DOI:
10.1039/D4MA00122B
(Review Article)
Mater. Adv., 2024,
5, 3629-3643
Hydrogels as local depots for on-demand therapeutic delivery: potential therapeutic approaches for tumor metastasis
Received
8th February 2024
, Accepted 26th March 2024
First published on 4th April 2024
Abstract
Tumor metastasis, the spread of tumor cells from their place of origin to surrounding tissues and then distant organs through vasculatures, is the major cause of cancer mortality. Despite the recent advances in therapeutic approaches for tumor metastasis, the therapeutic efficacy is still unsatisfactory. Fruitful treatment strategies for tumor metastasis largely depend on targeting tumor cells, the molecular and cellular features of circulating tumor cells, the TME, and the regulatory components of the TME. However, traditionally administered therapeutic agents in biological fluids are highly prone to inactivation, often fail to reach the target site, cause adverse effects, and exhibit poor pharmacokinetics with a short half-life. To overcome these limitations, hydrogel-based delivery systems are investigated as local depots for sustained and stable release to combat tumor metastasis. Herein, we review the extensive application of hydrogels in the local delivery systems of potential therapeutic agents for tumor metastasis therapy. This review summarizes the recent advances in tumor metastasis inhibition strategies and potential therapeutic approaches using hydrogels for the local delivery of various therapeutic agents.
1. Introduction
Metastasis is a multistep process in which cancer cells spread from a primary tumor to colonize distant organs.1 It is a major cause of death in cancer patients. Metastatic cancer patients have limited treatment options and significantly lower survival rates than patients with localized cancer. Systemic chemotherapy, the first line of treatment for metastatic cancer, fails to target metastatic cancer cells; has other serious side effects such as off-target accumulation, toxicity, and rapid renal and hepatic clearance; and has the potential to induce metastasis.2,3 It has been reported that over 90% of failures in chemotherapy occur during the invasion and metastasis of cancers.4 Recently, advanced treatment options such as immunotherapy, epigenome-modifying agents, and combination therapy have reduced toxicity in metastatic cancer patients but have limited efficacy in inhibiting tumor metastasis.5 Despite the advances in therapeutic modalities and innovations in the development of active therapeutic options, the complexity of the multistep process creates a huge burden for the development of effective therapies.6–8 However, the growing knowledge of the metastatic cascade has a significant impact on guiding therapeutic innovation and delivery systems.5,6,9
Localized biomaterial-assisted delivery systems for metastatic cancer therapeutic agents have received much attention to overcome the limitations of systemic chemotherapy and have the potential to directly deliver therapeutic agents to target pathological sites.10,11 This provides an efficient delivery strategy to prevent post-surgical tumor recurrence and metastasis by achieving precise, high-dose controlled release and prolonged exposure of cancer cells to therapeutics. Meanwhile, a single local administration of therapeutics can reduce the side effects of systemic therapy and improve patient compliance.12 Thus far, among various biomaterial-supported delivery systems, hydrogels are promising materials for various biomedical applications, including drug and other therapeutic agent delivery systems. Hydrogels are 3D polymeric network materials with structural similarity to natural extracellular matrixes that are frequently investigated as delivery vehicles for therapeutic agents. They are an ideal material for encapsulation and utilized as localized depots for therapeutic agents and offer improved delivery efficiency and specificity to the area of interest.13,14 Moreover, they have been used as 3D models of different diseases such as tumor models,15 cell encapsulation for enabling highly efficient tissue regeneration,16 and functionalization with special materials for bioimaging and biosensing.17 A hydrogel can be engineered from natural or synthetic materials to have distinct properties and features desirable for biomedical applications via various preparation methods. The steps and mechanisms of metastasis as well as the application of hydrogels have been extensively reviewed. This review discusses hydrogel-based local delivery systems for current and potential therapeutic approaches that control or prevent metastases. It highlights the recent advances in the use of hydrogels as local depots for the sustained delivery of therapeutic agents for tumor metastasis by targeting various steps in the metastatic process.
2. Research methodology
A search for original and review articles was conducted from various indexed journals reporting hydrogel-based local therapy for tumor metastasis. The following search terms were used for the literature search: hydrogel, delivery system, tumor metastasis, local delivery, in situ gel, chemotherapy, immunotherapy, phototherapy, and metastasis cascade from search engines, PubMed, Scopus, Google Scholar, and Web of Science databases. Advanced searches were performed from these databases such as (((hydrogel)) and (local delivery)) and (tumor metastasis). This review included original peer-reviewed studies based on the following criteria: (1) publication in an English-language journal; (2) papers related to local delivery systems for tumor transplantation; (3) all papers were included based on therapeutic approaches, inhibition of metastasis, and long-term local treatment delivery; and (4) no limitation has been placed on the date of publication.
3. Targeting metastatic cascade
Metastasis is a highly complex process in which cancer cells detach from the primary site and go through the following steps (metastatic cascade): local invasion, intravasation, circulation, extravasation, and colonization, to form a secondary tumor in distant parts of the body (Fig. 1). Infiltration of tumor cells into adjacent tissues begins with complete loss of intercellular adhesion molecules of tumor cells, which allows the cells to move together as detached clusters or single cells.18 Several factors such as external stress, nutrient and oxygen deficiencies, chromosomal instability, and morphological and phenotypical changes of tumor cells create favorable conditions for tumor cells to invade the surrounding tissues.19 For the most part, metastasis initiation genes play a critical role and can promote cell motility, epithelial–mesenchymal transition (EMT), extracellular matrix degradation, angiogenesis, or evasion of the immune system.20 The epithelial-to-mesenchymal transition plays a role in invading and invasive properties of tumor cells, which reduces the adhesion of tumor cells with each other and the extracellular matrix (ECM), resulting in detachment, invading adjacent tissues and intravasation of cancer cells into the circulation.21
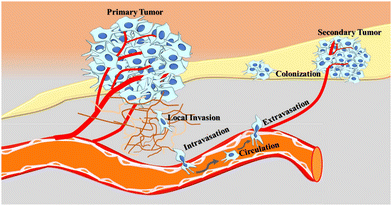 |
| Fig. 1 Metastatic cascade: invasion, intravasation, circulation, extravasation, and colonization. | |
Once the cell loses polarity and detaches from surrounding cells, it migrates through the extracellular matrix (ECM) into the blood and lymphatic vessels.22 Determining factors for the intravasation of tumor cells into the circulation could be the intrinsic properties of cancer cells, tumor microenvironment, mechanical cues, a feature of newly formed blood vessels, and accessibility of tumor cells to vessels.23 Rapid cellular division and growth of cancer cells increase the need for nutrition (change in metabolism) and oxygen (hypoxia) for each cell, which is believed to be the main reason for the formation of blood vessels (angiogenesis) and lymphatic vessels (lymphangiogenesis). Newly formed blood vessels of primary tumors are malformed, highly branched, and leaky, allowing tumor cells to escape from the primary site.24,25 Once tumor cells enter the bloodstream as single cells or in a clustered form, most circulating tumor cells (CTCs) die in the circulation, possibly due to physical stress, oxidative stress, anoikis, and the lack of growth factors and cytokines, and only a small percentage (0.01%) of cells can give rise to metastases. To escape from ensuing barriers in the bloodstream and generate a metastatic lesion, CTCs acquire key specific features: stem-like phenotype, hybrid epithelial–mesenchymal transition (EMT) state, escape from the immune system, low sensitivity to therapy, resistance anoikis and circulatory shear stress.26–28 In addition, it has been reported that platelets adhering to CTCs act as a shield against fluid sheer stresses (FSS), prevent cytolysis by natural killer cells, hide CTCs from immune cell recognition, and secrete growth factors that help CTCs grow in the circulation.29 Besides protection from immune cell attack and cytolysis, platelet adhesion to CTCs also reveals valuable impacts for tumor cell adhesion and extravasation to distant organs.30
The metastatic potential of tumor cells is not limited to surviving the hostile intravascular environment but also relies on their ability to exit the bloodstream and cross the endothelium to invade the surrounding tissue. The process of tumor cells exiting the bloodstream through transendothelial migration and invading the surrounding tissue is called extravasation. This process involves three major events that include adhesion of CTCs to vessel walls, remodeling of the endothelial barrier, and transendothelial migration (TEM).31 In most cases, extravasation of tumor cells is paracellular migration, where tumor cells migrate between two endothelial cells. Such form of extravasation relies on the interaction of tumor cells with blood cells, ligands, and receptors.32 In rare cases, tumor cells can migrate transcellularly by penetrating individual cell bodies. After successful extravasation, most cancer cells do not result in metastases but form micrometastases and become dormant. When micrometastases escape from dormancy, cancer cells interact with the microenvironment to form a pre-metastatic niche that helps to restart the growth of tumor cells at the secondary site. A suitable pre-metastatic niche creates favorable conditions for cancer cells to develop angiogenic switches and recruit various cells that enhance local tissue and microenvironments that facilitate the growth and expansion of the metastases.24,33
4. Therapeutic approaches for tumor metastasis
