DOI:
10.1039/D3BM01838E
(Minireview)
Biomater. Sci., 2024,
12, 108-115
Single atom nanozymes for bacterial infection therapy
Received
10th November 2023
, Accepted 20th November 2023
First published on 22nd November 2023
Abstract
Bacterial infection-related diseases continue to pose a significant challenge to global human health. Antibiotic therapy, as a conventional therapeutic strategy, has been extensively employed in clinical settings to treat bacterial infections. However, the effectiveness of these conventional strategies is often impeded by the antimicrobial resistance of bacteria. Consequently, the development of alternative antibacterial agents has emerged as a promising approach to addressing this issue. In recent years, single-atom nanozymes (SAzymes), a novel class of nanocatalytic medicines, have garnered increasing attention due to their numerous advantages, including uniformly dispersed metal active sites, tunable coordination structures, and maximal metal atomic utilization efficiency. To date, a variety of SAzymes have been developed and widely applied in antibacterial therapy. In this minireview, we provide an overview of the latest advances in the synthesis and antibacterial application of different metal-based SAzymes. Furthermore, we discuss the future challenges and opportunities of utilizing SAzymes for bacterial infection treatment. It is our hope that this minireview will contribute to the development of the next generation of SAzyme-based antibacterial agents.
1. Introduction
Bacterial infection-related diseases are still among the most serious health problems worldwide.1 Currently, antibiotic therapy is still the main method to treat bacterial infections. However, the overuse and misuse of antibiotics have caused the emergence of multi-resistant bacteria, which significantly impedes the treatment outcomes of bacterial infections.2,3 Developing alternative antimicrobial agents has been regarded as a promising way to avoid bacterial resistance.
Nanozymes, a kind of nanomaterial, have the capability to mimic the structure, properties, and biological functions of natural enzymes.4,5 As early as 2007, Yan and her co-workers reported that Fe3O4 nanoparticles can mimic peroxidase (POD) to catalyze the decomposition reaction of H2O2 to produce hydroxyl radicals (˙OH).6 Since then, a wide range of nanomaterials, including metal oxides,7 metal sulfides,8 metal organic frameworks (MOFs),9 metal nanoparticles10etc., have been extensively developed to mimic natural enzymes. Recently, nanozymes have been widely used in the fields of catalysis, environmental remediation, food safety, biosensing, and biomedicine due to their inherent advantages over natural enzymes, such as low cost, high stability, and easy storage.5,11 Although studies on nanozymes have made considerable achievements in the past few decades, the catalytic activity of nanozymes is still far below that of natural enzymes.12
The catalytic activity of nanozymes is widely acknowledged to be highly dependent on the density of metal active sites.13 Numerous studies have demonstrated that decreasing the size of metal nanoparticles to the single-atom level is an effective strategy for enhancing the catalytically active site of catalysts. In 2011, Zhang et al.14 first described single-atom catalysts (SACs) with atomically dispersed metal sites on supports. Due to the benefits of uniformly distributed catalytically active sites and nearly 100% atomic utilization,15–17 SACs have been extensively investigated and used in the fields of energy catalysis, environmental remediation, and biomedicine.
Drawing inspiration from the M–Nx sites of natural metalloproteinases, researchers have developed a vast array of carbon-based SACs with M–N–C structures (M = Fe, Co, Cu, Zn, Mn, etc.). These SACs have been widely used to mimic the catalytic function of natural enzymes.18,19 Owing to the adjustable coordination environment and electronic structure of the metal site, single-atom nanozymes (SAzymes) exhibit a diverse range of enzyme-like catalytic activities, including peroxidase (POD), oxidase (OXD), catalase (CAT), superoxide dismutase (SOD), and glutathione peroxidase (GPx).20 Thus far, SAzymes have been successfully applied in the treatment of cancer, bacterial infections, and oxidative stress-related diseases.21,22 Although several outstanding reviews have summarized the development of SAzymes in the field of biomedicine,23,24 no specific review pertaining to antibacterial treatment has been reported.
Herein, we focus on the application of SAzymes in antimicrobial treatment and summarize the latest advancements in the development of SAzyme-based antimicrobial agents. In recent years, various SAzymes incorporating different metals, including Pt, Ir, Ru, Fe, Cu, Zn, Co, and Mn, have been synthesized and employed as antibacterial agents to treat bacterial infections (Scheme 1). Compared with other therapeutic approaches, catalytic therapy against bacteria using SAzymes exhibits several advantages: (1) the generated reactive oxygen species (ROS, including ˙OH and O2˙−) possess strong oxidative capacity and can easily disrupt biofilms and induce bacterial death due to oxidative damage. (2) Utilizing SAzyme-based antimicrobial agents can evade the emergence of multi-resistant bacteria caused by antibiotics. (3) In contrast to antibiotic therapy, SAzyme-based catalytic therapy demonstrates superior broad-spectrum antimicrobial activity.1,2 We hope that this minireview will stimulate further advancements in SAzyme-based catalytic therapy against bacterial infections.
