct and complex pathway. In fermentation-induced gels, a protein dispersion in a liquid state transforms into a deformable gel structure. During this transformation, microorganism-derived metabolites and enzymes mediate biochemical reactions resulting in a unique texture, flavour and gel functionality. Plant proteins must expose their hydrophibic residues during an unfolding step prior to fermentation. During acidification, the pH approaches the pea protein isoelectric point (approximately pH 4.8 for legumin and pH 5.5 for vicilin9), allowing for the formation of protein aggregates that further constitute gels.10 Furthermore, bacterial protein metabolism might hydrolyse and otherwise modify proteins leading to new reactive sites for crosslinking. Understanding and controlling these mechanisms of fermentation-induced gelation is crucial to create plant-based cheeses with optimal textural properties. Furthermore, continued bacterial metabolic activity during storage of these gels can further affect their structural and physicochemical properties.
The nature and number of protein–protein interactions involved in gel formation can have a bigger impact on gel hardness than the type of protein involved in gel formation.11 Different hydrolyzed pea protein fractions, for example, have been shown to form gels with identical rheological behavior.11 Considering a plant-based cheese as a three-dimensional protein network, one potentially effective strategy to increase protein–protein interactions is to enzymatically crosslink the unfolded globular plant proteins with transglutaminase (TG). In pea protein-based systems, this enzyme creates covalent bonds between the amino groups on lysine residues and the carboxyamide groups on glutamine residues.12 These bonds can occur within a protein (intra-molecular bonds) or between different proteins (inter-molecular bonds),13 giving a variety of cross-links. TG has been successfully applied to increase curd yield in dairy cheese production, where casein that would otherwise be lost in the whey is incorporated into the cheese curd.14 The effect of TG on gel firmness is less clear and potentially system dependent, as acid-induced TG casein gels tend to be more brittle and less firm compared to rennet-induced casein gels of the same protein concentration.1 Consequently TG treatment reduced fracture stress and the storage modulus of rennet-induced dairy cheese.15 TG has been used successfully to increase gel firmness in other gel-based systems, however, including pea protein.13,16–21
Textural improvements using TG have also been reported for plant protein matrices for meat and seafood analogues,22 bread,23 heat-induced pea protein gels,13,24 fermentation-induced mung bean gels,20 soy protein cream cheese16 and cheeses made from soy/peanut-dairy blends.25 Yet these studies did not investigate fermentation-induced gels made from pea protein emulsions and have not evaluated the effect of TG in an acidic pea protein environment. Therefore, the potential of TG to increase molecular crosslinking to stabilise fermentation-induced pea protein emulsion gels is not yet known, despite this potentially being a promising route to create plant-based cheese with optimal textural properties.
The storage of plant protein gels can also affect structural and physicochemical properties. This can include physical changes arising from rearrangement of the gel network, as well as fermentation-induced biochemical processes that include lipolysis and proteolysis.26 There are only a few studies examining the effect of storage on plant protein curds, which differ in their findings. Enzyme-ripened27 and mould-fermented28 soy curds showed a decrease in hardness over time, attributed to proteolysis caused by endogenous enzymes when studied after 16 days and 90 days. In contrast, the hardness of lactic acid bacteria (LAB)-fermented soy curd was found to increase over time up to 35 days of storage.26 Proteolysis cleaves the peptide bonds to progressively form smaller proteins and eventually amino acids. In this way, the protein network can be broken, leading to a softer texture over time. Alternatively, water entrapped in the protein network may bind to ionic groups made available through proteolysis, increasing gel hardness.29 These differing results show that there is still much to learn about the development of texture over time in plant protein gel systems, which will enable the textural and rheological properties to be optimised.
Pea protein already has a large market share in the ingredient industry, mainly due to its use in meat alternatives, as well as the high yield, high nutritional value, low allergenicity, and low environmental impact compared to proteins sourced from other crops. These factors are driving an increasing interest in the use of pea protein for fermented dairy alternatives.30–32 The purpose of this study was to evaluate the effect of TG treatment on the microstructure, textural and rheological properties of fermentation-induced 10% w/w pea protein isolate emulsion gels to determine if TG could enhance gel properties. The effect of storage time on functional properties was also assessed.
2 Materials and methods
2.1 Materials
Pea protein isolate (PPI) was purchased from ADM (Chicago, IL, USA). The declared composition was: 81.3% protein, 7% fat and 9% fiber. Other matrix ingredients were sunflower oil (Woolworths, Auckland, New Zealand), sucrose, and dextrose (Sigma Aldrich, Søborg, Denmark). A lactic acid bacteria starter culture comprising Streptococcus thermophilus and Lactobacillus bulgaricus (Chr. Hansen, Hørsholm, Denmark) was used to ferment the pea protein matrix and the transglutaminase (TG) was purchased from Ajinomoto Co., Inc. (Chuo City, Tokyo, Japan).
2.2 Base preparation, particle size and protein solubility
PPI was suspended at 12% w/w in a 1% w/w glucose- and 1% w/w sucrose–water solution and blended with a triple-blade hand blender for 3 min (Kenwood Limited, Woking, United Kingdom) until dispersed. The particle size distribution was monomodal with the volume weighted mean (d 4,3) = 42 ± 2 μm. Analysis was performed using a Mastersizer 3000 (Malvern Instruments, Worcestershire, UK) with a refractive index of 1.33 and 1.462 for water and protein particles, respectively. The solubility of the PPI in solution was measured as a ratio between the protein concentration of supernatant compared to the original PPI suspension × 100, where an aliquot of PPI dispersion (40 g) was centrifuged at 3840 × g for 30 min using a JS-4.2 swing bucket rotor at room temperature (22 °C) (J6-MI, Beckman Coulter, USA). The supernatant (1.0 g) was collected and analysed together with an aliquot of the original experimental milk for nitrogen content using a nitrogen analyser (LECO, Castle Hill NSW, Australia; conversion factor of 6.38). Particle size analysis and solubility was determined in triplicate for duplicate biological replicates. The dispersion was pre-homogenized with 12% sunflower oil using the same blender to form a coarse oil-in-water emulsion and homogenized with an FT91 two stage (150 bar; 50 bar) homogenizer (Armfield, Hampshire, England) in a single pass. Homogenized emulsions were heat-treated at 90 °C for 20 min while stirring in a Bellini Supercook (New York, USA) and cooled to room temperature prior to microbial inoculation. The protein–oil ratios in the production of the matrix were adjusted so that, after inoculation of the diluted starter culture and enzyme, the final protein content would be 10% and the final oil content would be 10%.