Unlike primary tumors, treating metastasis is challenging and every therapeutic approach does not effectively target metastatic cancers, often limited by their inability to eliminate already-seeded non-proliferative, growth-arrested, or therapy-resistant tumor cells. Although metastases are still the leading cause of cancer death, advances in cancer biology and the science of the metastatic cascade have the potential to improve the treatment of tumor metastasis (Table 1). This growing knowledge of the science of the stages of metastasis provides an opportunity to identify key drivers of metastasis and regulators of cancer cell migration, to distinguish the biological differences between micro- and macro metastases, to target the susceptibility of metastatic cancer cells, and to exploit the properties of metastatic tumor microenvironments, which provide an excellent basis for a therapeutic approach to metastasis.34,35
Table 1 Potential anti-metastatic therapeutic approaches
Target |
Approach |
Advantages |
Limitations |
Ref. |
Primary lesions/postoperative residual cancer |
Chemotherapy |
→ Idea implantation at tumor resection cavity |
→ Limited by their inability to target metastatic cancer cells and eliminate seeded non-proliferative, growth-arrested, and therapy-resistant tumor cells |
56
|
PTX-NPs-DN hydrogel |
→ Sustained-release PTX over ten days and higher accumulation in local tissue |
→ Avoids systemically side effects |
Immunotherapy (iSGels@aOX40) |
→ Relieving the immunosuppressive microenvironment at the tumor resection bed |
→ Low response rate |
57
|
→ Effective stimulating systemic antitumor immune responses and memory effects |
→ Immune-related adverse events |
Combination therapies CTX&aPDL1@Gel |
→ Sequential release of CTX and aPDL1 |
→ Low response rate for circulating cancer cells |
58
|
→ Selective depletion of regulatory T cells (Treg) |
→ Inhibition of cancer recurrence after surgery |
→ Stimulation in systemic immune and T memory cells |
Phototherapy |
→ Ultrasimple synthesis and administration procedures |
→ Low photostability |
59
|
ICG–alginate hydrogel |
→ Excellent biocompatibility avoidance of the leakage from injection sites |
→ Potential toxicity of ICG |
→ Improvement of the accumulation in tumors |
→ Poor aqueous stability |
→ High-performance PTT |
Reprogramming tumor microenvironment |
Chemo-immunotherapy |
→ Reduces the numbers of both myeloid derived suppressor cells and Tregs in tumor microenvironment |
→ Initial burst release of DOX from the hydrogels leads to toxicity of normal cells |
60
|
ATC/DOX@mPEG-b-PELG |
→ Promotes the efficacy of adoptively transferred T cells against solid tumors |
Hypoxia inhibitors |
→ Combination of hypoxia alleviation and photodynamic therapy (PDT) to improve anticancer efficacy |
→ Hypoxia can be expressed in some normal tissues and drugs targeting hypoxia can have serious side effects |
61
|
Prolonged oxygen-generating phototherapy hydrogel (POP-Gel) |
→ POP-Gel elevate the oxygen level and significantly increasing immune efficacy |
Angiogenesis inhibitors |
→ Downregulates the expression of VEGF-A and Ang-2, destroys angiogenesis in tumors and reduces the density of micro-vessels |
→ Anti-angiogenic therapies reduce drugs delivery to tumors |
62
|
PDLX-PMI hydrogel |
→ Inhibit the proliferation of cancer cells and inhibit tumor metastasis |
Effective therapeutic approaches to metastatic cancer should not only target the disseminated cancer cells themselves but also consider the mechanisms of cancer cell transformation and the processes involved in the metastasis cascade. Strategies such as targeting tumor microenvironment, molecular and cellular features of circulating tumor cells, cancer–immune interactions, and other regulator components of the metastatic tumor microenvironment are particularly promising therapeutic approaches.36 The tumor microenvironment consists of various cellular components including tumor cells, cancer-associated fibroblasts, mesenchymal stromal cells, pericytes, adipocytes, vasculature, lymphatic networks, myeloid populations, myeloid-derived suppressor cells, immune cells, and inflammatory cells, and non-cellular components such as extracellular matrix, matrix remodeling enzymes, cytokines, chemokines, exosomes, growth factors, and inflammatory enzymes.37 Angiogenesis promotes tumor growth by providing oxygen and nutrients and is essential for tumor metastasis as a route for cancer cell dissemination.24 Therefore, targeting tumor-induced angiogenesis has been suggested as a potential tumor metastasis prevention strategy. Another potential approach in metastasis therapy/prevention is targeting the molecular and cellular features of metastatic circulating tumor cells.38 Similarly, targeting different steps in the metastatic cascade with therapeutics, such as antibodies, are promising candidates for inhibiting tumor cell metastases.39 Not all disseminated tumor cells (DTCs) are guaranteed to metastasize, as a few DTCs enter a growth arrest (dormancy) until conditions are favorable to reactivate and reinstate their cancerous nature. At this stage, the cells are non-dividing and resistant to therapeutics, as most anti-tumor treatments target cell division. Therefore, targeting dormant tumor cells through their metastasis suppressors has been of therapeutic interest in treating metastasis. Metastasis suppressor inhibits metastasis by keeping the disseminated tumor cells in a state of dormancy.40 RECK is a membrane-anchored protein regulator, which is often downregulated in several types of cancers. As described by Yoko et al., enforced expression of RECK in tumor cells by a potent RECK-inducer, DSK638, inhibits metastasis.41 Myeloid-derived suppressor cells (MDSCs) are immature myeloid cells that are important for PMN formation and accumulate in patients with cancer. Pre-metastatic niche (PMN) is a preconditioned microenvironment that is a key determinant of cancer metastasis.42 Since MDSCs play a critical role in PMN formation, it is realistic to design MDSC-based strategies to combat PMN.43 Lu et al. demonstrated that targeting myeloid cells using adjuvant epigenetic therapy disrupts pre-metastatic microenvironment formation and prevents metastasis through a selective effect on myeloid-derived suppressor cells (MDSCs).44 In general, identifying and targeting the key drivers of tumor metastasis, such as epithelial–mesenchymal transition (EMT),45,46 angiogenesis,47 the ECM,48–50 circulating cancer cells,51,52 micro- and macro-metastasis, and their molecular-drivers53 (including their interaction with cancer cells at the various stages of metastasis), are the potential therapeutic strategies for metastatic cancer.54,55
5. Hydrogels for the local delivery of drugs for tumor metastasis therapy
Hydrogels are three-dimensional water-swollen networks of physically or chemically linked polymers, proteins, small molecules, or colloids. They can be prepared from natural or synthetic materials, via physical or chemical interactions, in different sizes (nano-gels, micro- and macroscopic gels), and conventional and stimuli-responsive forms.63–65 Hydrogels are a suitable and versatile platform for delivery due to their ability to encapsulate and preserve pharmaceuticals and provide sustained and remotely programmable release.64,66 In biomedical applications, hydrogels mostly offer four basic properties: biocompatibility, biodegradability, drug-loading ability, and controlled drug release.67 Due to these and other unique properties, hydrogels have gained considerable interest in therapeutic agent delivery systems. Delivery approaches for therapeutic agents require outstanding improvements to achieve the desired therapeutic effect and avoid side effects. In the therapeutic area, hydrogel-based local delivery systems are promising platforms and have received tremendous attention due to their advantages over conventional delivery systems.68,69 Maximizing the efficacy of the treatment depends on the dose of drug that reaches the target site, so therapy is carried out at the target site through localized delivery platforms.70 Local delivery systems have the ability to deliver high doses of therapeutic molecules at the target site and provide controlled and sustained drug molecule release with minimized nonspecific distribution of therapeutics in healthy tissues.71,72 Hydrogel-based local drug delivery system is one of the long-acting drug delivery formulations that allow controlled drug release over days, weeks, months, and years after single-dose administration and a stable pharmacokinetic profile. It is best suited for patients who require long-term therapy, such as prostate cancer patients who receive 365 injections of Lupron Depot Therapy per year. Hydrogels can strongly entrap and encapsulate drugs and short-half-life molecules such as proteins and small particles through networks of mesh size and molecular interaction, which reduces clearance rate and increases retention time at the injection site.73 It can be the protective delivery vehicle of therapeutics and avoid premature degradation. As a local delivery platform, hydrogels can increase the concentration of therapeutics at the injection site, which improves antitumor efficacy, stimulates local immune response, and reduces off-target effects, systemic toxicity, and systemic reactions. Hydrogels can be used to encapsulate hydrophilic and hydrophobic molecules and release both molecules simultaneously, sequentially, or at completely different times, making them ideal for the codelivery of therapeutics for synergistic effects. Hydrogels are attractive for the controlled delivery of therapeutic agents such as chemotherapeutic drugs, immunosuppressive agents, antibodies, vaccines, cytokines, and immune cells.70 Despite the interesting properties of hydrogels in drug delivery systems, some limitations still exist (Table 2). Because they are soft, hydrophilic, and wet materials, hydrogels are limited to encapsulating hydrophobic drugs and have relatively low tensile strengths. Therefore, strengthening and appropriate modification of their (supra)molecular structures and physicochemical properties have been the main focus of research on hydrogel biomedical applications. Extensive research has been conducted on strategies to develop hydrogels with (supra)mechanical properties and improve their tissue adhesiveness, drug loading capacities, environmental responsiveness, and drug release kinetics. Various designing strategies have been developed to overcome these limitations and to achieve high stretchability and toughness, including the modification of network structures,74 such as the formation of double networks and dual physical contact, as well as the incorporation of different nanomaterials in the hydrogels.75 Hydrogels with tunable properties are intended to achieve desired local drug delivery systems and exhibit the necessary mechanical and biochemical properties for controlled drug release without side effects. The most explored strategy to achieve on-demand localized drug delivery is the formation of ‘‘smart’’, dynamic hydrogels that can respond to environmental stimuli.76,77 These smart hydrogels can provide a controlled and sustained delivery system of therapeutics in a time-dependent manner and minimally invasive procedures. Among the potential hydrogel designs, smart injectable hydrogels that respond to external stimuli are gaining attention and are optimal candidates for on-demand local delivery systems with minimally invasive procedures.