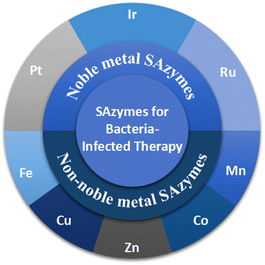 |
| Scheme 1 Schematic illustration of SAzyme antibacterials based on various metals. | |
2. Noble metal single atom nanozymes
Noble metal-based nanomaterials, including Au, Ag, Pt and Pd, etc., have been widely used in the treatment of bacterial infections through physical, mechanical or chemical damage strategies.25 Among these strategies, ROS-induced oxidative stress is typically considered the most effective chemical damage method against bacteria. More recently, noble metal SAzymes with isolated metal active sites have gained increasing attention due to their excellent antibacterial activity compared to noble nanoparticles. Extensive research has demonstrated that SAzymes’ superior bacteriostatic activity is attributed to their abundance of coordinatively unsaturated metal sites. These high catalytic activity sites can effectively convert O2 or H2O2 to highly toxic O2˙− or ˙OH, which can subsequently destroy the biofilm of bacteria and kill them through oxidative damage.
2.1. Pt-based single atom nanozymes
The strong interaction between metal single atoms and supports is the determining factor of the catalytic activity of SAzymes. In 2021, Chen et al.26 synthesized a Pt-based SAzyme using a high-temperature thermal atomization strategy. Pt nanoparticles were atomized into single atoms and anchored on N-doped carbon derived from zeolitic imidazolate frameworks (ZIF-8). This reversing thermal sintering method not only improved the interaction between Pt single atoms and N-doped carbon, but also increased the exposure of the catalytic sites. Owing to the synergistic effects of Pt, N, P, and S atoms, PtTS–SAzyme exhibited excellent antibacterial effects against many bacteria, including E. coli, P. aeruginosa, S. enteritidis, K. pneumoniae, and S. aureus. Methicillin-resistant Staphylococcus aureus (MRSA) induced infection is the main reason for bone destruction at the osteomyelitis site.
In order to enhance the treatment efficacy of MRSA-infected osteomyelitis, Yu et al.28 developed a multifunctional single atom catalyst RBC–HNTM–Pt@Au, composed of a Pt single atom-doped porphyrin metal–organic framework (MOF, named HMTM), gold nanorods and an erythrocyte membrane (RBC). Pt single atoms and Au nanorods can synergistically promote the transfer of ultrasonic excited electrons, which could further enhance the sonodynamic therapy (SDT) performance of HNTM–Pt@Au. Moreover, the RBC coating enabled HNTM–Pt@Au to gain excellent biocompatibility and toxin neutralization ability. They revealed that RBC–HNTM–Pt@Au can kill 99.9% of MRSA within 15 min under ultrasonic irradiation. In vivo experimental results demonstrated that RBC–HNTM–Pt@Au possesses strong SDT and dynamic detoxification abilities for treating MRSA-infected osteomyelitis and preventing bone destruction. More recently, they reported another Pt-based SAzyme composed of PEG-400/PEG-4000 and a Pt single-atom doped porphyrin MOF (PCN-222-Pt).27 The prepared injectable ointment could be used to treat periodontitis owing to its potent OXD-like and POD-like activities. In vitro and in vivo results demonstrated that PCN-222-Pt SAzymes can successfully alleviate inflammation and reduce bone loss in biofilm-induced periodontitis (Fig. 1).
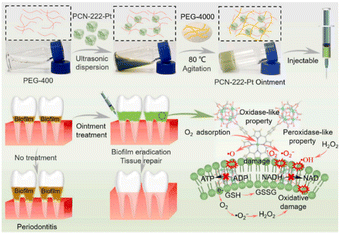 |
| Fig. 1 Preparation of the injectable HNTM–Pt ointment and the treatment of periodontitis through the catalysis systems’ activities.27 Copyright 2022, Elsevier. | |
Two-dimensional nanomaterials, such as g-C3N4, graphene, MXenes, etc., are widely used as carriers for constructing SAzymes due to their excellent electron transport capacity. In 2022, Fan et al.29 embedded single atom Pt into carbon nitride nanorods (g-C3N4) to create a Pt-based SAzyme (SA–Pt/g-C3N4–K). The prepared SA–Pt/g-C3N4–K, which functions as a single-atom peroxidase, exhibits dual capabilities of bacterial eradication and detection of antibiotic residues. Additionally, two-dimensional metal carbides and nitrides (2D MXene) can serve as effective supports for constructing single atom catalysts by forming a strong metal–carbon bond between the single atom and MXene. More recently, Li et al.30 have constructed a Ti3C2-based nanozyme by anchoring a Pt single atom on Ti3C2 MXene (Pt–Ti3C2). Experimental results and theoretical calculations demonstrated that the introduction of the Pt single atom significantly enhances the photothermal and POD-like activities of Pt–Ti3C2. Moreover, owing to its photothermally-amplified nanozyme catalysis effect, Pt–Ti3C2 exhibits remarkable efficacy in eliminating S. aureus in deep-seated infections and accelerating abscess resolution in deep-seated subcutaneous tissue (Fig. 2).