2.3 Production and storage of fermentation-induced PPI gels
Fermentation parameters were established based on previous studies.4,33 A volume of 0.02% w/w inoculum of the commercial starter culture VEGA™ Classic was used to inoculate the PPI base matrices (initial pH 7.0). 0.5% w/w TG was added to half of the samples immediately after inoculation. Inoculated samples were incubated at 43 °C for 8 h until the pH reached 4.5. Gelled samples were stored in individual containers under refrigerated conditions (4 °C) or at room temperature (22 °C) in the dark and the expelled water was drained after 1 week to avoid continuous contact with the gel.
2.4 Gel moisture
Gel moisture was measured for each sample at week 1 and week 16 by weighing gel samples, drying them until the water content was completely evaporated and measuring the sample again. The water content was calculated following eqn (1): |  | (1) |
Gel moisture measurements were performed in technical duplicates for biological triplicates, obtaining a total of six readings for each storage time point.
2.5 Confocal laser scanning microscopy
The microstructure of the fermentation-induced PPI gels was visualized using an SP8 confocal laser scanning microscopy (CLSM, Leica Microsystems, Heidelberg, Germany). Protein was stained with Fast Green FCF and the oil phase stained with Nile Red. Samples were exposed to 488 nm and 633 nm excitation lasers to visualize protein and oil, respectively, following a published method34 and where sequential 2-D and 3-D images were subsequently obtained using 32–36 layers, creating a z-stack depth of ∼10 μm. Image analysis was performed with Imaris (Bitplane, USA) following Ong et al.'s published method.35 The 2D image layers were normalized to compensate for the decrease in fluorescence intensity along the Z-axis, which represents sample depth. The software-determined background intensity was subtracted from each image, and a histogram equalization function was applied to improve image quality. For the 3D images, the processed 2D image layers were stacked together to create a 3D reconstruction of the sample's microstructure. ‘Iso surface’ function in Imaris was used to separately render the fat and protein surfaces. The porosity of the sample was calculated as the total volume of unstained sample. The total volume and mean volume of fat droplets were calculated from the rendered stained fat surface. Two technical replicates of each biological triplicate were visualized, obtaining a total of at least six micrographs per sample. Image processing and analysis was performed with Imaris Microscopy Image Analysis Software (Oxford Instruments, Abingdon, United Kingdom). Three biological replicates of each type of gel were visualized and the quantitative image analysis was performed for three 3D images for each treatment at each time point.
2.6 Texture profile analysis
Samples were subjected to Texture Profile Analysis (TPA) at room temperature (22 °C) after 1, 4, 8, 12, and 16 weeks of storage using a texture analyzer TA-HD (Stable Micro Systems, Godalming, England). Cylinders of 2.5 cm × 2.5 cm were cut with a stainless-steel cylinder from the central part of the samples, and a sample compression of 40% was applied with a 50 mm diameter cylindrical aluminium probe using two compression cycles at a constant crosshead speed of 2 mm s−1 using a trigger force of 3 g. Samples were assessed for hardness and springiness. Hardness was calculated as the maximum force of the first compression, and springiness was calculated as the ratio between the compression distance during the second compression divided by the original compression distance. Compression tests were performed in technical duplicates for biological triplicates, obtaining a total of six readings for each storage time point. Samples temperature was equilibrated to 22 °C before running the analysis.
2.7 Small deformation rheology
The rheological properties of the gelled samples were analyzed in an HR-2 Discovery Hybrid rheometer (TA Instruments, New Castle, USA) equipped with plate-to-plate geometry (flat parallel plate diameter: 40 mm). Slices of each sample (5 mm thickness) were placed in the lower plate and after a resting period of 1 min to release the stress and elevate their temperature to 22 °C, they were compressed between both plates at a 1 mm gap and subjected to a frequency sweep at room temperature from 0.01 Hz to 10 Hz at 0.1% strain, within the previously determined linear viscoelastic region (LVR) of the samples (data not shown). The storage modulus (G′, Pa) and loss modulus (G′, Pa) of each sample was obtained to evaluate their viscoelastic properties. Rheological tests were performed in technical duplicates for biological triplicates, obtaining a total of 6 readings for each time point. Rheological changes during gel formation were followed by fermenting the PPI emulsions in situ under the same conditions explained in Section 2.3 with and without TG using an ElastoSens Bio (Rheolution, Montreal, Canada). Fermentation was performed for gels with and without TG, and G′ was measured every min at 0.1% strain and a fixed frequency of 0.8 Hz and a representative fermentation run presented. Acidification was simultaneously followed in a parallel setting with a pH meter to ensure pH drop. The inflection point, where the maximum increase in the storage modulus occurred, was calculated with the second derivative of the gelation data.
2.8 Statistical analysis
All samples were compared using analysis of variance with a factorial design, where the effect of the presence of TG and the storage time were evaluated. Tukey tests were performed in cases where there were more than two levels per factor, and t-student tests in the case of two levels per factor, to assess significance. The gelation curves were smoothened following the adjacent-averaging method with 10 points used for the window. The software used for the statistical analysis was JMP Pro 16 (SAS Institute, Cary, North Carolina, United States), and p values of less than 0.05 (p < 0.05) were interpreted as significant differences. All analyses were performed with technical duplicates for each biological replicate.
3 Results and discussion
This study was divided into two sections: the first considered the characterization of the gels and the effect of TG in fermentation-induced pea protein emulsion gels; and the second evaluated how textural, rheological, and microstructural properties are affected over a storage period of 4 months under refrigeration at 4 °C. Multi-component colloidal oil-in-water emulsions stabilized by pea protein were fermented in the presence or absence of transglutaminase (TG) into fermentation-induced emulsion gels with a total protein content of 10% and an oil content of 10%. The gels without TG were labelled as “control” gels and the those including TG were referred to as “TG” samples. The structure, gelation dynamics, mechanical and rheological properties were assessed to characterize the gels and evaluate the effect of TG treatment and storage time.