Table 2 Limitations of hydrogels as drug delivery systems and possible solutions
Limitations and disadvantages |
Types of hydrogel material/example |
Possible solutions |
Ref. |
Non-biocompatible and non-biodegradable properties |
Poly(N-isopropylacrylamide) based hydrogels |
Introduce biodegradable, biocompatible linker into PNIPAM backbone |
78
|
Toxicity of small cross-linkers molecules |
Glutaraldehyde cross-linked polymeric hydrogels |
Polymer–polymer cross-linking or hybrid polymer networks (HPN) |
79
|
Slow stimuli response and |
Poly(N-isopropylacrylamide-co-2-hydroxyethylacrylate (HEA)) hydrogels cross-linked via di-selenides |
Fine-tuned by adjusting the stimuli and incorporating of several stimuli in a single polymer |
80
|
Initial burst drug release |
Poly(ethylene glycol) hydrogels |
Designee hybrid membranes of hydrogel to prolong drug release and better encapsulate hydrophobic drugs |
|
Poor encapsulation of hydrophobic drugs |
Hydroxypropyl methylcellulose (HPMC) hydrogels |
Introduction of molecules capable of forming inclusion complexes and/or the incorporation of hydrophobic moieties |
80
|
Weak mechanical characteristics |
Poly(N-isopropylacrylamide) (PNIPAAm) hydrogel |
Copolymerization and development of hybrid materials with natural polysaccharides |
81
|
Loaded drugs may alter responsiveness of hydrogels |
Ibuprofen and salicylate sodium salts loaded 2-(2-methoxyethoxy) ethyl methacrylate (MEO MA), 2-hydroxyethyl methacrylate (HEMA) and ethylene glycol 2 dimethacrylate (EGDMA) (100 : 2 : 1 mol ratio) |
Understanding of drugs and polymer ratio, interaction of drugs and hydrogel moieties, and drug binding moieties |
82
|
Drug deactivation and during polymer binding |
Chemical bonding loading strategies proteins, peptides and small molecules in chitosan hydrogels |
Understanding of the trends and cross-linking technologies hydrogels formation |
83
|
6. Hydrogels for the local delivery of chemotherapeutics for tumor metastasis
Locally targeted chemotherapy offers an opportunity to overcome the limitations of conventional systemic chemotherapy: off-target delivery with significant adverse effects of chemotherapeutic agents. Drug carriers such as hydrogels have attracted much attention for effective local delivery of drugs to achieve therapeutic efficacy with low administration frequency, thereby alleviating systemic toxicity, avoiding the inconvenience of multiple injections, and preventing the development of resistance during repeated drug administration.73,84 Most notably, the properties of hydrogels can be tuned and improved according to the raw materials used and preparation methods. The most important property of local drug delivery is their injectability, which is minimally invasive and targeted implantation even in poorly accessible tissue sites. An injectable hydrogel is a type of in situ gel-forming material that requires the sol (pre-gel) to be transported to the target site and sol–gel transition at a specific time interval at the implanted site.85 It has been widely explored as a local drug delivery system for single drug delivery, multidrug co-delivery, and other combination therapies.86–88
In recent decades, various injectable hydrogels have been investigated as local delivery platforms for tumor metastasis chemotherapy. Leng et al. designed an injectable hyaluronic acid (HA)-based hydrogel loaded with paclitaxel (PTX) nanoparticles and epirubicin (EPB) (PPNPs/EPB@HA-Gel) for the treatment of tumor recurrence and metastasis.89 Encapsulated drugs (paclitaxel (PTX) and epirubicin (EPB)) were steadily released from the hydrogel to inhibit the proliferation and migration of tumor cells, thereby preventing long-term tumor recurrence and metastasis in a post-operative breast tumor model. As a nutrient delivery pathway for tumor cells, angiogenesis has been a hot target for metastasis therapeutic approaches to inhibit tumor growth and dissemination. When the nutrition continuously travels through the blood vessels to the tumor cells, the tumor cells also spread through it, which ultimately results in cancer cell growth and metastasis. Thus, disruption of nutrient supply and prevention of angiogenesis is a promising strategy to prevent tumor metastasis. Wang et al. prepared a photo-responsive injectable hydrogel using a polydopamine cross-linked collagen/silk fibroin composite to deliver thrombin to occlude blood vessels and prevent angiogenesis.90 Localized delivery of vascular disrupting agents through an injectable thermosensitive PLGA–PEG–PLGA copolymer to tumor-bearing mouse models has been investigated as a promising tumor metastasis preventive strategy.91 These vascular disruptive agents can significantly inhibit the proliferation of cancer cells and prolong the survival rate of tumor-bearing mouse models.
Pancreatic cancer is an aggressive form of cancer with a dismal prognosis. The lack of any reliable early diagnostic marker of the disease makes early diagnosis challenging, and most patients are diagnosed at a later stage after the disease has advanced or metastasized.92,93 Surgical resection and chemotherapy are front-line treatments for local stages of pancreatic cancer. A remarkable feature of injectable hydrogels in the treatment of metastasis is the minimally invasive local implantation in such poorly accessible tissues with a fine needle. Yang et al. designed an endoscopic ultrasound-guided thermo-sensitive injectable hydrogel (PLGA–PEG–PLGA, PPP) for local sustained chemotherapy to pancreatic tumors.73 This fine-needle-injectable in situ gel-forming material demonstrated sustained delivery of the drug and the best therapeutic effect, significantly reducing the proliferation, invasion, and migration of pancreatic cancer cells. Similarly, Braet et al. demonstrated a pH-sensitive hydrogel-based intraperitoneal drug delivery approach for the treatment of peritoneal metastases, which was a more promising drug delivery method for unresectable peritoneal metastases, maintaining cytotoxicity and delaying tumor growth.94
Although surgery is currently considered one of the main treatment modalities for cancer, local post-surgical cancer recurrence and metastasis formation of disseminated cancer cells through cut blood vessels greatly affect the prognosis of cancer and the patient's survival rates. Cancer-associated adipocytes and angiogenesis in the postoperative tumor microenvironment significantly affect the recurrence and metastasis of tumor cells. Effectively killing residual tumor cells, simultaneously inhibiting the function of cancer-associated adipocytes and angiogenesis, may be a promising strategy to prevent tumor recurrence and metastasis after surgery. Li et al. studied anticancer drugs (doxorubicin and metformin) encapsulated in pH-responsive dopamine and 3-aminobenzeneboronic-acid-grafted hyaluronic acid hydrogels for implantation into the tumor surgical cavity.95 Sustained-release drugs effectively killed residual tumor cells, hindered adipocyte-stimulated tumor proliferation and migration, and inhibited tumor angiogenesis, leading to significant inhibition of tumor recurrence and metastasis after surgery. Injectable in situ formed hydrogel is a suitable local drug delivery alternative post-surgical cavity implant for the prevention of cancer recurrence and distant metastasis.96–99 Overall, hydrogel-based local delivery of chemotherapy can combat tumor metastasis by eradicating tumor cells,100 remodeling the tumor microenvironment,101,102 such as blocking the function of cancer-associated adipocytes to inhibit adipocyte-stimulated tumor cell proliferation, cell migration, and angiogenesis,66 and inducing immunogenic cell death103 (Fig. 2).
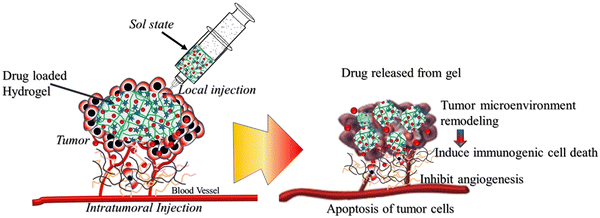 |
| Fig. 2 Hydrogel-based chemotherapeutic approaches to metastatic cancer via targeting tumor microenvironment, inducing immunogenic cell death and inhibiting angiogenesis. | |
7. Hydrogels for the local delivery of phototherapeutic agents for tumor metastasis
Phototherapy, which includes photodynamic therapy (PDT) and photothermal therapy (PTT), is a treatment modality that has been widely used in recent years to destroy tumor cells by exposure to light without damaging normal tissue. Both PDT and PTT are minimally invasive antitumor approaches that are applied in combination with light activation of a photosensitizer (PS) and a photothermal agent (PTA), respectively (Fig. 3). After activation by light, PS produces reactive oxygen species (ROS), which can oxidize nearby biological macromolecules on tumor cells, causing cytotoxicity of tumor cells, while the photothermal agent (PTA) converts light energy into heat energy and kills tumor cells, as the tumor cells are more sensitive to high temperatures than normal cells.104–106 Although phototherapy has been highly successful in tumor therapy, traditional phototherapeutic administrations have been associated with phototoxicity, limiting clinical applications. Sustained and controlled delivery of phototherapeutic agents directly to tumor sites is an effective antitumor phototherapy that minimizes off-target effects in normal tissues. In recent years, hydrogels in antitumor phototherapy have gained great interest due to their ability to deliver phototherapeutic agents in a localized site and minimize the distribution of phototherapeutic agents in non-target sites.107–109 The application of hydrogels as photothermal agents and photosensitizer carriers has been extensively investigated for antitumor PTT and PDT. Jin et al. achieved a successful combination of photothermal therapy (PTT) and photodynamic therapy (PDT) incorporating gold nanorods (AuNRs) and methylene blue (MB) into a multifunctional hydrogel.110 Yao et al. synthesized thermosensitive Pluronic F127 hydrogels and loaded with titanium carbide nanoparticles as photothermal agents to achieve excellent photothermal therapeutic effects against breast cancer.111 In another study, Pan and co-workers developed an indocyanine green-alginate hydrogel for local tumor ablation. The in situ synthesized hydrogel exhibits strong indocyanine green accumulation ability in tumors and performs remarkable local PPT of tumors.59 Similarly, Zhang et al. designed an injectable thermosensitive hydrogel as an effective carrier of UZC (NaYF4:Yb3+, Tm3+ (UCPs), Zn2GeO4:Mn2+ (ZGM), and g-C3N4); photosensitizers, under 980 nm laser irradiation, can generate ROS and lead to the destruction of tumor cells.112 Moreover, Wang et al. successfully incorporated photosensitizer chlorin e6 and manganese dioxide (MnO2) nanoparticles into the stimulus-responsive injectable hydrogel to achieve synergistic photodynamic/photothermal therapy of breast cancer.113
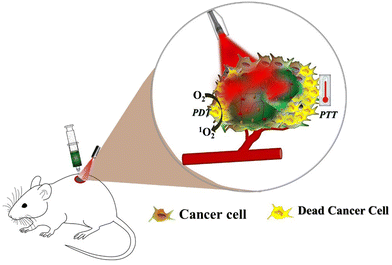 |
| Fig. 3 Schematic illustration of the hydrogel-based localized delivery platform for anti-tumor PTT/PDT. | |
8. Hydrogels for the local delivery of immunotherapeutic agents for tumor metastasis
The immune system can recognize, target, and eliminate cancer cells, whereas cancer cells struggle to resist the immune system and develop tumors. Cancer cells that overcome immune defense can grow into tumors and interact with a variety of host cells to create a pro-tumor microenvironment that promotes invasion and metastasis. Cancer cells that survive against the elimination by immunosurveillance accumulate various genetic and epigenetic changes, which provide cancer cells the stealth ability to escape immune system.114,115 Those that escape from the immune system form a tumor, establish a tumor micro-environment, and accumulate malignant properties to settle in a metastatic niche, eventually forming metastatic tumors.