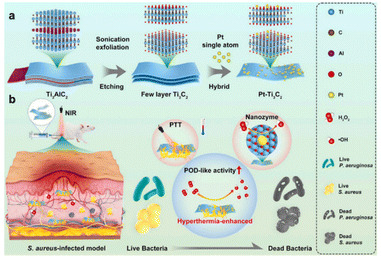 |
| Fig. 2 Illustration of the preparation procedure (a) and the antibacterial mechanism (b) of Pt–Ti3C2.30 Copyright 2023, Elsevier. | |
2.2. Other noble single atom nanozymes
Ferroptosis-like cell death has emerged as a promising strategy for antibacterial therapy. Studies have shown that delivering metallic iron into infected tissue can induce a ferroptosis-like response in pathogenic bacteria.31–33 However, the therapeutic efficiency based on iron-based nanomaterials is currently unsatisfactory. More recently, Sun et al.34 have developed two types of SAzymes by anchoring single atoms of Ir and Ru onto sp2-carbon-linked covalent organic frameworks (Ir SACs and Ru SACs). The introduction of Ir and Ru metal sites significantly reduced the bandgap of the COFs, promoting the production of 1O2 and O2˙− under the irradiation of red light. More importantly, the synergistic effects of highly dispersed metal sites and sp2c-COF hosts endowed Ir SACs and Ru SACs with excellent POD-like activity. Leveraging their POD-like performance and photocatalytic activity, both Ir SACs and Ru SACs demonstrated remarkable antibacterial efficiency against Gram-positive bacteria, Gram-negative bacteria, and MRSA. The authors proposed an antibacterial mechanism for Ir SACs and Ru SACs, as illustrated in Fig. 3. It was observed that both Ir SACs and Ru SACs induced ferroptosis-like responses in bacteria by damaging fatty acids or peptidoglycan, disrupting the antioxidant system, impairing nitrogen and respiratory metabolisms, and hindering DNA biosynthesis.
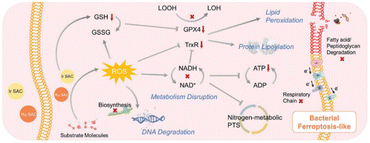 |
| Fig. 3 Mechanism of bacterial ferroptosis-like responses induced by Ir SACs and Ru SACs.34 Copyright 2023, Wiley. | |
3. Non-noble metal single atom nanozymes
Although noble metal SAzymes have demonstrated promising bacterial inactivation properties, their high cost remains a bottleneck for practical applications. To overcome this obstacle, the development of non-noble metal SAzymes has become a burgeoning focus in the field of bacteria-infected therapy. Recent research efforts have been directed towards synthesizing non-noble metal SAzymes, including Fe, Cu, Zn, Co, and Mn, which have been explored for the treatment of various bacteria-infected diseases.35 The use of non-noble metals in SAzymes offers several advantages, such as their similar structure and properties to natural enzymes, low cost, and ease of synthesis, making them practical alternatives to noble metals.
3.1. Fe-based single atom nanozymes
Iron-based SAzymes are among the most extensively investigated single atom nanozymes, mainly due to their resemblance to the iron–porphyrin structure found in natural horseradish peroxidase.36 In 2019, Huo et al.37 developed Fe-based SAzymes, termed SAF NCs, by anchoring single atoms of iron into nitrogen-doped carbon using an “encapsulated-pyrolysis” strategy. Acting as peroxidase mimics, SAF NCs can effectively catalyze the decomposition of H2O2 to generate abundant ˙OH, enabling them to eradicate E. coli and S. aureus pathogens, as confirmed by in vitro and in vivo antibacterial assays. Similarly, in the same year, Huang et al.38 discovered that regulation of the coordination environment of single atom Fe could markedly enhance its OXD-like activity. They constructed a five-coordinated single atom Fe nanozyme (FeN5 SA/CNF) by introducing an axial coordinated N atom via a bottom-up strategy. Compared to SAzymes with FeN4 structures, FeN5 SA/CNF with a confined FeN5 active center exhibited superior OXD-like catalytic activity. Experimental and theoretical analyses unveiled that the exceptional bactericidal properties of FeN5 SA/CNF arise from the electron push effect of the axial coordinated nitrogen atom and the synergistic effects of the Fe atom and the axial nitrogen-coordinated structure.
As a physical and metabolic barrier, biofilm can protect bacteria from the attack of the immune system and antibiotics.39 Meanwhile, the inherent features of the biofilm microenvironment (BME), including hypoxia, lower pH, a higher level of H2O2etc., also provide opportunities for targeted therapies towards bacteria.40 In 2021, Xu et al.41 reported nanofusiform-mediated Fe-based SAzymes (FePN SAzymes) with BME-activated performance. The high concentration of H2O2 in the BME triggered and enhanced the synergistic effect of photothermal therapy (PTT) and chemodynamic therapy (CDT). The results of DFT calculations revealed that the outstanding therapeutic effects of FePN SAzymes against E. coli and S. aureus are attributed to the high density of single-atom Fe sites. It is well known that the mass transfer rate is one of the key factors determining the catalytic activity of nanocatalysts. Therefore, enhancing the mass transfer rate would benefit increasing the catalytic activity of SAzymes. In 2022, Feng et al.42 constructed a spherical mesoporous Fe–N–C SAzyme with 4 nm pore size. The prepared Fe–N–C SAzyme exhibited high POD-like activity and photothermal conversion efficiency benefiting from its mesoporous structure. Owing to the generated ROS and photothermal effect, the spherical mesoporous Fe–N–C SAzyme can effectively avoid bacterial infection and increase wound healing in vivo (Fig. 4). Recently, Yang et al.43 have introduced boric acid group-functionalized carboxylated chitosan (CCS–PBA) into a single atom Fe catalyst to obtain a modified SAzyme Fe–SACs@CCS–PBA. They demonstrated that the CCS–PBA coating can significantly enhance the interactions between Fe–SACs and Gram-positive bacteria. Bactericidal experiments in vitro and in vivo demonstrated that Fe–SACs@CCS–PBA can effectively eradicate multidrug-resistant bacterial strains and also promote wound healing.