3.1 Structure of the fermentation-induced PPI gel
The model PPI emulsion gels of this study were formed from multi-component colloidal dispersions of PPI in water and oil. The PPI used herein is almost completely denatured as a result of the commercial scale extraction/isolation process,22 confirmed via preliminary fluorescence analysis. The solubility of the PPI suspension was low (22 ± 2%), however, no phase separation was observed over the time taken to prepare emulsions and subsequent gels, likely due to the viscous nature of the high protein suspension. The post-homogenisation heat-treatment ensured that any remaining secondary structure of the globular PPI was unfolded, exposing hydrophobic active sites36 that include the lysine and glutamine residues needed for further crosslinking, as shown by the schematic diagram in Fig. 1. These proteins were then blended with oil to form an oil-in-water Pickering-emulsion, where the oil droplets are stabilized by protein aggregates. This emulsion was then treated with TG to promote covalent crosslinking between protein molecules at the emulsion interface and in solution during fermentation. The structure of the formed gel is a three-dimensional network of protein aggregates supported by protein–protein interactions that entraps both water and the emulsified oil droplets within its pores (Fig. 2). The proteins are expected to form aggregated clusters first and further aggregate to form a heterogeneous gel network during gelation. The structure resembles a particulate percolating network structure, rather than a fine-stranded percolating network.7
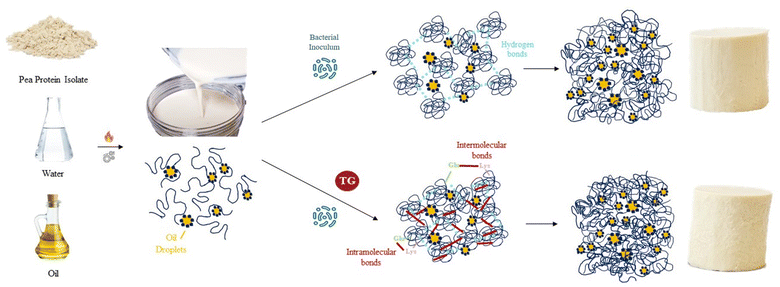 |
| Fig. 1 Schematic diagram of fermentation-induced pea protein gelation; with and without transglutaminase (TG). The pea protein matrix undergoes heat treatment, where proteins unfold and expose their hydrophobic residues. After bacterial inoculation, acidification causes protein aggregation and the establishment of hydrophobic interactions and hydrogen bonds that hold the protein aggregates in a network. In the sample with TG, a tighter network with lower porosity is formed, held also by covalent bonds established by TG (depicted in red), which enhances structural cohesion, leading to differences in textural and rheological properties. | |
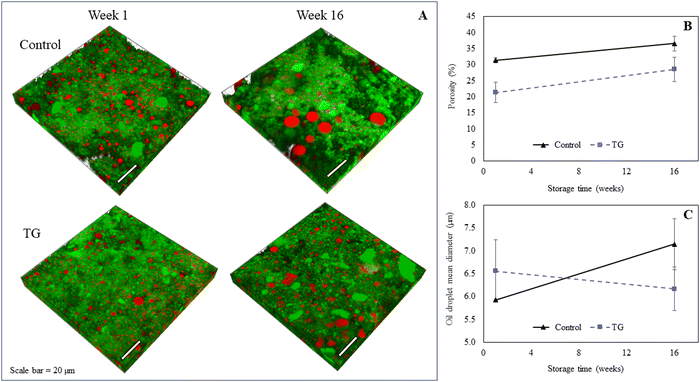 |
| Fig. 2 (A) Microstructure of fermentation-induced pea protein isolate (PPI) gels; without (control) and with transglutaminase (TG), and after 1 and 16 weeks of storage under refrigeration at 4 °C. Protein appears green and oil droplets appear red. Pockets of air/water within the structural network are unstained (black). Scale bar = 20 μm. (B) Porosity (%) in control and TG samples after 1 week and 16 weeks of storage under refrigeration at 4 °C. (C) Oil droplet mean diameter (μm) in control and TG samples after 1 week and 16 weeks of storage under refrigeration at 4 °C. | |
TG was added to the PPI emulsions to enhance the firmness of the structure by creating covalent intramolecular or intermolecular bonds, between exposed glutamine and lysine residues12,13 (Fig. 1). TG has previously shown to increase gel firmness in plant protein gels.13,16–21 As it can be seen in Fig. 3, hydrophobic interactions of the control fermentation-induced pea protein emulsion gel result in a weakly structured gel that is not strong or elastic enough to withstand irreversible deformation after compression. While the microstructure of the gels had a similar visual appearance, quantitative image analysis of the 3D images indicated that the TG treated gels were significantly less porous (Fig. 2), as a result of cross-linking induced by TG treatment, which is known to occur on a nanometer scale. The average oil droplet size was similar for both samples (Fig. 2), reflecting the common process of oil emulsification used to produce both samples.
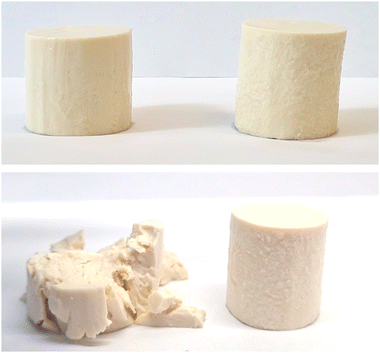 |
| Fig. 3 Fermentation-induced pea protein emulsion gels without TG (left) and with TG (right). The top image shows the gels standing prior to compression, and the lower image shows the gels after compression. | |