Recently, immunotherapies have been considered revolutionary therapeutic strategies and breakthrough in the treatment of metastatic disease.116,117 Representative types of immunotherapeutic strategies used in tumor metastasis are immune checkpoint blockade, cytokine therapy, cancer vaccines, and adoptive T cell therapy.118–121 Immunotherapies combat tumor metastasis either by limiting the immunosuppressive ability of cancer cells or boosting the host immune system to promote their ability to target cancer cells.122 Targeting cancer stem cells, tumor microenvironment, and key signaling pathways through immunotherapy can yield dramatic results in preventing metastasis. Tumor-initiating cells play a crucial role in cancer metastasis, though immunotherapy is an ideal treatment option to eliminate cancer stem cells and halt cancer metastasis.123
Immunosuppressive tumor microenvironment (TME) has a significant impact on tumor metastasis, so modulating the tumor microenvironment is one way to improve immune function and inhibit tumor metastasis. Remodeling the tumor microenvironment promotes immune cell infiltration, suppresses tumor angiogenesis, and enhances the anti-tumor effects of drugs, thereby restraining tumor metastasis.124 Recently, remodeling the tumor microenvironment has attracted the attention of researchers as a therapeutic target for cancer metastasis (Fig. 4). Han et al. investigated the delivery of anti-tumor drugs and immunostimulants (baicalin, antigen, and CpG-ODN) to a melanoma-bearing mouse model, thereby remodeling the tumor microenvironment and killing tumor cells.125
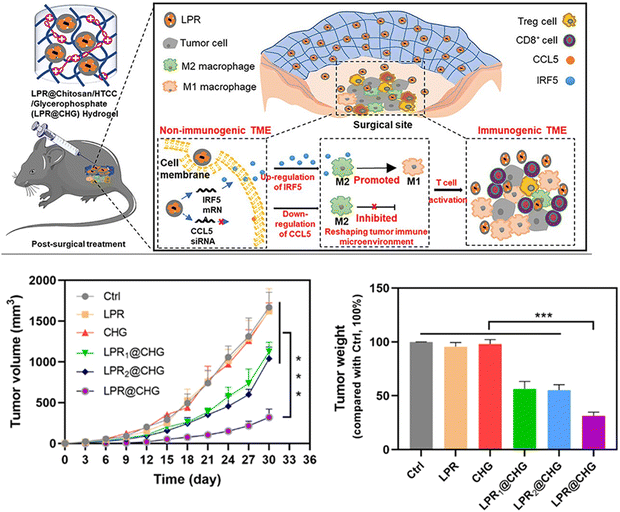 |
| Fig. 4 Approach used to target the TME for tumor metastasis: modulating the immunosuppressive tumor microenvironment via macrophage infiltration and controlling the tumor growth by initiating T-cell-mediated immune responses.126 Copyright © 2022, American Chemical Society. | |
Although immunotherapy has attracted tremendous attention as a treatment modality, low response rates and severe systemic immune-related adverse events limit the efficacy and overall impact of therapy.127 Such drawbacks are mainly attributed to the systemic administration of high dosages or multiple injections of immunotherapeutic agents. The intratumoral delivery approach is a promising method for immunotherapeutic agents to reduce systemic side effects and combat mechanisms of tumor immune suppression within the tumor microenvironment. In particular, smart hydrogels are highly desirable for local delivery, allowing controlled and sustained release of immunotherapeutic agents to tumor sites to enhance antitumor immune responses and inhibit metastasis and tumor recurrence.128–130 Most previous studies have used injectable hydrogels as local delivery platforms for immune-mediated and immunotherapeutic agents. Immunotherapeutic agents, chemotherapeutic drugs, photosensitizers, combinational chemo-immunotherapy, and photoimmunotherapy can be encapsulated into injectable hydrogels, resulting in sustained and controlled release at target sites to mediate immunotherapy. For instance, Song et al. developed a PEGylated polypeptide injectable hydrogel for local co-delivery of tumor vaccine and immune checkpoint inhibitors.131 Sustained release of vaccines and immune checkpoint inhibitors efficiently recruited endogenous dendritic cells and elicited strong humoral and cellular responses, thereby inhibiting tumor growth and attenuating the immunosuppressive tumor environment. Zhuang et al. developed hydrogel-based combinational therapy (photothermal agents, chemotherapeutics, and immune adjuvants) for metastasis inhibition. Similarly, Liu et al. demonstrated the local delivery of an immunogenic cell death (ICD) inducer and immune checkpoint blockade (ICB) therapy through injectable hydrogels to induce antitumor immune response and T-cell-mediated immune responses.132
Immunogenic cell death (ICD): a crucial mechanism of action of tumor immunotherapy is induced by various chemotherapies.133 The general principles of chemotherapies to stimulate immunotherapy efficacy are: (1) controlled and sustained release of chemotherapeutics from locally implanted hydrogels, (2) tumor cell immunogenic cell death (ICD) with tumor-associated antigens (TAAs) and damage-associated molecular pattern (DAMPs), (3) maturation of dendritic cells, and (4) release damage-associated molecular patterns (DAMPs) attracted by dendritic cells (DCs), thereby stimulating the maturation of lymphocytes and the anti-tumor immune response134 (Fig. 5). Wang et al. designed an injectable thermosensitive hydrogel loaded with oxaliplatin (OxP) and resiquimod (R848) to induce immunogenic cell death. Upon local injection, the hydrogel exhibits sustained release of the drugs that are cytotoxic and enhance the immunogenicity of tumors by inducing immunogenic cell death.135
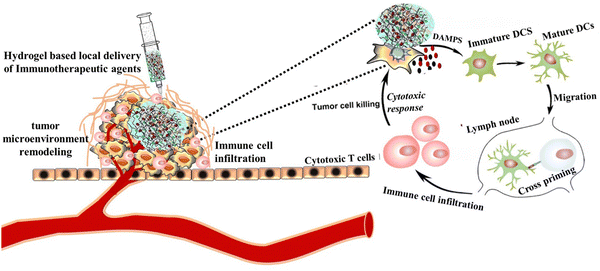 |
| Fig. 5 Schematic illustration of immunogenic cell death (ICD) induction on tumor cells via hydrogel-based local delivery of therapeutics to improve the efficacy of immunotherapy. | |
9. Hydrogel-based local delivery of combination therapeutics for tumor metastasis
Despite continuous improvements in tumor metastasis inhibition and tumor therapeutic approaches, tumor cells’ adaptive mechanisms to escape the host's immune system, drug resistance, and toxicity are the basis of treatment failure and tumor progression. Combination therapy is the most effective therapeutic approach that combines two or more therapeutic agents to target different pathways within tumors and the tumor microenvironment, which aids in tumor progression and metastasis. It is more effective in targeting target tumor heterogeneity, drug resistance, and killing cancer stem cells. Over mono-therapeutic approaches, combination therapy enhances efficacy and decreases toxicity due to the synergistic or additive principle of action that requires lower dosage of each therapeutic agent, addresses heterogeneous nature of tumors, and strikes cancer cells in more than one place. In combination therapy, the therapeutic potential of some drugs to produce a synergistic effect depends on the sequence, making it difficult to design simultaneous combinations. In addition, the synergistic effects of combination therapies strongly depend on the effective combination of multiple therapeutic molecules in a single platform rather than simple mixing (Fig. 6). With recent advances in drug delivery systems, hydrogels have the potential to assemble multiple therapeutic agents (drugs, cells, growth factors, and particles) into a single platform without losing bioactivity via chemical or simple physical interactions.86,136–138
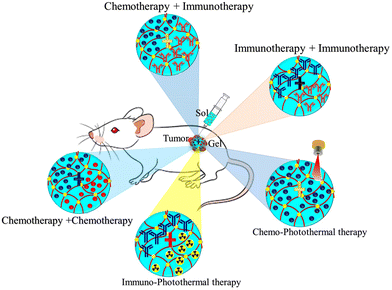 |
| Fig. 6 Schematic illustration of the potential application of hydrogels for local delivery of combination therapeutics of tumor metastasis. | |
Recently, various investigations have been conducted to explore the application of injectable hydrogels as local delivery platforms of combination therapeutics for tumor metastasis (Table 3). Xiao et al. designed multifunctional injectable alginate hydrogels for synergistic tumor combination therapy.139 Hydrogels (GPI/R848@ALG) were prepared by simply mixing alginate (ALG) sols with glucose oxide (GOx)-conjugated polyacrylic acid stabilized-iron oxide nanoparticles (GPI NPs) and the Toll-like receptor 7/8 agonist resiquimod (R848). Glucose oxidase (GOx) blocks the glucose supply for starvation therapy, Fe3O4 NPs boost the Fenton reaction efficacy for enhanced chemodynamic therapy, and resiquimod (R848) reprograms the TAMs towards M1-like phenotype for the inhibition of tumor progression. Combined chemo-photothermal therapy has been reported to produce synergistic effects and enhance therapeutic index by enhancing cell membrane penetration, drug release at the target region, and drug cytotoxicity. Combined chemo-photothermal therapy has been reported to produce synergistic effects and enhance the therapeutic index by improving cell membrane penetration, drug release at the target region, and drug cytotoxicity. Sun et al. designed a hydrogel-based chemo-photothermal combination therapy for localized NIR-triggered drug release to enhance antitumor effects.140 Similarly, Hu et al. synthesized tumor-microenvironment-responsive injectable nanocomposite hydrogels (Au NBPs&Pt NCs@DOX gel) for synergistic photothermal, chemotherapeutic, and chemodynamic cancer therapies.141 Localized chemo-photothermal therapies are promising therapeutic strategies to eradicate primary tumor cells but not likely distant metastasis. Besides directly killing primary tumor cells, metastasis requires a systemic tumor-specific immune response. Zhuang et al. developed injectable hydrogel-based combinational therapy photothermal agents, chemotherapeutics, and immune adjuvants to inhibit metastasis.142 Lu and co-workers investigated the combination of a hydrogel vaccine system containing a granulocyte-macrophage colony-stimulating factor (GM-CSF) and programmed death-ligand-1 antibody (αPD-L1) in a post-surgical mouse tumor model.143 This locally implanted combination therapy induced the production of powerful antigen-specific T cells and stimulated an anti-tumor immune response, which effectively inhibited tumor recurrence and metastasis. Similarly, Wang et al. investigated hydrogel-based local delivery of combined sonodynamic therapy (SDT) and chemodynamic therapy (CDT) for breast cancer and inhibition of tumor metastasis144 (Fig. 7). An in situ formed alginate-based hydrogel was loaded with manganese dioxide (MnO2) nanoparticles, an organic polymer, and an SIS3 drug as the CDT agents, sonosensitizer for SDT and therapeutic drug for inhibiting tumor metastasis respectively. Upon exposure to ultrasound (US) radiation at the tumor site, the hydrogel generated hydroxyl radicals (˙OH) and singlet oxygen (1O2) through CDT and SDT effects to induce tumor cell death. Zhou et al. also studied hydrogels loaded with a combination of antitumor drugs and monoclonal antibodies for the inhibition of postoperative tumor recurrence and metastasis145 (Fig. 8). Sustained release of the drug and monoclonal antibody resulted in CXCR4 inhibition, ICD induction, and PD-L1 blockage, which directly turned the residual tumor seeds into an in situ vaccine and remotely disturbed the PMN. Overall, local delivery of combination therapeutics has shown remarkable success in killing tumor cells, controlling the tumor microenvironment, and providing benefits over monotherapy in various tumor metastases.