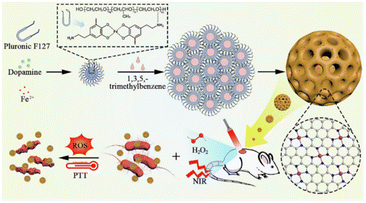 |
| Fig. 4 Schematic illustration of the synthesis and antibacterial application of spherical mesoporous Fe–N–C SAzymes.42 Copyright 2022, Elsevier. | |
3.2. Cu-based single atom nanozymes
It has been reported that the catalytic activity of cuprous ions in Fenton-like reactions can be more efficient or exhibit a higher reaction rate than that of iron ions in traditional Fenton reactions under comparable conditions.44 More recently, some Cu-based SAzymes have been developed and used for treating bacteria-infected wounds. In 2021, Wang et al.45 constructed a Cu-based SAzyme (Cu SASs/NPC) by anchoring single atom Cu into N-doped porous carbon derived from ZIF-8. Compared to N-doped porous carbon alone, Cu SASs/NPC exhibited higher POD-like activity, PTT efficiency, and glutathione (GSH)-depleting function. The antibacterial effects of Cu SASs/NPC against E. coli and MRSA in vitro and in vivo were carefully investigated. According to the results, Cu SASs/NPC showed nearly 100% antibacterial efficiency. For treating infectious disease induced by multiple pathogenic bacteria, Zhao et al.46 developed a Cu single atom catalyst (Cu SAC) with excellent OXD-like activity. The emulsion-template method ensured that Cu SAC obtained an ultra-large pore size (23.80 nm) and a small particle size (97.71 nm). The unique structure of Cu SAC is beneficial for exposing the Cu catalytic site and accelerating mass transfer. Therefore, Cu SAC could smoothly transform O2 into more toxic O2˙− and further cause oxidative stress in cells. They demonstrated that the inhibition rate of Cu SAC against four different pathogenic bacteria in vitro and in vivo could all reach up to 99.75%. Recently, Zhu et al.47 have reported a Cu SAzyme (Cu–N–C) with OXD and POD-like multienzyme activities. The O2˙− and ˙OH produced by Cu–N–C could destroy the membrane structure of the biofilm and further kill bacteria. Compared to vancomycin, cephalexin and gentamicin, Cu–N–C exhibited a superior sterilizing effect against MRSA (Fig. 5). Additionally, for treating wounds infected with multi-drug-resistant (MDR) bacteria, Qiu et al.48 developed a multifunctional Cu-based SAzyme therapeutic platform (L-Arg@Cu–SAzymes). Owing to their excellent catalase (CAT)-like and OXD-like activities, Cu–SAzymes can convert H2O2 to highly toxic O2˙−via dual enzyme cascade catalysis effects. Furthermore, H2O2 can oxidize L-Arg to produce NO. On one hand, NO would be converted into the peroxynitrite group (ONOO−) by O2˙−. On the other hand, NO can also improve wound inflammation and boost collagen synthesis and angiogenesis. L-Arg@Cu–SAzymes exhibited excellent inhibition efficacy against MDR bacteria and aided wound healing.
 |
| Fig. 5 Schematic illustration of the synthesis process and antibacterial effect of Cu–N–C SAzymes (a) and the diagram of wound treatment (b) with Cu–N–C SAzymes.47 Copyright 2022, ACS. | |
The above results proved that Cu-based SAzymes could be used as a potential candidate to combat a range of bacteria infected diseases. However, the slower diffusion rate of substrates in deep infected tissue significantly inhibits the generation of ROS catalyzed by Cu-based SAzymes.49 Enhancing the catalytic activity of Cu-based SAzymes is a promising way to meet this challenge. Previous studies have demonstrated that the catalytic activity of a Cu-based single atom catalyst depends on the N coordination number of the Cu atom.50 More recently, Bai et al.51 developed Cu-based SAzymes with an adjustable N coordination number by pyrolyzing Cu–silk fibroin complexes. The authors found that CuNx–CNS SAzymes with a CuN4 structure exhibited superior multienzyme activity (POD-, OXD- and CAT-like) than those with a CuN2 structure. Subsequently, they investigated the antibacterial activity of CuNx–CNS SAzymes in vitro and in vivo. All the results revealed that CuN4–CNS SAzymes have better therapeutic effects against bacterial infections in either superficial or deep tissues (Fig. 6).