3.1.1 Gelation dynamics and gel macrostructure.
The gelation dynamics were investigated in situ during fermentation at 43 °C. The storage modulus of PPI emulsions significantly increased over time for both samples with and without TG (Fig. 4). This increase was expected, as the gradual decrease of pH during fermentation brought the emulsion near the isoelectric point of pea globulins (pH 4.8 for legumin and pH 5.5 for vicilin9), neutralising the negative charges on the pea proteins and promoting hydrophobic interactions between proteins.10
Interestingly, in the control samples, the storage modulus began to rapidly increase after 38 min of incubation at a pH of around 6.8, still far from the isoelectric point of pea globulins. The inflection point of the control sample, where G′ reaches its maximum increase, was after 49 min. This aligns with the findings of Klost et al., who also found the gelation onset of fermentation-induced pea gels to occur at pH 6 within the first few hours of fermentation.37 They also reported these results to be very different to the gelation occurring in dairy protein gels, where it takes place close to the isoelectric point of milk proteins. In their case, however, pH 6 was closer to vicilins isoelectric point (pH 5.5) compared to pH 6.8 here. They concluded that the globulin subunits start aggregating first as their charges decrease, and therefore, are responsible for the initial formation of the gel network.37 The onset of gelation was slower in the presence of TG, with the storage modulus starting to increase after 45 min of incubation, with the inflection point occurring after 77 min (Fig. 4), indicating a subtle difference in aggregation and network formation in these samples. This raises intriguing questions regarding the role of TG in fermentation-induced protein network formation and its impact on the gelation process. In the absence of TG, the proteins naturally interact with each other during fermentation forming a protein network held by non-covalent bonds, such as hydrogen bonds, hydrophobic interactions, and electrostatic interactions. In the presence of TG, the formation of covalent crosslinks between protein molecules is catalyzed by the enzyme.
As shown in Fig. 4, the initial G′ values are higher in the TG sample than in the control sample (8332 ± 3050 compared to 5831 ± 1352 Pa). This indicates that the TG is changing the structuring dynamics immediately after enzyme addition to the PPI solution, namely, the crosslinking starts almost immediately upon the inclusion of TG. This potentially slows down the formation of fermentation-induced hydrogen bonds, compared to the control, by hindering the accessibility of the hydrophobic sites; therefore, slowing down the fermentation-induced protein–protein interactions.38 This results in a delayed gelation onset in the TG sample compared to the control sample where TG-mediated crosslinking may require a longer period to establish sufficient fermentation-derived intermolecular connections between proteins, compared to where hydrogen bonding is allowed to proceed unhindered.
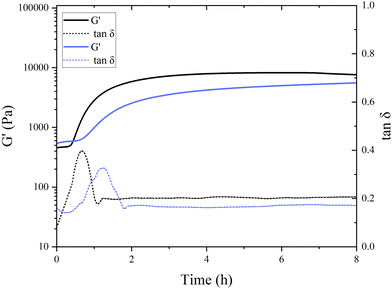 |
| Fig. 4 Gelation kinetics of pea protein emulsion gels with (purple plot) and without transglutaminase (TG) (black plot) during fermentation-induced gelation over 8 h of incubation. The storage modulus (G′) and the loss tangent (tan δ) were recorded every minute at a constant frequency of 0.8 Hz. | |
A different initial rate of gel formation can also be observed between gel systems (Fig. 4). In the initial stages of gelation, a greater number of bonds are established during protein reorganization than those that are dissolved.39 The control sample reaches its maximum within 2–3 h, which is then followed by structural rearrangement and a (presumed) loosening of structure leading to the progressive decrease in gel strength. The TG sample did not reach a stable or maximum G′ value within 20 h of the experiment run, indicating that the gel strength continues to increase upon storage. The gel strength also surpassed the control sample after 12 h. During cooling, rearrangements in the hydrogen bonds in both gels potentially keep taking place, which translates into an increase in gel firmness.40 An increase in short-range interactions that contribute to the enhancement of the protein network has also been reported for heat-induced pea legumin gel systems during the cooling process.41
The initial increase in tan
δ in both samples suggests that the gels are undergoing changes in their viscoelastic properties during the first phases of gelation. Pea protein molecules are undergoing structural changes and interactions that result in increased energy dissipation. The subsequent decrease in tan
δ after reaching its maximum indicates that the gel is setting and its mechanical properties are shifting from more viscous to a more elastic behavior. During this phase, the gel might be strengthening, and the interactions between protein molecules are likely in the process of being stabilized, leading to a decrease in energy dissipation. A similar trend with initial increase and subsequent decrease in tan
δ was also observed in acid-induced gelation of heated milk.42 The subsequent plateau phase after 2 h of incubation suggests that the viscoelastic properties of the gel reached a relatively stable state. At that point, the energy dissipation is relatively low and remains constant.
The visual appearance of the resulting fermentation-induced PPI emulsion gels was not affected by TG treatment, with both presenting as a light yellow/white-colored standing gel resembling a fresh cheese, similar to those previously produced by Masiá et al.4 (Fig. 3). Differences in gel microstructure between the TG and control samples, however, were subsequently shown to influence the physiochemical behavior of the gels, demonstrating the impact of the covalent cross-linking bonds formed in the TG samples on gel behavior.
3.1.2 Rheological behavior.
A slower gelation onset in TG samples, as explained in Section 3.1.1 can be beneficial for a slower rearrangement of the protein aggregates, which after one week of storage under refrigeration, developed into a stronger network in TG samples (Fig. 5 and Table 1). A similar gel-like behavior was observed in samples both with and without TG, where the storage modulus (G′) was higher than the loss modulus (G′′) in the frequency sweeps of the gel samples (Fig. 5).