Table 3 Recent reports on hydrogel-based local delivery for cancer metastasis
Functional materials |
Application/advantages |
Limitations |
Ref. |
PDLLA-PEG-PDLLA |
• Sustained co-delivery of 5-fluorouracil and cis-platinum |
→ In vivo burst release |
99
|
• Thermo-sensitive and injectable |
• Synergistic combination chemotherapy |
• Communitive release time: more 30 days |
Niche-like CS-FTP-gel (chitosan polymer) |
→ In situ gel forming, ROS-responsive niche-like hydrogel for AQ4N prodrug sustained release |
→ Not applicable in relatively low hypoxic or oxygenated tissue environment |
146
|
→ Utilized for remodeling of tumor hypoxic microenvironment |
→ Use relatively non-toxic crosslinker |
DSDMA–SBMA zwitterionic hydrogels |
→ Co-delivery of sunitinib (Sun) and chlorin e6 (Ce6) |
→ Use of chemical crosslinker |
71
|
→ Redox-responsive zwitterionic hydrogel |
→ Initial burst drug release within 2 hours |
→ Injectable and in situ gel forming |
Poly(acrylic acid-co-4-vinylphenylboronic acid) nanohydrogels (P(AA-co-4-VPBA) NG) |
→ Codelivery of combretastatin-A4 phosphate (CA4P) and doxorubicin (DOX) |
→ Poor encapsulation of hydrophobic drugs |
147
|
→ Loading and release of hydrophilic drug and nanogel |
→ Release of drug in normal physiology without nanogels |
→ Releasing time up to14 days |
Chitosan LPR@CHG hydrogel |
→ In situ injectable thermosensitive hydrogel |
→ Receptor dependent delivery system |
126
|
→ Release immunotherapeutic to reprogram the antitumoral immune niche |
→ Need to deliver in cellular organelle label |
→ Less toxic and minimally invasive |
Uracil grafted N-isopropylacrylamide (NIPAM) |
→ Achieve in situ gel formation at 37 °C |
→ Less targeted and diffusion-based release |
148
|
→ Free cross-linking agents due the presence of multiple hydrogen bonding |
→ Minimally invasive material for tumor treatment |
Bombyx mori silk fibroin (BMSF) and Antheraea assamensis silk fibroin (AASF) blended hydrogels |
→ Injectable hydrogel for co-delivery of drugs |
→ Drug–drug interaction and drug deactivation |
149
|
Anticancer peptide (KL) based KPFD hydrogels |
→ Used as therapeutic hydrogel |
→ Less space for co-encapsulation of drugs with photothermal |
150
|
→ Surve as synergistic therapeutic effect (combinational photothermal–chemotherapy) |
→ Inducing membrane destabilization and facilitating the cell uptake drugs |
Methacrylated polymers combinations (DexMa-CsMa) |
→ Sustained and controlled release |
→ Initial burst release |
151
|
→ For topical treatment approach |
→ Drug–polymer interaction |
→ Bio-adhesive on a biological simulating membrane |
ICG–alginate hydrogel |
→ Ultrasimple synthesis and administration procedures |
|
59
|
→ In situ fabrication of the photothermal hydrogel |
PQ/PB-Gel |
→ Simple preparation and administration method |
|
152
|
→ Low production cost |
→ Excellent efficacy and low toxicity |
ERT@HMSNs/PLEL |
→ In situ physically crosslinked non-flowing gel at physiological temperature |
→ Stimuli responsiveness is influenced by loaded materials |
153
|
→ Long term sustained release |
→ Need cell membrane targeting and penetration moieties |
→ Nano-scaled delivery system for localized anticancer therapies |
PTX@BN/4aPEG-OPA |
→ Tissue-adhesive ability |
→ Polymer–drug interaction |
154
|
→ Immobilization of drug-loaded systems at tumor sites to increase local drug concentration |
→ Slow-release rate |
→ Long term release over 30 days |
CPN@GO-CET/CPT11@shRNA hydrogel |
→ Multifunctional drug/gene delivery system |
→ Initial burst release within 2 h |
155
|
→ Ligand-mediated active targeting and the pH-triggered drug release features |
→ In situ gel formation |
→ Co-delivery of anticancer drugs and siRNA |
ES/HA-Tyr hydrogels |
→ Sustained release |
|
156
|
→ Lower systemic toxicity, and better anti-tumor effects |
GEM/(PLGA–PEG–PLGA, PPP) hydrogel |
→ Exhibit excellent injectability, biocompatibility, and the capability of sustained drug delivery |
→ Slow stimuli response |
73
|
→ In situ forming hydrogel |
→ Burst release |
PVLA-PEG-PVLA hydrogel |
→ Sustained delivery of drugs |
→ Slow stimuli response |
157
|
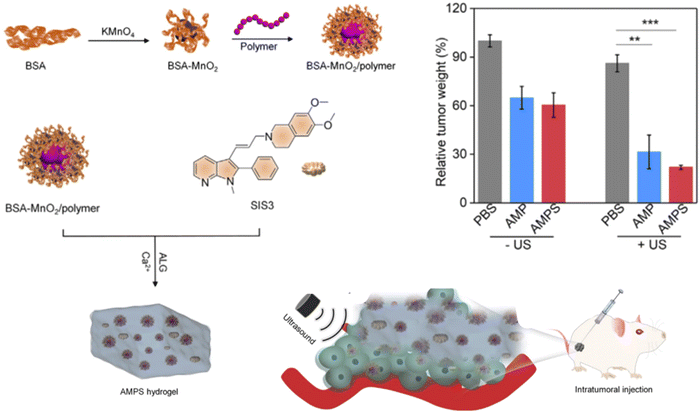 |
| Fig. 7 Schematic illustration of the in situ formed alginate-based hydrogel for SDT-CDT combination therapy for tumor metastasis inhibition,144 Copyright 2023, Wang et al. | |
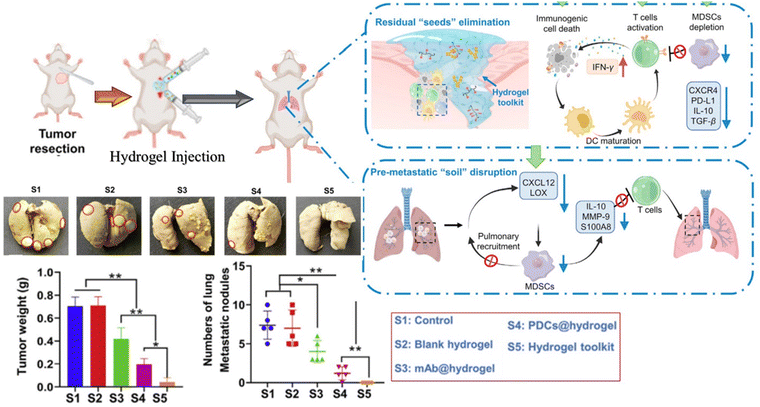 |
| Fig. 8 Illustration of the in situ formed hydrogel for the inhibition of postoperative tumor recurrence and metastasis,145 Copyright 2022, Chinese Pharmaceutical Association and Institute of Materia Medica. | |
10. Conclusions and future perspectives
Tumor metastasis is a deadly event and a complicated problem in clinical tumor therapy. Despite the advances in therapeutic modalities and innovations in the field, metastasis remains the leading cause of cancer-related death. Several factors are involved in the migration of cancer cells and the formation of tumor metastasis. Targeting the entire process of metastasis is explored as a novel therapeutic approach to inhibit tumor metastasis by intervening in any steps of tumor cell migration. Various therapeutic agents have recently been developed with the potential to induce primary tumor cell apoptosis, modulate TME, and eradicate circulating tumor cells. However, off-target accumulation with severe side effects in the systemic administration of these therapeutic agents has impeded their therapeutic application. Owing to the unique properties of biocompatibility, embedding biologically active agents, and controlled release capacity, hydrogels have been shown to have great potential in the delivery of therapeutic agents in tumor metastasis. Moreover, hydrogel-based localized delivery systems have received much attention to overcome the limitations of systemic therapy and provide effective delivery to target pathological sites through a controlled release manner, resulting in long-term exposure to tumor cells to therapeutics. This review highlighted the recent applications of hydrogels as local delivery systems to inhibit tumor metastasis. Hydrogels, in particular smart hydrogels, can serve as a perfect platform for localized depots and sustained release of drugs in chemotherapy, radiotherapy, phototherapy, immunotherapy, and/or combination therapy to reduce the patient's multiple dose burden, making them promising for the future treatment of tumor metastasis. Smart hydrogels can intelligently respond to environmental stimuli and can be delivered less invasively, thereby enabling the remotely controlled and on-demand release of therapeutic agents. The significance of a hydrogel-based localized delivery approach over systemic therapy is to expand the range of therapeutic inhibition of tumor metastasis, reduce systemic toxicity and repeated dosing, and enhance patient compliance, which will improve the quality of clinical responses.
Conflicts of interest
The authors declare no conflict of interest.
Acknowledgements
We are grateful for the financial support from RX Biotech PTE. LTD for the publication of this work.
References
- L. R. Patel, D. F. Camacho, Y. Shiozawa, K. J. Pienta and R. S. Taichman, Future Oncol., 2011, 7, 1285–1297 CrossRef CAS PubMed.
- J.-x Su, S.-j Li, X.-f Zhou, Z.-j Zhang, Y. Yan, S.-l Liu and Q. Qi, Acta Pharmacol. Sin., 2023, 1–12 Search PubMed.