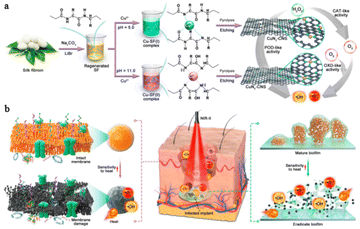 |
| Fig. 6 Illustration of the synthesis procedure (a) and the antibacterial therapy mechanism (b) of CuNx–CNS SAzymes.51 Copyright 2023, Research. | |
The common supports for constructing Cu-based SAzymes are carbon-based materials, including N-doped carbon, MOF-derived carbon, and carbon nitride. Replacing carbon-based supports with protein-based materials might be a promising strategy for enhancing the biodegradability of SAzymes. Recently, Wang et al.52 have constructed a BSA-based Cu SAzyme (BSA–Cu SAN) to eliminate the intratumor Fusobacterium (F.) nucleatum and synchronously kill colorectal cancer cells. The authors demonstrated that BSA–Cu SAN can efficiently catalyze H2O2 decomposition to produce ˙OH and further destroy the pathogen-tumor symbionts. Interestingly, BSA–Cu SAN could be cleared by kidneys after completing therapeutic effects.
3.3. Zn-based single atom nanozymes
Metallic Zn plays a critical role in zinc metalloenzymes, which participate in biological catalysis.53,54 In 2019, Xu et al.19 reported that an N-doped carbon nanosphere with a Zn-centered porphyrin-like structure (PMCS) exhibited POD-like activity. The highly dispersed single atom Zn site with PMCS exerted excellent inhibition effects on Pseudomonas aeruginosa by up to 99.87%. DFT calculations further demonstrated that the high catalytic activity of PMCS toward the H2O2 decomposition reaction is from the coordinatively unsaturated Zn–N4 active sites. In addition, the prepared PMCS SAzymes could significantly promote P. aeruginosa infected wound healing.
It is well known that the metal loading of SAzymes is the key to determining the catalytic activity. Increasing the metal loading is a promising method to promote the enzymatic activity of SAzymes. However, the conventional calcination method is easy to cause the aggregation of thermodynamically unstable single metal atoms.55 Therefore, the metal loading of most reported SAzymes is lower than 2%.56 Recently, Wang et al.57 have synthesized a 2D MOF-based single atom Zn nanozyme (SZN–MOFs) via a surfactant-assisted strategy. The inductively coupled plasma atomic emission spectra demonstrated that the Zn loading was up to 4.6%. The high-density single atom Zn site endowed SZN–MOFs with remarkable POD-like activity. Owing to the abundant highly toxic ˙OH generated by the Fenton-like reaction, SZN–MOFs could effectively eradicate biofilms and enhance wound healing. Apart from metal loading, insufficient endogenous H2O2 in the infected wound is another obstacle that needs to be overcome. To meet this challenge, Song et al.58 constructed a CuO2 modified Zn SAzyme (Zn SACs@CuO2). In an acid-infected wound microenvironment, CuO2 could react with H+ to produce Cu2+ and H2O2. Subsequently, single atom Zn and Cu2+ would catalyze H2O2 to produce ˙OH and destroy biofilms. Combining with the PTT effects, Zn SACs@CuO2 can efficiently heal the biofilm-infected wound.
3.4. Mn-based and Co-based single atom nanozymes
Increasing the exposure of the metal sites of SAzymes is an effective way to enhance their catalytic activity. In 2022, Xu et al.59 employed the nanoemulsion assembly method to construct spherical mesoporous single atom Mn catalysts (Mn SACs). The mesoporous structure of Mn SACs maximizes the exposure of Mn catalytic sites, leading to improved peroxidase-like (POD-like) activity. With the assistance of photothermal effects, Mn SACs could significantly increase the reaction rate of H2O2 decomposition, resulting in ˙OH production. This combination results in an excellent inhibitory effect against both E. coli and S. aureus. In an in vivo setting, Mn SACs demonstrate significant promotion of wound healing by preventing early inflammatory responses and accelerating angiogenesis (Fig. 7). However, the limited supply of H2O2 in wound tissue hinders the long-term antibacterial effect of SAzymes during the process of infected wound healing. O2 is a readily available substrate compared to H2O2 in the wound tissue. Liu et al.60 have recently introduced a Co-based SAzyme (Co–SAC) with OXD-like activity. Under 1064 nm laser irradiation, Co–SAC effectively converts O2 into O2˙− and increases the wound temperature to 53 °C. Through the synergistic effect of combined chemo-thermal therapy (CDT) and photothermal therapy (PTT), Co–SAC rapidly eliminates bacteria and promotes wound healing.