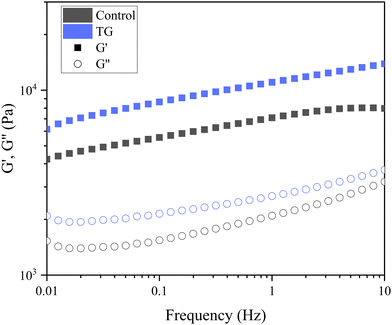 |
| Fig. 5 Frequency sweep of fermentation-induced emulsion gels with and without transglutaminase (TG). The sweep was performed at 0.1% strain, and the plots are an average of six replicated sweeps. | |
Table 1 Storage modulus (G′) and loss modulus (G′′) of fermented pea protein isolate (PPI) emulsion gels with and without transglutaminase (TG) at a frequency of 1 Hz
|
Moduli (Pa) |
G′ |
G′′ |
AB Means with different uppercase superscripts indicate significant differences between different G′ values (p < 0.05).ab Means with different lowercase superscripts indicate significant differences between different G′′ values (p < 0.05). |
Control |
7094 ± 1605B |
2095 ± 516a |
TG |
11 040 ± 3334A |
2427 ± 911a |
The storage modulus measures a materials ability to store elastic energy when subjected to deformation. The sample without enzymatic treatment presented a G′ of 7094 ± 1605 Pa at a frequency of 1 Hz, whereas the sample with TG had a G′ of 11040 ± 3334 Pa (Table 1). These results reflect that the elastic component of the sample with TG was significantly higher, and therefore, firmer, more elastic and more resistant to deformation than the sample without TG. This difference could be explained by the additional covalent crosslinking density of junction points in samples with TG. Although the two gels have different elastic responses, their loss modulus was not significantly different (Table 1), suggesting similar viscous responses. This could be due to similar mobility of the gel components with and without TG. It is important to note that the lack of a significant difference in the loss modulus does not imply that TG has no effect on the viscoelastic behavior of the gel. Instead, it suggests that the changes induced by TG primarily affect the elastic response (storage modulus) of the gel, while the viscous behavior (loss modulus) remains relatively unchanged.
3.1.3 Gel hardness and springiness.
All gels were subjected to a double compression test, and their textural properties were evaluated by their hardness and springiness. Hardness values provide information about the firmness of the gels, whereas springiness values reflect the ability of a sample to recover its structure after being compressed.43 Prior to the compression test, it was not easy to distinguish both samples by eye, whereas their appearance was clearly affected after the compression (Fig. 3). The samples treated with TG were significantly firmer than those without, with a hardness (or maximum force at first compression) of 1153 g and 456 g, respectively (Fig. 6). Moreover, samples containing TG were able to recover 86% of the sample height, whereas those without TG were fractured after the first compression and only recovered 43% of the sample height (Fig. 6), as shown earlier by Shand et al. in heat-induced pea protein gels treated with TG.13
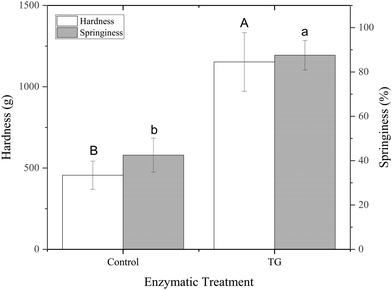 |
| Fig. 6 Hardness (left Y axis) and springiness (right Y axis) of fermented PPI gels with and without TG. AB Means with different uppercase superscripts indicate significant differences between different hardness values (p < 0.05). ab Means with different lowercase superscripts indicate significant differences between different springiness values (p < 0.05). | |
The greater springiness/hardness in TG gels is explained by the additional covalent bonds, which create more junction points throughout the protein stranded network of these samples. Pea protein globulins have previously been reported as a poor substrate for TG, due to their tightly folded structures.44 The mostly denatured PPI used in this study, however, combined with the heat treatment step applied to the emulsions prior to fermentation, results in an unfolded globulin structure, allowing TG access, with 20 lysine and 30 glutamine groups in legumins and 29 glutamine and 32 lysine groups in vicilins/convicilins present in their primary sequence45 and potentially available for TG crosslinking.
These observations align with the findings of Djoullah et al., who reported that native pea globulin can be crosslinked by TG but that protein denaturation can be beneficial for promoting this crosslinking.46 The unfolded proteins in this study are expected to be available to establish covalent bonds when TG is present, strengthening the fermentation-induced protein networks.
3.2 Effect of storage time
3.2.1 Changes in microstructure and water content.
Gels with and without TG were stored for up to sixteen weeks in closed containers at 4 °C, and the structural properties, together with water retention, were assessed as a function of time. After 16 weeks of storage, the CLSM micrographs revealed a similar particulate percolating network of protein aggregates to that observed for week 1 samples (Fig. 2). Quantitative analysis of 3D images, shows an increase in porosity of both gel samples (Fig. 2), indicating some rearrangement of the gel network or change in protein structure as a function of storage time.
A higher degree of oil coalescence was observed for the control samples after 16 weeks of storage, resulting several larger oil droplets distributed throughout the protein network (Fig. 2). Some large coalesced oil droplets were also observed in the week 16 TG samples compared to week 1, however, the majority of lipid droplets appeared to remain intact during storage. These observations were supported by quantitative image analysis of 3D images, which revealed an increase in fat globule size in control samples but not in samples with TG (Fig. 2). This difference in coalescence suggests that the TG-treated samples were better at constraining and stabilizing the oil droplets, due to the crosslinked protein network, despite a small increase in porosity within the network on storage.
Around 1.3% more water was retained in the TG samples compared to the control after 1 week of storage. Although statistically different (Table 2), in practical terms this difference in water content is minimal, and similar levels of moisture (∼5.4–6.5%) were lost across both gel systems as storage continued to week 16 (Table 2). This indicates that while some small degree of syneresis has occurred across both gel systems, concurrent with the increase in porosity for both gels as assessed by quantitative analysis of CLSM micrographs, the addition of TG does not greatly affect the moisture content of fermentation-induced PPI emulsion gels. This observation is in contrast to previous studies on dairy protein gels, where an increase in water retention was reported when TG was present, which was attributed to protein polymerization and enhancement of the protein network.14,47
Table 2 Water content (%) in control samples and samples with TG after 1 week and 16 weeks of storage under refrigeration
|
Storage time (weeks) |
1 |
16 |
AB Means with different uppercase superscripts indicate significant differences between different storage times (p < 0.05).ab Means with different lowercase superscripts indicate significant differences between control samples and TG samples after 1 week and after 16 weeks separately (p < 0.05). |