- M. C. Pope, M. C. Olson, K. T. Flicek, N. J. Patel, C. W. Bolan, C. O. Menias, Z. Wang and S. K. Venkatesh, Diagn. Interv. Radiol., 2023, 29, 571–578 CrossRef PubMed.
- B. Mansoori, A. Mohammadi, S. Davudian, S. Shirjang and B. Baradaran, Adv. Pharm. Bull., 2017, 7, 339–348 CrossRef CAS PubMed.
- M. Castaneda, P. den Hollander, N. A. Kuburich, J. M. Rosen and S. A. Mani, Semin. Cancer Biol., 2022, 87, 17–31 CrossRef CAS PubMed.
- A. Schegoleva, A. Khozyainova, T. Gerashchenko, L. Zhuikova and E. V. Denisov, Clin. Exp. Metastasis, 2022, 39, 505–519 CrossRef CAS PubMed.
- J. Majidpoor and K. Mortezaee, Med. Oncol., 2021, 38, 3 CrossRef PubMed.
- A. A. Schegoleva, A. A. Khozyainova, T. S. Gerashchenko, L. D. Zhuikova and E. V. Denisov, Clin. Exp. Metastasis, 2022, 39, 505–519 CrossRef CAS PubMed.
- J. Ko, M. M. Winslow and J. Sage, EMBO Mol. Med., 2021, 13, e13122 CrossRef CAS PubMed.
- Q. Chen, M. Chen and Z. Liu, Chem. Soc. Rev., 2019, 48, 5506–5526 RSC.
- F. Weiss, D. Lauffenburger and P. Friedl, Nat. Rev. Cancer, 2022, 22, 157–173 CrossRef CAS PubMed.
- H. Shan, K. Li, D. Zhao, C. Chi, Q. Tan, X. Wang, J. Yu and M. Piao, Front. Pharmacol., 2020, 11, 573 CrossRef CAS PubMed.
- H. F. Darge, A. T. Andrgie, E. Y. Hanurry, Y. S. Birhan, T. W. Mekonnen, H. Y. Chou, W. H. Hsu, J. Y. Lai, S. Y. Lin and H. C. Tsai, Int. J. Pharm., 2019, 572, 118799 CrossRef CAS PubMed.
- H. Ma, C. He and X. Chen, Macromol. Biosci., 2021, 21, e2100039 CrossRef PubMed.
- K. Fernando, L. G. Kwang, J. T. C. Lim and E. L. S. Fong, Biomater. Sci., 2021, 9, 2362–2383 RSC.
- P. Khayambashi, J. Iyer, S. Pillai, A. Upadhyay, Y. Zhang and S. D. Tran, Int. J. Mol. Sci., 2021, 22, 684 CrossRef CAS.
- S. Wei, Z. Li, W. Lu, H. Liu, J. Zhang, T. Chen and B. Z. Tang, Angew. Chem., Int. Ed., 2021, 60, 8608–8624 CrossRef CAS.
- N. V. Krakhmal, M. V. Zavyalova, E. V. Denisov, S. V. Vtorushin and V. M. Perelmuter, Acta Nat., 2015, 7, 17–28 CrossRef CAS.
- G. Pani, T. Galeotti and P. Chiarugi, Cancer Metastasis Rev., 2010, 29, 351–378 CrossRef CAS PubMed.
-
T. A. Martin, L. Ye, A. J. Sanders, J. Lane and W. G. Jiang, Madame Curie Bioscience Database [Internet], Landes Bioscience, 2013 Search PubMed.
- C. J. Creighton, D. L. Gibbons and J. M. Kurie, Cancer Manage. Res., 2013, 187–195 CrossRef PubMed.
- M. Zavyalova, E. Denisov, L. Tashireva, O. Savelieva, E. Kaigorodova, N. Krakhmal and V. Perelmuter, Biochemistry, 2019, 84, 762–772 CAS.
- M. K. Sznurkowska and N. Aceto, FEBS J., 2022, 289, 4336–4354 CrossRef CAS PubMed.
- P. d H. M. Castaneda, N. A. Kuburich, J. M. Rosen and S. A. Mani, Semin. Cancer Biol., 2022, 87, 17–31 CrossRef PubMed.
- S. P. Chiang, R. M. Cabrera and J. E. Segall, Am. J. Physiol.: Cell Physiol., 2016, 311, C1–c14 CrossRef PubMed.
- I. Tinhofer, M. Saki, F. Niehr, U. Keilholz and V. Budach, Int. J. Radiat. Biol., 2014, 90, 622–627 CrossRef CAS PubMed.
-
T. B. Steinbichler, D. Savic, J. Dudás, I. Kvitsaridze, S. Skvortsov, H. Riechelmann and I.-I. Skvortsova, 2020.
- K. Pantel and M. Speicher, Oncogene, 2016, 35, 1216–1224 CrossRef CAS PubMed.
- S. Anvari, E. Osei and N. Maftoon, Sci. Rep., 2021, 11, 15477 CrossRef CAS PubMed.
- M. Schlesinger, J. Hematol. Oncol., 2018, 11, 125 CrossRef CAS PubMed.
- M. B. Chen, J. A. Whisler, J. S. Jeon and R. D. Kamm, Integr. Biol., 2013, 5, 1262–1271 CrossRef CAS PubMed.
- A. Foss, L. Muñoz-Sagredo, J. Sleeman and W. Thiele, Clin. Exp. Metastasis, 2020, 37, 47–67 CrossRef PubMed.
- B. Strilic and S. Offermanns, Cancer Cell, 2017, 32, 282–293 CrossRef CAS PubMed.
- K. Stoletov, P. H. Beatty and J. D. Lewis, Expert Rev. Anticancer Ther., 2020, 20, 97–109 CrossRef CAS PubMed.
- J. M. K. Ganesh, Nat. Med., 2021, 27, 34–44 CrossRef PubMed.
- K. G. K. Deepak, R. Vempati, G. P. Nagaraju, V. R. Dasari, N. S. D. N. Rao and R. R. Malla, Pharmacol. Res., 2020, 153, 104683 CrossRef CAS PubMed.
- S. Kumari, D. Advani, S. Sharma, R. K. Ambasta and P. Kumar, Biochim. Biophys. Acta, Rev. Cancer, 2021, 1876, 188585 CrossRef CAS PubMed.
- M. E. Menyailo, U. A. Bokova, E. E. Ivanyuk, A. A. Khozyainova and E. V. Denisov, Mol. Diagn. Ther., 2021, 25, 549–562 CrossRef PubMed.
- H. Yang, Y. H. Kuo, Z. I. Smith and J. Spangler, Wiley Interdiscip. Rev.: Nanomed. Nanobiotechnol., 2021, 13, e1698 CAS.
- S. Harihar and D. R. Welch, Cancer Metastasis Rev., 2023, 42, 183–196 CrossRef CAS PubMed.
- Y. Yoshida, K. Yuki, S. Dan, K. Yamazaki and M. Noda, Sci. Rep., 2022, 12, 2319 CrossRef CAS PubMed.
- Y. Wang, Y. Ding, N. Guo and S. Wang, Front. Immunol., 2019, 10, 172 CrossRef CAS PubMed.
- A. M. Law, F. Valdes-Mora and D. Gallego-Ortega, Cells, 2020, 9, 561 CrossRef CAS PubMed.
- Z. Lu, J. Zou, S. Li, M. J. Topper, Y. Tao, H. Zhang, X. Jiao, W. Xie, X. Kong, M. Vaz, H. Li, Y. Cai, L. Xia, P. Huang, K. Rodgers, B. Lee, J. B. Riemer, C. P. Day, R. C. Yen, Y. Cui, Y. Wang, Y. Wang, W. Zhang, H. Easwaran, A. Hulbert, K. Kim, R. A. Juergens, S. C. Yang, R. J. Battafarano, E. L. Bush, S. R. Broderick, S. M. Cattaneo, J. R. Brahmer, C. M. Rudin, J. Wrangle, Y. Mei, Y. J. Kim, B. Zhang, K. K. Wang, P. M. Forde, J. B. Margolick, B. D. Nelkin, C. A. Zahnow, D. M. Pardoll, F. Housseau, S. B. Baylin, L. Shen and M. V. Brock, Nature, 2020, 579, 284–290 CrossRef CAS PubMed.
- N. Zhang, A. S. Ng, S. Cai, Q. Li, L. Yang and D. Kerr, Lancet Oncol., 2021, 22, e358–e368 CrossRef CAS PubMed.
- S. Jonckheere, J. Adams, D. De Groote, K. Campbell, G. Berx and S. Goossens, Cells Tissues Organs, 2022, 211, 157–182 CrossRef CAS PubMed.
- R. I. Teleanu, C. Chircov, A. M. Grumezescu and D. M. Teleanu, J. Clin. Med., 2019, 9, 84 CrossRef PubMed.
- Y. Jiang, H. Zhang, J. Wang, Y. Liu, T. Luo and H. Hua, J. Hematol. Oncol., 2022, 15, 34 CrossRef PubMed.
- Y. Zhao, X. Zheng, Y. Zheng, Y. Chen, W. Fei, F. Wang and C. Zheng, Front. Oncol., 2021, 11, 650453 CrossRef CAS PubMed.
- C. M. Neophytou, M. Panagi, T. Stylianopoulos and P. Papageorgis, Cancers, 2021, 13, 2053 CrossRef CAS PubMed.
- H. Dianat-Moghadam, A. Mahari, M. Heidarifard, N. Parnianfard, L. Pourmousavi-Kh, R. Rahbarghazi and Z. Amoozgar, Cancer Lett., 2021, 497, 41–53 CrossRef CAS PubMed.
- D. J. Smit, K. Pantel and M. Jücker, Biochem. Pharmacol., 2021, 188, 114589 CrossRef CAS PubMed.
- S. S. Das, S. Alkahtani, P. Bharadwaj, M. T. Ansari, M. D. ALKahtani, Z. Pang, M. S. Hasnain, A. K. Nayak and T. M. Aminabhavi, Int. J. Pharm., 2020, 585, 119556 CrossRef CAS PubMed.
- C. Roma-Rodrigues, R. Mendes, P. V. Baptista and A. R. Fernandes, Int. J. Mol. Sci., 2019, 20, 840 CrossRef CAS PubMed.