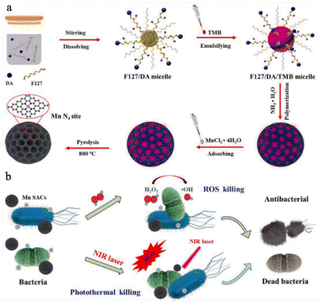 |
| Fig. 7 Illustration of the synthesis process (a) and the antibacterial mechanism (b) of Mn SACs.59 Copyright 2022, Elsevier. | |
4. Conclusion
As new-generation nanozymes, SAzymes have exhibited superior applied potential in the field of antibacterial therapy compared with conventional nanozymes. The highly dispersed catalytically active sites of SAzymes effectively catalyze the conversion of O2 or H2O2 into highly toxic reactive species such as O2˙− or ˙OH, leading to oxidative damage and bacterial death. Furthermore, the well-defined coordination structure of metal sites in SAzymes plays a crucial role in elucidating the structure–activity relationship. This minireview provides a comprehensive summary of the latest research progress in antibacterial therapy using SAzymes. We hope that this work could provide valuable insights into the development of SAzymes as innovative antibacterial agents, addressing the challenges of bacteria and biofilms and inspiring the design of future biocatalytic nanoagents for clinical applications. Despite the satisfactory bacteriostatic potential demonstrated by SAzymes, significant challenges and obstacles persist in the development of this emerging field.
Firstly, most of the reported SAzymes are carbon-based single atom catalysts. This is because N-doped carbon provides abundant N atoms to coordinate with metal atoms, resulting in a metal–Nx coordinate structure similar to natural enzymes. Therefore, N-doped carbon materials have been considered ideal supports for constructing SAzymes. Although carbon materials exhibit better biocompatibility, their long-term accumulation can still pose potential health risks. Further studies on the pharmacokinetics and dose-related toxicology are needed to better understand the potential implications of carbon-based SAzymes. Exploring the development of metabolizable SAzymes would be a promising approach for preventing the accumulation of carbon-based SAzymes.
Secondly, most of the reported SAzymes lack targeting capacity for pathogenic bacteria. In the treatment of bacterial infections, achieving precise delivery of drugs to pathogenic bacteria is crucial for improving bactericidal efficacy. Research has demonstrated that incorporating pH or GSH-responsive properties into SAzymes is an effective approach for achieving targeted delivery. Furthermore, only a limited number of reported SAzymes exhibit specific substrate recognition capabilities. To enhance the selectivity of SAzymes, various techniques such as surface conjugation, heteroatom doping, and dual-atom synergy can be employed.
Thirdly, the current studies on the catalytic mechanism of SAzymes lack a comprehensive perspective. Currently, most studies on the catalytic mechanisms of SAzymes rely on DFT calculations that primarily focus on the adsorption and activation of substrates, such as O2 and H2O2. The therapeutic process of diseases is thought to involve oxidative reactions between desorbed ROS from SAzymes and bacteria. However, this hypothesis does not take into account the complexities of the real biological environment. Therefore, there is an urgent need to develop advanced in situ characterization technologies to reveal the catalytic therapy mechanisms of SAzymes.
Conflicts of interest
The authors declare no conflict of interest in this manuscript.
Acknowledgements
Financial support from the National Natural Science Foundation of China (32171385 and 32172898) is acknowledged.
References
- X. Fan, F. Yang, C. Nie, L. Ma, C. Cheng and R. Haag, Adv. Mater., 2021, 33, e2100637 CrossRef.
- C. Zhou, Q. Wang, J. Jiang and L. Gao, Antibiotics, 2022, 11, 390 CrossRef CAS.
- B. Brar, S. Marwaha, A. K. Poonia, B. Koul, S. Kajla and V. D. Rajput, Arch. Microbiol., 2023, 205, 62 CrossRef CAS.
- Y. Xu, Z. Zhou, N. Deng, K. Fu, C. Zhu, Q. Hong, Y. Shen, S. Liu and Y. Zhang, Sci. China: Chem., 2023, 66, 1–18 Search PubMed.
- Y. Ai, Z. N. Hu, X. Liang, H. B. Sun, H. Xin and Q. Liang, Adv. Funct. Mater., 2021, 32, 2110432 CrossRef.
- L. Gao, J. Zhuang, L. Nie, J. Zhang, Y. Zhang, N. Gu, T. Wang, J. Feng, D. Yang, S. Perrett and X. Yan, Nat. Nanotechnol., 2007, 2, 577–583 CrossRef CAS.
- Q. Liu, A. Zhang, R. Wang, Q. Zhang and D. Cui, Nano-Micro Lett., 2021, 13, 154 CrossRef CAS.
- X. Zhang, S. Lin, R. Huang, A. Gupta, S. Fedeli, R. Cao-Milan, D. C. Luther, Y. Liu, M. Jiang, G. Li, B. Rondon, H. Wei and V. M. Rotello, J. Am. Chem. Soc., 2022, 144, 12893–12900 CrossRef CAS.
- Y. Wang, M. Zulpya, X. Zhang, S. Xu, J. Sun and B. Dong, Chem. Res. Chin. Univ., 2022, 38, 1324–1343 CrossRef CAS.
- J. Chen, X. Liu, G. Zheng, W. Feng, P. Wang, J. Gao, J. Liu, M. Wang and Q. Wang, Small, 2023, 19, e2205924 CrossRef.
- X. Zhang, G. Li, G. Chen, D. Wu, Y. Wu and T. D. James, Adv. Funct. Mater., 2021, 31, 2106139 CrossRef CAS.