Control |
79.3 ± 0.2Ab |
73.9 ± 0.8Ba |
TG |
80.6 ± 0.3Aa |
74.1 ± 0.6Ba |
3.2.2 Gel hardness and springiness changes.
No significant differences in hardness were observed as a function of storage time for gels with TG at any of the evaluated time points investigated (weeks 1, 4, 8, 12, and 16) (Fig. 7). The average hardness increased from 1153 g at week 1 to 1660 g at week 16, however, due to the variability between replicate samples. Similarly, no significant differences were observed in the control gels as a function of storage (Fig. 7), although these remained significantly softer than the TG treated samples throughout the storage period. This observation suggests that the maximum gel hardness was reached within the first week of storage and remained relatively stable over the 16 weeks period. No significant differences were observed in the springiness of the gels with or without TG throughout the 16 weeks of storage (Fig. 8). This again indicates that the springiness remained relatively consistent over time (Fig. 8). The limited effect of storage time on the hardness and springiness of gels with or without TG might be correlated to the high moisture content after 16 weeks of storage (Table 2). It is also consistent with the assumption that TG activity following fermentation was low, due to the acidic pH after fermentation and the low refrigeration temperature (4 °C), since the optimal pH for a 100% relative activity of TG lies between pH 5 and 8 according to the manufacturers guidelines and the optimal temperature of TG is around 50 °C.48,49 It was not possible to inactivate the TG via heat-treatment after fermentation, in this study, as this would have inactivated the microorganisms present in the gels. A future study could track TG activity over time, although the obtained gel hardness and springiness values indicate that TG activity was minimal under the conditions of storage examined in this study.
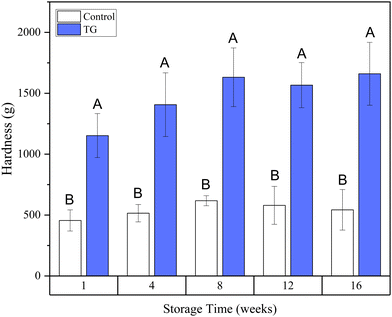 |
| Fig. 7 Development of hardness over time in fermentation-induced pea protein isolate (PPI) emulsion gels with and without transglutaminase (TG). AB Means with different uppercase superscripts indicate significant differences between samples with and without TG (p < 0.05). | |
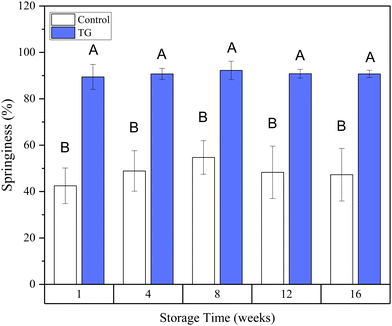 |
| Fig. 8 Development of springiness over time in fermentation-induced pea protein isolate (PPI) emulsion gels with and without TG. AB Means with different uppercase superscripts indicate significant differences between samples with and without TG (p < 0.05). | |
3.2.3 Rheological changes.
The rheological characterization of the control and TG gel systems provided valuable insights into the viscoelastic behavior of these soft matter systems over time. The storage moduli remained higher after TG treatment compared to control samples for all storage time points (Fig. 9). This finding suggests that TG treatment reinforces and strengthens the gel, promoting the formation of a network that is more robust against deformation over time due to covalent glutamine–lysine crosslinks that remain stable with time. This aligns with the lower porosity of the TG treated samples observed for all timepoints. The occurrence of larger oil droplets in the control sample may also contribute to the weaker gel structure, acting as a structure breaker, similar to differences observed for dairy cheese prepared from milk containing smaller and larger distributions of milk fat globules.50 These observations indicate that the nature of the bonding in the pea protein emulsion gel systems did not change significantly with time, despite the small increase observed in gel porosity.
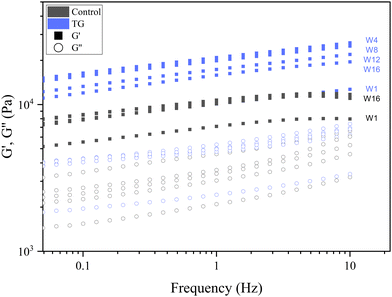 |
| Fig. 9 Frequency sweeps of fermentation induced pea protein isolate (PPI) emulsion samples with and without transglutaminase TG after 1, 4, 8, 12, and 16 weeks of storage under refrigeration. | |
Whilst the storage modulus measured during storage was highly variable (Fig. 10), particularly for TG treated samples, an increasing trend in G′ was observed in the early stages of the storage period between weeks 1 and 4, after which the storage modulus seems to plateau and stabilize, e.g., from (5.83 ± 1.35 × 103 Pa to 8.35 ± 0.68 × 103 Pa for the control and 8.3 ± 3.0 × 103 Pa to 17.2 ± 5.8 × 103 Pa for the TG sample). The upward trend in G′ between weeks 1 and 4 is likely due to the continued formation of fermentation-induced hydrogen bonds in both gel systems. Some structural rearrangement may also occur in the protein network with time, as indicated by the increase in porosity, detected in both samples with 3D image analysis and increase in oil coalescence, observed for control gels (Fig. 2). These findings indicate that TG promotes higher storage moduli during storage, indicating a reinforced gel structure. It is important to highlight the two different processes occurring in these gels: fermentation-induced gelation occurs in both samples but also a more intensive protein aggregation occurs in the TG sample, due to crosslinking. These processes might be responsible for the heterogeneity observed over time and the higher variability observed for TG treated samples for both storage modulus and hardness. Whilst proteolysis was not measured in the current study and could be a focus for further work, the data on hardness (Fig. 7) and storage modulus (Fig. 10), indicate that a significant portion of the protein network remains intact over the short storage times examined here, even with a slight increase in porosity in both gel types, suggesting that proteolysis does not significantly impact the physical properties of the pea plant gel systems, although rearrangement of protein networks may occur during storage.