- K. Stoletov, P. H. Beatty and J. D. Lewis, Expert Rev. Anticancer Ther., 2020, 20, 97–109 CrossRef CAS PubMed.
- M. Wang, J. Chen, W. Li, F. Zang, X. Liu and S. Qin, Mater. Sci. Eng., C, 2020, 114, 111046 CrossRef CAS PubMed.
- X. Si, G. Ji, S. Ma, Y. Xu, J. Zhao, Y. Zhang, Z. Huang, Z. Tang, W. Song and X. Chen, Adv. Healthcare Mater., 2021, 10, e2100862 CrossRef PubMed.
- L. Zhang, J. Zhou, L. Hu, X. Han, X. Zou, Q. Chen, Y. Chen, Z. Liu and C. Wang, Adv. Funct. Mater., 2020, 30, 1906922 CrossRef CAS.
- H. Pan, C. Zhang, T. Wang, J. Chen and S.-K. Sun, ACS Appl. Mater. Interfaces, 2018, 11, 2782–2789 CrossRef PubMed.
- Y. Cao, Y. Zhou, Z. Chen, Z. Zhang, X. Chen and C. He, Adv. Healthcare Mater., 2021, 10, 2100814 CrossRef CAS PubMed.
- T.-J. Zhou, L. Xing, Y.-T. Fan, P.-F. Cui and H.-L. Jiang, J. Controlled Release, 2019, 309, 82–93 CrossRef CAS PubMed.
- X. Liu, Y. Huangfu, J. Wang, P. Kong, W. Tian, P. Liu, C. Fang, S. Li, Y. Nie and Z. Feng, Adv. Sci., 2023, 10, 2300637 CrossRef CAS PubMed.
- A. Chyzy, M. Tomczykowa and M. E. Plonska-Brzezinska, Materials, 2020, 13, 188 CrossRef CAS PubMed.
- C. A. Dreiss, Curr. Opin. Colloid Interface Sci., 2020, 48, 1–17 CrossRef CAS.
- Z. Sun, C. Song, C. Wang, Y. Hu and J. Wu, Mol. Pharmaceutics, 2019, 17, 373–391 Search PubMed.
- H. F. Darge, A. T. Andrgie, E. Y. Hanurry, Y. S. Birhan, T. W. Mekonnen, H.-Y. Chou, W.-H. Hsu, J.-Y. Lai, S.-Y. Lin and H.-C. Tsai, Int. J. Pharm., 2019, 572, 118799 CrossRef CAS PubMed.
- A. Onaciu, R. A. Munteanu, A. I. Moldovan, C. S. Moldovan and I. Berindan-Neagoe, Pharmaceutics, 2019, 11, 432 CrossRef CAS PubMed.
- B. Tan, L. Huang, Y. Wu and J. Liao, J. Biomed. Mater. Res. Part A, 2021, 109, 404–425 CrossRef CAS PubMed.
- A. Pandya, L. Vora, C. Umeyor, D. Surve, A. Patel, S. Biswas, K. Patel and V. Patravale, Adv. Drug Delivery Rev., 2023, 115003 CrossRef CAS PubMed.
- R. N. Woodring, E. G. Gurysh, E. M. Bachelder and K. M. Ainslie, ACS Appl. Bio Mater., 2023, 6, 934–950 CrossRef CAS PubMed.
- Z. Yu, Z. Xiao, X. Shuai and J. Tian, J. Mater. Chem. B, 2020, 8, 6418–6428 RSC.
- Y. Hu, X. Chen, Z. Li, S. Zheng and Y. Cheng, J. Biomed. Nanotechnol., 2020, 16, 54–64 CrossRef CAS PubMed.
- D. Yang, J. Ning, X. Liao, H. Jiang and S. Qin, Int. J. Nanomed., 2023, 18, 3989–4005 CrossRef CAS PubMed.
- C. Maiti, K. B. C. Imani and J. Yoon, ChemPlusChem, 2021, 86, 601–611 CrossRef CAS PubMed.
- F. Rizzo and N. S. Kehr, Adv. Healthcare Mater., 2021, 10, 2001341 CrossRef CAS PubMed.
- D. Wei, Y. Sun, H. Zhu and Q. Fu, ACS Nano, 2023, 17, 23223–23261 CrossRef CAS PubMed.
- Z. Li, W. Xu, J. Yang, J. Wang, J. Wang, G. Zhu, D. Li, J. Ding and T. Sun, Adv. Mater., 2022, 34, 2200449 CrossRef CAS PubMed.
- A. Alexander, J. Khan, S. Saraf and S. Saraf, Eur. J. Pharm. Biopharm., 2014, 88, 575–585 CrossRef CAS PubMed.
- L. Picton, M. Morel, M. Madau, L. Didier, V. Dulong, G. Anne-Claire and A. Malzert-Freon, J. Mater. Chem. B, 2024, 12, 2807–2817 RSC.
- M. Karimi, P. Sahandi Zangabad, A. Ghasemi, M. Amiri, M. Bahrami, H. Malekzad, H. Ghahramanzadeh Asl, Z. Mahdieh, M. Bozorgomid and A. Ghasemi, ACS Appl. Mater. Interfaces, 2016, 8, 21107–21133 CrossRef CAS PubMed.
- S. Zhao, M. Cao, H. Li, L. Li and W. Xu, Carbohydr. Res., 2010, 345, 425–431 CrossRef CAS PubMed.
- C. Alvarez-Lorenzo, V. Y. Grinberg, T. V. Burova and A. Concheiro, Int. J. Pharm., 2020, 579, 119157 CrossRef CAS PubMed.
- B. Tian, S. Hua, Y. Tian and J. Liu, J. Mater. Chem. B, 2020, 8, 10050–10064 RSC.
- B. Zhuang, T. Chen, Z. Xiao and Y. Jin, Int. J. Pharm., 2020, 577, 119048 CrossRef CAS PubMed.
- Y. Sun, D. Nan, H. Jin and X. Qu, Polym. Test., 2020, 81, 106283 CrossRef CAS.
- S. Yu, C. He and X. Chen, Macromol. Biosci., 2018, 18, 1800240 CrossRef PubMed.
- D. Y. Kwon, J. S. Kwon, J. H. Park, S. H. Park, H. J. Oh, J. H. Kim, B. H. Min, K. Park and M. S. Kim, Biomaterials, 2016, 85, 232–245 CrossRef PubMed.
- X. Wang, J. Gao, C. Li, C. Xu, X. Li, F. Meng, Q. Liu, Q. Wang, L. Yu and B. Liu, Mater. Today Bio, 2022, 15, 100305 CrossRef CAS PubMed.
- Q. Leng, Y. Li, P. Zhou, K. Xiong, Y. Lu, Y. Cui, B. Wang, Z. Wu, L. Zhao and S. Fu, Mater. Sci. Eng., C, 2021, 129, 112390 CrossRef CAS PubMed.
- H. Wang, Y. Jin, Y. Tan, H. Zhu, W. Huo, P. Niu, Z. Li, J. Zhang, X. J. Liang and X. Yang, Biomaterials, 2021, 275, 120992 CrossRef CAS PubMed.
- F. Li, X. Shao, D. Liu, X. Jiao, X. Yang, W. Yang and X. Liu, Pharmaceutics, 2022, 14, 1809 CrossRef CAS PubMed.
- S. De Dosso, A. R. Siebenhüner, T. Winder, A. Meisel, R. Fritsch, C. Astaras, P. Szturz and M. Borner, Cancer Treat. Rev., 2021, 96, 102180 CrossRef CAS PubMed.
- S. Das and S. K. Batra, Curr. Pharm. Des., 2015, 21, 1249–1255 CrossRef CAS PubMed.
- H. Braet, P.-P. Fransen, R. Mariën, G. Lollo, W. Ceelen, C. Vervaet, L. Balcaen, F. Vanhaecke, C. Vanhove and S. van der Vegte, ACS Appl. Mater. Interfaces, 2023, 15, 49022–49034 CrossRef CAS PubMed.
- J. Li, Q. Chen, S. Li, X. Zeng, J. Qin, X. Li, Z. Chen, W. Zheng, Y. Zhao and Z. Huang, Chem. Eng. J., 2023, 473, 145212 CrossRef CAS.
- B. Zhuang, T. Chen, Z. Xiao and Y. Jin, Int. J. Pharm., 2020, 577, 119048 CrossRef CAS PubMed.
- J. Zhang, Y. Guo, G. Pan, P. Wang, Y. Li, X. Zhu and C. Zhang, ACS Appl. Mater. Interfaces, 2020, 12, 21441–21449 CrossRef CAS PubMed.
- R. Fan, W. Sun, T. Zhang, R. Wang, Y. Tian, H. Zhang, J. Li, A. Zheng and S. Song, Biomed. Pharmacother., 2022, 150, 113017 CrossRef CAS PubMed.
- W. Chen, K. Shi, J. Liu, P. Yang, R. Han, M. Pan, L. Yuan, C. Fang, Y. Yu and Z. Qian, Bioact. Mater., 2023, 23, 1–15 CAS.
- A. T. Andrgie, S. L. Mekuria, K. D. Addisu, B. Z. Hailemeskel, W. H. Hsu, H. C. Tsai and J. Y. Lai, Macromol. Biosci., 2019, 19, 1800409 CrossRef PubMed.
- L. Meng, Z. Wang, Z. Hou, H. Wang, X. Zhang, X. Zhang, X. He, X. Zhang, B. Qin, J. Li, Z. Zhang, X. Xue and Y. Wei, Front. Immunol., 2022, 13, 1064047 CrossRef CAS PubMed.
- Y. Fang, S. Huang, Q. Hu, J. Zhang, J. A. King, Y. Wang, Z. Wei, J. Lu, Z. He, X. Kong, X. Yang, J. Ji, J. Li, G. Zhai and L. Ye, ACS Nano, 2023, 17(24), 24883–24900 CrossRef CAS PubMed.
- E. Ren, Y. Wang, T. Liang, H. Zheng, J. Shi, Z. Cheng, H. Li and Z. Gu, Small Methods, 2023, 2300347 CrossRef CAS PubMed.
- J. Sun, F. Xing, J. Braun, F. Traub, P. M. Rommens, Z. Xiang and U. Ritz, Int. J. Mol. Sci., 2021, 22, 11354 CrossRef CAS PubMed.