- S. Ji, B. Jiang, H. Hao, Y. Chen, J. Dong, Y. Mao, Z. Zhang, R. Gao, W. Chen, R. Zhang, Q. Liang, H. Li, S. Liu, Y. Wang, Q. Zhang, L. Gu, D. Duan, M. Liang, D. Wang, X. Yan and Y. Li, Nat. Catal., 2021, 4, 407–417 CrossRef CAS.
- X. Zhang, G. Li, G. Chen, D. Wu, X. Zhou and Y. Wu, Coord. Chem. Rev., 2020, 418, 213376 CrossRef CAS.
- B. Qiao, A. Wang, X. Yang, L. F. Allard, Z. Jiang, Y. Cui, J. Liu, J. Li and T. Zhang, Nat. Chem., 2011, 3, 634–641 CrossRef CAS PubMed.
- M. Tang, J. Li, X. Cai, T. Sun and C. Chen, Chem. – Asian J., 2022, 17, e202101422 CrossRef CAS PubMed.
- B. Jiang, Z. Guo and M. Liang, Nano Res., 2023, 16, 1878–1889 CrossRef CAS PubMed.
- C. Jin, S. Fan, Z. Zhuang and Y. Zhou, Nano Res., 2023, 16, 1992–2002 CrossRef.
- Y. Sun, B. Xu, X. Pan, H. Wang, Q. Wu, S. Li, B. Jiang and H. Liu, Coord. Chem. Rev., 2023, 475, 214896 CrossRef CAS.
- B. Xu, H. Wang, W. Wang, L. Gao, S. Li, X. Pan, H. Wang, H. Yang, X. Meng, Q. Wu, L. Zheng, S. Chen, X. Shi, K. Fan, X. Yan and H. Liu, Angew. Chem., Int. Ed., 2019, 58, 4911–4916 CrossRef CAS.
- Y. Zhu, Y. Liao, J. Zou, J. Cheng, Y. Pan, L. Lin and X. Chen, Small, 2023, e2300750 CrossRef.
- F. Meng, P. Zhu, L. Yang, L. Xia and H. Liu, Chem. Eng. J., 2023, 452, 139411 CrossRef CAS.
- T. Shi, Y. Cui, H. Yuan, R. Qi and Y. Yu, Nanomaterials, 2023, 13, 2760 CrossRef CAS PubMed.
- C. Peng, R. Pang, J. Li and E. Wang, Adv. Mater., 2023, 2211724 CrossRef.
- S. Cai, W. Zhang and R. Yang, Nano Res., 2023 DOI:10.1007/s12274-023-5864-y.
- L. Ye, Z. Cao, X. Liu, Z. Cui, Z. Li, Y. Liang, S. Zhu and S. Wu, J. Alloys Compd., 2022, 904, 164091 CrossRef CAS.
- Y. Chen, P. Wang, H. Hao, J. Hong, H. Li, S. Ji, A. Li, R. Gao, J. Dong, X. Han, M. Liang, D. Wang and Y. Li, J. Am. Chem. Soc., 2021, 143, 18643–18651 CrossRef CAS PubMed.
- Y. Yu, Y. Cheng, L. Tan, X. Liu, Z. Li, Y. Zheng, T. Wu, Y. Liang, Z. Cui, S. Zhu and S. Wu, Chem. Eng. J., 2022, 431, 133279 CrossRef CAS.
- Y. Yu, L. Tan, Z. Li, X. Liu, Y. Zheng, X. Feng, Y. Liang, Z. Cui, S. Zhu and S. Wu, ACS Nano, 2021, 15, 10628–10639 CrossRef CAS PubMed.
- Y. Fan, X. Gan, H. Zhao, Z. Zeng, W. You and X. Quan, Chem. Eng. J., 2022, 427, 131572 CrossRef CAS.
- Z. Li, D. Xu, Z. Deng, J. Yin, Y. Qian, J.-T. Hou, X. Ding, J. Shen and X. He, Chem. Eng. J., 2023, 452, 139587 CrossRef CAS.
- Z. Wang, H. Li, W. Zhou, J. Lee, Z. Liu, Z. An, D. Xu, H. Mo, L. Hu and X. Zhou, Biomaterials, 2022, 290, 121842 CrossRef CAS PubMed.
- X. Shen, R. Ma, Y. Huang, L. Chen, Z. Xu, D. Li, X. Meng, K. Fan, J. Xi, X. Yan, H. Koo, Y. Yang, J. Jiang and L. Gao, Nano Today, 2020, 35, 100981 CrossRef CAS.
- L. Fang, R. Ma, X. J. Gao, L. Chen, Y. Liu, Y. Huo, T. Wei, X. Wang, Q. Wang, H. Wang, C. Cui, Q. Shi, J. Jiang and L. Gao, Adv. Sci., 2022, 9, e2104341 CrossRef.
- B. Sun, X. Wang, Z. Ye, J. Zhang, X. Chen, N. Zhou, M. Zhang, C. Yao, F. Wu and J. Shen, Adv. Sci., 2023, e2207507 CrossRef.
- J. Pei, R. Zhao, X. Mu, J. Wang, C. Liu and X. D. Zhang, Biomater. Sci., 2020, 8, 6428–6441 RSC.