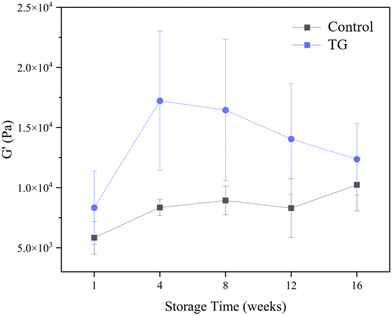 |
| Fig. 10 Storage modulus (G′) of fermented pea protein isolate (PPI) gels with and without TG after 1, 4, 8, 12, and 16 weeks of storage under refrigeration at 4 °C, at a frequency of 1 Hz. | |
Interestingly, when a similar set of samples was stored at 22 °C for 16 weeks, rather than 4 °C, the microstructure, textural and rheological properties resembled those measured for samples stored under refrigerated conditions (data not shown). As samples were equilibrated to the same temperature for testing, it can be assumed that the storage at 4 °C and 22 °C did not affect the structural or functional properties of the gels. However, the storage temperature, as well as the storage time, could affect other parameters, such as flavour. Further elucidation of the volatile and non-volatile compounds of samples stored at different storage periods and temperatures may therefore demonstrate differences in enzymatic activities of the microorganisms and could provide insights into the aroma and flavour profiles of fermentation-induced PPI emulsion gels.
4 Conclusions
This study determined the effect of TG on the microstructure, textural and rheological properties of fermentation-induced 10% w/w pea protein isolate emulsion gels stored for up 16 weeks. The TG samples gelled slower than the control samples, resulting in less porous, firmer and more elastic gels. While the structure of both gels appeared to be a particulate percolating network, the cross-linking in TG treated gels led to properties most closely resembling those formed during production of traditional dairy-based cheese. The difference in gel formation kinetics was likely due to the covalent bonds formed through TG addition, which hindered access to hydrophobic sites, slowing the formation of fermentation-derived protein–protein interactions but leading to the formation of a more robust cross-linked network. More pronounced coalescence of oil droplets into fewer and larger pockets of oil was observed for the control, likely because of a more porous and weaker protein network structure. Some rearrangement of protein bonds and the network appears to occur once gels have formed and cooled, as shown by the increase in porosity in both gel types. The limited change in textural attributes from week 4 onwards and similar levels of water loss/retention with time, however, suggest pea protein emulsion gels formed with or without TG are both relatively stable upon storage.
Future investigations could assess the influence of other factors, such as temperature and pH fluctuations during storage, to gain a comprehensive understanding of the pea gel stability and functionality during prolonged storage periods. The ability to further process pea gels to form a range of other structures could also be assessed. In conclusion, this study demonstrates the positive effect of transglutaminase on the microstructure, texture, and rheological properties of fermentation-induced pea protein emulsion gels. The formation of a less-porous and robust cross-linked network through TG addition highlights the potential of protein cross-linking to achieve dairy-like properties in plant-based protein gels. These findings contribute to our understanding of how TG can be utilized as a valuable tool in the design and formulation of novel plant-based cheese products with enhanced textural characteristics and stability. As the demand for sustainable and plant-based alternatives continues to grow, this research opens new avenues for creating improved pea protein-based products that can meet consumer preferences and expectations.
Author contributions
Carmen Masiá: conceptualization, methodology, software, validation, formal analysis, investigation, data curation, writing – original draft, writing – review and editing, visualization, project administration, funding acquisition; Lydia Ong: conceptualization, methodology, formal analysis, software, validation, investigation, resources, writing – review and editing, visualization, supervision, project administration; Amy Logan: methodology, validation, investigation, resources, data curation, writing – review and editing; Regine Stockmann: draft, writing – review and editing; Joanna Gambetta: writing – review and editing; Poul Erik Jensen: writing – review and editing, supervision, project administration, funding acquisition; Saeed Rahimi Yazdi: writing – review and editing, visualization, supervision, project administration; Sally Gras: conceptualization, methodology, validation, resources, writing – review and editing, supervision, project administration.
Conflicts of interest
There are no conflicts to declare.
Acknowledgements
Sally Gras and Lydia Ong are supported by The Dairy Innovation Hub. The authors thank the Biological Optical Microscopy Platform (BOMP) at The Bio21 Molecular Science and Technology Institute for granting access to confocal microscopy and image analysis software, and Olga Glagovskaia from CSIRO Agriculture and Food for nitrogen analysis of the solubilised PPI suspensions.
Notes and references
- M. Bayrak, J. Mata, J. K. Raynes, M. Greaves, J. White, C. E. Conn, J. Floury and A. Logan, J. Colloid Interface Sci., 2021, 594, 561–574 CrossRef CAS PubMed.
- X. D. Sun and S. D. Arntfield, Food Hydrocolloids, 2012, 28, 325–332 CrossRef CAS.
- S. Drusch, M. Klost and H. Kieserling, Curr. Opin. Colloid Interface Sci., 2021, 56, 101503 CrossRef CAS.
- C. Masiá, P. E. Jensen, I. L. Petersen and P. Buldo, Foods, 2022, 11, 1–13 CrossRef PubMed.
- C. Masiá, S. Keshanidokht, L. D. Preisler, J. Risbo and P. E. Jensen, LWT – Food Sci. Technol., 2023, 182 DOI:10.1016/j.lwt.2023.114890.
- E. C. Short, A. J. Kinchla and A. A. Nolden, Foods, 2021, 10, 725, DOI:10.3390/foods10040725.
- T. Nicolai, Adv. Colloid Interface Sci., 2019, 270, 147–164 CrossRef CAS.
- E. A. Foegeding, Food Biophysics, 2006, 1, 41–50 CrossRef.
- C.-E. Danielsson, Acta Chem., 1950, 762–771 CrossRef CAS.
-
M. S. Klost, PhD thesis, Technischen Universität Berlin, 2021.
- M. Klost, G. Giménez-Ribes and S. Drusch, Food Hydrocolloids, 2020, 105, 105793 CrossRef.
- C. Marco, G. Pérez, P. Ribotta and C. M. Rosell, J. Sci. Food Agric., 2007, 87, 2576–2582 CrossRef CAS PubMed.
- P. J. Shand, H. Ya, Z. Pietrasik and P. K. J. P. D. Wanasundara, Food Chem., 2008, 107, 692–699 CrossRef CAS.
- W. El Kiyat, E. Laurenthia, J. Michaela and R. F. Pari, ASEAN J. Sci. Technol. Dev., 2021, 38, 83–88 Search PubMed.
- A. Sayadi, A. Madadlou and A. Khosrowshahi, Int. Dairy J., 2013, 29, 88–92 CrossRef CAS.