- J. Yang, Q. Fu, H. Jiang, Y. Li and M. Liu, Front. Oncol., 2022, 12, 1022973 CrossRef CAS PubMed.
- S. Zhou, Z. Wang, Y. Wang and L. Feng, ACS Appl. Bio Mater., 2020, 3, 1730–1737 CrossRef CAS PubMed.
- S. Gan, Y. Wu, X. Zhang, Z. Zheng, M. Zhang, L. Long, J. Liao and W. Chen, Gels, 2023, 9, 286 CrossRef CAS PubMed.
- J. Zeng, D. Shi, Y. Gu, T. Kaneko, L. Zhang, H. Zhang, D. Kaneko and M. Chen, Biomacromolecules, 2019, 20, 3375–3384 CrossRef CAS PubMed.
- S. Wang, F. Wang, X. Zhao, F. Yang, Y. Xu, F. Yan, D. Xia and Y. Liu, Mater. Des., 2022, 217, 110621 CrossRef CAS.
- X. Jin, S. Yao, F. Qiu, Z. Mao and B. Wang, Colloids Surf., A, 2021, 614, 126154 CrossRef CAS.
- J. Yao, C. Zhu, T. Peng, Q. Ma and S. Gao, Front. Bioeng. Biotechnol., 2021, 9, 791891 CrossRef PubMed.
- L. Zhang, M. Yang, Y. Ji, K. Xiao, J. Shi and L. Wang, Biomater. Sci., 2021, 9, 2124–2136 RSC.
- J. Wang, W. Xu, J. Qian, Y. Wang, G. Hou, A. Suo and Y. Ma, J. Colloid Interface Sci., 2022, 628, 79–94 CrossRef CAS PubMed.
- M. Abbott and Y. Ustoyev, Semin. Oncol. Nurs., 2019, 35, 150923 CrossRef PubMed.
- J. H. Cha, L. C. Chan, M. S. Song and M. C. Hung, Cold Spring Harbor Perspect. Med., 2020, 10, a036863 CrossRef CAS PubMed.
- S. C. Edwards, W. H. M. Hoevenaar and S. B. Coffelt, Br. J. Cancer, 2021, 124, 37–48 CrossRef PubMed.
- L. Chen and A. E. Musa, Phytother. Res., 2021, 35, 5514–5526 CrossRef CAS PubMed.
- S. L. Gupta, S. Basu, V. Soni and R. K. Jaiswal, Mol. Biol. Rep., 2022, 49, 9903–9913 CrossRef CAS PubMed.
- J. Kleponis, R. Skelton and L. Zheng, Cancer Biol. Med., 2015, 12, 201–208 CAS.
- S. Farkona, E. P. Diamandis and I. M. Blasutig, BMC Med., 2016, 14, 1–18 CrossRef PubMed.
- Y. Zhang and Z. Zhang, Cell. Mol. Immunol., 2020, 17, 807–821 CrossRef CAS PubMed.
- P. Abdou, Z. Wang, Q. Chen, A. Chan, D. R. Zhou, V. Gunadhi and Z. Gu, Wiley Interdiscip. Rev.: Nanomed. Nanobiotechnol., 2020, 12, e1632 Search PubMed.
- D. Singh, M. A. Khan and H. R. Siddique, Biochem. Pharmacol., 2022, 198, 114955 CrossRef CAS PubMed.
- T. Fang, X. Cao, L. Wang, M. Chen, Y. Deng and G. Chen, Bioact. Mater., 2024, 32, 530–542 CAS.
- S. Han, W. Wang, S. Wang, T. Yang, G. Zhang, D. Wang, R. Ju, Y. Lu, H. Wang and L. Wang, Theranostics, 2021, 11, 2892–2916 CrossRef CAS PubMed.
- C. Gao, K. Cheng, Y. Li, R. Gong, X. Zhao, G. Nie and H. Ren, Nano Lett., 2022, 22, 8801–8809 CrossRef CAS PubMed.
- A. Kichloo, M. Albosta, D. Dahiya, J. C. Guidi, M. Aljadah, J. Singh, H. Shaka, F. Wani, A. Kumar and M. Lekkala, World J. Clin. Oncol., 2021, 12, 150–163 CrossRef PubMed.
- Z. Xie, J. Shen, H. Sun, J. Li and X. Wang, Biomed. Pharmacother., 2021, 137, 111333 CrossRef CAS PubMed.
- X. Zhang, X. Guo, Y. Wu and J. Gao, Gels, 2021, 7, 224 CrossRef CAS PubMed.
- X. Han, H. Li, D. Zhou, Z. Chen and Z. Gu, Acc. Chem. Res., 2020, 53, 2521–2533 CrossRef CAS PubMed.
- H. Song, P. Yang, P. Huang, C. Zhang, D. Kong and W. Wang, Theranostics, 2019, 9, 2299–2314 CrossRef CAS PubMed.
- M. Liu, Z. Cao, R. Zhang, Y. Chen and X. Yang, ACS Appl. Mater. Interfaces, 2021, 13, 33874–33884 CrossRef CAS PubMed.
- J. Zhai, X. Gu, Y. Liu, Y. Hu, Y. Jiang and Z. Zhang, Front. Pharmacol., 2023, 14, 1152934 CrossRef CAS PubMed.
- D. Xie, Q. Wang and G. Wu, Front. Immunol., 2022, 13, 1017400 CrossRef CAS PubMed.
- M. Wang, D. Hu, Y. Yang, K. Shi, J. Li, Q. Liu, Y. Li, R. Li, M. Pan, D. Mo, W. Chen, X. Li and Z. Qian, Adv. Sci., 2023, e2303819, DOI:10.1002/advs.202303819.
- J. Kim, Y. Choi, D.-H. Kim, H. Y. Yoon and K. Kim, Pharmaceutics, 2022, 14, 1908 CrossRef CAS PubMed.
- M. Al-Haideri, S. B. Tondok, S. H. Safa, A. H. Maleki, S. Rostami, A. T. Jalil, M. E. Al-Gazally, F. Alsaikhan, J. A. Rizaev and T. A. M. Mohammad, Cancer Cell Int., 2022, 22, 365 CrossRef PubMed.
- J. Chen, X. Zhang, J. Zhang, Z. Wang, G. Zhu, M. Geng, J. Zhu, Y. Chen, W. Wang and Y. Xu, J. Controlled Release, 2024, 365, 74–88 CrossRef CAS PubMed.
- T. Xiao, J. Zhu, L. Jia, H. Che, J. Liu, J. Deckers, J. C. van Hest and X. Shi, J. Controlled Release, 2022, 348, 239–249 CrossRef CAS PubMed.
- P. Sun, T. Huang, X. Wang, G. Wang, Z. Liu, G. Chen and Q. Fan, Biomacromolecules, 2019, 21, 556–565 CrossRef PubMed.
- P. Hu, W. Wang, J. Sha, Y. Xing, Y. Wang, C. Wu, J. Li, K. Gao, H. Dong and S. Zheng, Mater. Des., 2023, 225, 111429 CrossRef CAS.
- B. Zhuang, T. Chen, Y. Huang, Z. Xiao and Y. Jin, Acta Pharm. Sin. B, 2022, 12, 1447–1459 CrossRef CAS PubMed.
- Y. Lu, C. Wu, Y. Yang, X. Chen, F. Ge, J. Wang and J. Deng, Biomater. Sci., 2022, 10, 1352–1363 RSC.
- X. Wang, L. Zhu, J. Zhou, L. Zhao, J. Li and C. Liu, Front. Bioeng. Biotechnol., 2023, 11, 1281157 CrossRef PubMed.
- M. Zhou, Q. Zuo, Y. Huang and L. Li, Acta Pharm. Sin. B, 2022, 12, 3383–3397 CrossRef CAS PubMed.
- S.-X. Chen, J. Zhang, F. Xue, W. Liu, Y. Kuang, B. Gu, S. Song and H. Chen, Bioact. Mater., 2023, 21, 86–96 CAS.
- W. J. Yang, P. Zhou, L. Liang, Y. Cao, J. Qiao, X. Li, Z. Teng and L. Wang, ACS Appl. Mater. Interfaces, 2018, 10, 18560–18573 CrossRef CAS PubMed.
- Q. Zhao, X. Yue, L. Miaomiao, W. Yanming and G. Wu, J. Biomater. Appl., 2023, 38, 614–628 CrossRef CAS PubMed.
- C. Jaiswal, T. Gupta, P. K. Jadi, J. C. Moses and B. B. Mandal, Biomater. Adv., 2023, 145, 213224 CrossRef CAS PubMed.
- N. Liu, S. Wu, X. Tian and X. Li, J. Mater. Chem. B, 2022, 10, 5165–5173 RSC.
- A. Luca, I. Nacu, S. Tanasache, C. A. Peptu, M. Butnaru and L. Verestiuc, Gels, 2023, 9, 371 CrossRef CAS PubMed.
- C. Li, Y. Liu, D. Li, Q. Wang, S. Zhou, H. Zhang, Y. Wang, Z. He, H. Liu and J. Sun, Theranostics, 2022, 12, 4581–4598 CrossRef CAS PubMed.
- X. Zhou, X. He, K. Shi, L. Yuan, Y. Yang, Q. Liu, Y. Ming, C. Yi and Z. Qian, Adv. Sci., 2020, 7, 2001442 CrossRef CAS PubMed.
- T. Wang, J. Ding, Z. Chen, Z. Zhang, Y. Rong, G. Li, C. He and X. Chen, ACS Appl. Mater. Interfaces, 2024, 16, 9868–9879 CrossRef CAS PubMed.
- Y. J. Lu, Y. H. Lan, C. C. Chuang, W. T. Lu, L. Y. Chan, P. W. Hsu and J. P. Chen, Int. J. Mol. Sci., 2020, 21, 7111 CrossRef CAS PubMed.
- N. Wang, Q. Gao, J. Tang, Y. Jiang, L. Yang, X. Shi, Y. Chen, Y. Zhang, S. Fu and S. Lin, Drug Delivery, 2021, 28, 183–194 CrossRef CAS PubMed.
- A. M. Shabana, S. P. Kambhampati, R. C. Hsia, R. M. Kannan and E. Kokkoli, Int. J. Pharm., 2021, 593, 120139 CrossRef CAS PubMed.
|
This journal is © The Royal Society of Chemistry 2024 |