- M. Li, H. Zhang, Y. Hou, X. Wang, C. Xue, W. Li, K. Cai, Y. Zhao and Z. Luo, Nanoscale Horiz., 2020, 5, 202–217 RSC.
- M. Huo, L. Wang, H. Zhang, L. Zhang, Y. Chen and J. Shi, Small, 2019, 15, e1901834 CrossRef.
- L. Huang, J. Chen, L. Gan, J. Wang and S. Dong, Sci. Adv., 2019, 5, eaav5490 CrossRef CAS.
- R. F. Landis, C. H. Li, A. Gupta, Y. W. Lee, M. Yazdani, N. Ngernyuang, I. Altinbasak, S. Mansoor, M. A. S. Khichi, A. Sanyal and V. M. Rotello, J. Am. Chem. Soc., 2018, 140, 6176–6182 CrossRef CAS PubMed.
- D. Hu, Y. Deng, F. Jia, Q. Jin and J. Ji, ACS Nano, 2020, 14, 347–359 CrossRef CAS.
- Q. Xu, Y. Hua, Y. Zhang, M. Lv, H. Wang, Y. Pi, J. Xie, C. Wang and Y. Yong, Adv. Healthcare Mater., 2021, 10, e2101374 CrossRef.
- Y. Feng, J. Qin, Y. Zhou, Q. Yue and J. Wei, J. Colloid Interface Sci., 2022, 606, 826–836 CrossRef CAS.
- R. Yang, Y. Wei, M. Zhao, M. Shi, Y. Zhao and P. Sun, Colloids Surf., B, 2022, 219, 112811 CrossRef CAS PubMed.
- B. Ma, S. Wang, F. Liu, S. Zhang, J. Duan, Z. Li, Y. Kong, Y. Sang, H. Liu, W. Bu and L. Li, J. Am. Chem. Soc., 2019, 141, 849–857 CrossRef CAS PubMed.
- X. Wang, Q. Shi, Z. Zha, D. Zhu, L. Zheng, L. Shi, X. Wei, L. Lian, K. Wu and L. Cheng, Bioact. Mater., 2021, 6, 4389–4401 CAS.
- Y. Zhao, Y. Yu, F. Gao, Z. Wang, W. Chen, C. Chen, J. Yang, Y. Yao, J. Du, C. Zhao and Y. Wu, Nano Res., 2021, 14, 4808–4813 CrossRef CAS.
- J. Zhu, Q. Li, X. Li, X. Wu, T. Yuan and Y. Yang, Langmuir, 2022, 38, 6860–6870 CrossRef CAS.
- X. Qiu, L. Zhuang, J. Yuan, H. Wang, X. Dong, S. He, S. Guan, Z. Chang and P. Bao, J. Colloid Interface Sci., 2023, 652, 1712–1725 CrossRef CAS PubMed.
- J. R. Raneses, A. L. Ellison, B. Liu and K. M. Davis, mBio, 2020, 11, e00901–e00920 CrossRef CAS PubMed.
- S. Ma, Z. Han, K. Leng, X. Liu, Y. Wang, Y. Qu and J. Bai, Small, 2020, 16, e2001384 CrossRef.
- J. Bai, Y. Feng, W. Li, Z. Cheng, J. M. Rosenholm, H. Yang, G. Pan, H. Zhang and D. Geng, Research, 2023, 6, 1–13 CrossRef.
- X. Wang, Q. Chen, Y. Zhu, K. Wang, Y. Chang, X. Wu, W. Bao, T. Cao, H. Chen, Y. Zhang and H. Qin, Signal Transduction Targeted Ther., 2023, 8, 277 CrossRef CAS PubMed.
- M. Hernick and C. A. Fierke, Arch. Biochem. Biophys., 2005, 433, 71–84 CrossRef CAS PubMed.
- V. Navratil, V. Klusak and L. Rulisek, Chemistry, 2013, 19, 16634–16645 CrossRef CAS.
- J. Li, S. Chen, N. Yang, M. Deng, S. Ibraheem, J. Deng, J. Li, L. Li and Z. Wei, Angew. Chem., Int. Ed., 2019, 58, 7035–7039 CrossRef CAS.
- W. Wu, L. Huang, E. Wang and S. Dong, Chem. Sci., 2020, 11, 9741–9756 RSC.
- X. Wang, W. Hu, X. H. Xia and C. Wang, Adv. Funct. Mater., 2022, 33, 2212798 CrossRef.
- J. Song, H. Chen, Y. Lv, W. Yang, F. Zhang, T. Wang, D. Liu, Y. Qu, L. Han, J. Fu and X. Kong, Chem. Eng. J., 2023, 474, 145706 CrossRef CAS.
- W. Xu, B. Sun, F. Wu, M. Mohammadniaei, Q. Song, X. Han, W. Wang, M. Zhang, N. Zhou and J. Shen, Chem. Eng. J., 2022, 438, 135636 CrossRef CAS.
- X. Liu, Q. Liu, X. He, G. Yang, X. Chen, J. Meng, B. Hu, Y. Qian, J. Shen, L. Jin and X. Zhang, Appl. Surf. Sci., 2023, 612, 155866 CrossRef CAS.
Footnote |
† These authors contributed equally to this work. |
|
This journal is © The Royal Society of Chemistry 2024 |