- T.-j Lim, A.-m Easa, A.-a Karim and R. Bhat, Br. Food J., 2011, 113, 1147–1172 CrossRef.
- X. D. Sun and S. D. Arntfield, Food Hydrocolloids, 2011, 25, 25–31 CrossRef CAS.
- E. M. Herz, S. Sch, N. Terjung, M. Gibis and J. Weiss, Food Sci. Technol., 2021, 1, 1412–1417 CAS.
- S. Ben-Harb, M. Panouillé, D. Huc-Mathis, G. Moulin, A. Saint-Eve, F. Irlinger, P. Bonnarme, C. Michon and I. Souchon, Food Hydrocolloids, 2018, 77, 75–84 CrossRef CAS.
- Y. Nie, Y. Liu, J. Jiang, Y. L. Xiong and X. Zhao, Food Hydrocolloids, 2022, 129, 107607 CrossRef CAS.
- M. Yang, N. Li, L. Tong, B. Fan, L. Wang, F. Wang and L. Liu, LWT – Food Sci. Technol., 2021, 152, 112390 CrossRef CAS.
- H. M. Moreno, C. A. Tovar, F. Domínguez-Timón, J. Cano-Báez, M. T. Díaz, M. M. Pedrosa and A. J. Borderías, Food Sci. Technol., 2020, 40, 800–809 CrossRef.
- O. Ogilvie, S. Roberts, K. Sutton, N. Larsen, J. Gerrard and L. Domigan, Food Chem., 2021, 340, 127903 CrossRef CAS PubMed.
- F. Zhan, X. Tang, R. Sobhy, B. Li and Y. Chen, Int. J. Food Sci. Technol., 2022, 57, 974–982 CrossRef CAS.
- A. Salinas-Valdés, J. D. l R. Millán, S. O. Serna-Saldívar and C. Chuck-Hernández, J. Food Sci., 2015, 80, 2950–2956 CrossRef PubMed.
- Y. Li, X. Zhang, J. J. Yang, X. Y. Ma, X. D. Jia, P. Du and A. Li Li, J. Food Process. Preserv., 2020, 44, 1–11 Search PubMed.
- Y. Y. Li, R. C. Yu and C. C. Chou, J. Agric. Food Chem., 2010, 58, 4888–4893 CrossRef CAS PubMed.
- Y. L. Ma, J. H. Wang, Y. Q. Cheng, L. J. Yin and L. T. Li, Int. J. Food Eng., 2013, 9, 45–54 CrossRef CAS.
- R. C. Lawrence, L. K. Creamer and J. Gilles, J. Dairy Sci., 1987, 70, 1748–1760 CrossRef CAS.
- F. Boukid, C. M. Rosell and M. Castellari, Trends Food Sci. Technol., 2021, 110, 729–742 CrossRef CAS.
- S. Qamar, B. Bhandari and S. Prakash, Food Res. Int., 2019, 116, 1374–1385 CrossRef CAS PubMed.
-
M. C. Tulbek, R. S. Lam, Y. C. Wang, P. Asavajaru and A. Lam, Pea: A Sustainable Vegetable Protein Crop, Elsevier Inc., 2016, pp. 145–164 Search PubMed.
- C. Masiá, R. Fernández-Varela, P. E. Jensen and S. R. Yazdi, Future Foods, 2023, 8, 100250 CrossRef.
- L. Ong, R. R. Dagastine, S. E. Kentish and S. L. Gras, LWT – Food Sci. Technol., 2011, 44, 1291–1302 CrossRef CAS.
- L. Ong, R. R. Dagastine, S. E. Kentish and S. L. Gras, Food Res. Int., 2012, 48, 119–130 CrossRef CAS.
- E. Dickinson, Food Hydrocolloids, 2011, 25, 1966–1983 CrossRef CAS.
- M. Klost and S. Drusch, Food Hydrocolloids, 2019, 94, 622–630 CrossRef CAS.
- V. D. Truong, D. A. Clare, G. L. Catignani and H. E. Swaisgood, J. Agric. Food Chem., 2004, 52, 1170–1176 CrossRef CAS.
- H. H. Winter and M. Mours, Adv. Polym. Sci., 1997, 134, 164–234 CrossRef.
- K. F. Grasberger, S. B. Gregersen, H. B. Jensen, K. W. Sanggaard and M. Corredig, Food Struct., 2021, 29, 100198 CrossRef CAS.
- F. E. O’Kane, R. P. Happe, J. M. Vereijken, H. Gruppen and M. A. Van Boekel, Heat-induced gelation of pea legumin: Comparison with soybean glycinin, J. Agric. Food Chem., 2004, 52(16), 5071–5078 CrossRef PubMed.
- J. A. Lucey, M. Tamehana, H. Singh and P. A. Munro, Food Res. Int., 1998, 31, 147–155 CrossRef CAS.
- P. Zhu, W. Huang, X. Guo and L. Chen, Food Hydrocolloids, 2021, 117, 106705 CrossRef CAS.
- C. Larré, M. G. Chenu, G. Viroben, J. Gueguen and Z. M. Kedzior, J. Agric. Food Chem., 1992, 40, 1121–1126 CrossRef.
- T. U. Consortium, Nucleic Acids Res., 2021, 49, D480–D489 CrossRef PubMed.
- A. Djoullah, Y. Djemaoune, F. Husson and R. Saurel, Process Biochem., 2015, 50, 1284–1292 CrossRef CAS.
- T. Aaltonen, I. Huumonen and P. Myllärinen, Int. Dairy J., 2014, 38, 179–182 CrossRef CAS.
- M. Motoki and K. Seguro, Trends Food Sci. Technol., 1998, 9, 204–210 CrossRef CAS.
- R. Garcia Alvarez, P. Karki, I. E. Langleite, R.-J. Bakksjø, L. A. Eichacker and C. Furnes, FEBS Open Bio, 2020, 10, 495–506 CrossRef PubMed.
- A. Logan, M. Xu, L. Day, T. Singh, S. C. Moore, M. Mazzonetto and M. A. Augustin, Int. Dairy J., 2017, 70, 46–54 CrossRef CAS.
|
This journal is © The Royal Society of Chemistry 2024 |