DOI:
10.1039/D2TC05270A
(Perspective)
J. Mater. Chem. C, 2023,
11, 2826-2830
Construction of gel networks via [2+2] photocycloaddition
Received
10th December 2022
, Accepted 23rd January 2023
First published on 13th February 2023
Abstract
Gel networks could be achieved via the photocycloaddition and cross-linking of olefin moieties in branched polymers. The formed cyclobutane unit made the bulky gel photoreversible, which shows potential for promising applications in self-healing, drug release and extracellular matrices. Related research on gel networks formed via [2+2] photocycloaddition was retraced and the potential utilization of visible light catalysis was also conceived.
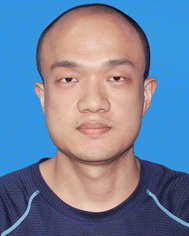
Lei-Min Zhao
| Dr Lei-Min Zhao obtained his PhD degree from Technical Institute of Physics and Chemistry, Chinese Academy of Sciences under the supervision of Prof. Li-Zhu Wu and Prof. Chen-Ho Tung. Now he is a research assistant professor in Southern University of Science and Technology. His research focuses on photocatalysis, cross couplings, gels and rotaxanes. |
The [2+2] photocycloaddition of two C
C bonds is a versatile tool to access carbocyclic compounds.1–7 In the reaction, one olefin is excited to its first excited singlet state or successively to its first excited triplet state, and then two new bonds are formed at both carbon atoms of excited olefins by another olefin to obtain cyclobutane products in a single operation.
For its convenience, [2+2] photocycloaddition plays a predominant role in total synthesis.8–12 Moreover, it also attracts materials scientists’ attention in the field of photoresponsive polymer gels13–18 owing to its intrinsic reversible property controlled by different wavelengths of UV light or by high temperatures. Usually, a longer wavelength of UV light causes the cycloaddition of olefins, while a shorter wavelength and high temperature induce reversion (Scheme 1).19,20
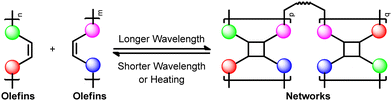 |
| Scheme 1 Construction of gel networks via 2+2 photocycloaddition. | |
Since gel networks built by full-fledged chemical covalent crosslinking or physical noncovalent interactions21–32 have been widely used in the field of biomaterials science and biomedical materials,33–37 the investigation of the new type of network via [2+2] cycloaddition is still on the way, and some seminal work emerged one after another, most of which were focusing on the modification of the monomer structure, reaction condition and biocompatibility. In this perspective, we will discuss the construction of gel networks via [2+2] photocycloaddition and the possible applications in biology, and present a brief future direction about the topic.
In 2013, Yang and coworkers38 demonstrated a novel multiresponsive hydrogel based on polyacrylamide-functionalized thymine derivatives (Fig. 1A). Under the irradiation of 365 nm wavelength light, a polyacrylamide-based hydrogel was obtained via the [2+2] photocycloaddition of two thymine monomers linked by a polyacrylamide chain. Furthermore, the gel transformed to a solution because of the thymine dimer's photocleavage upon UV-light irradiation (λmax = 240 nm).
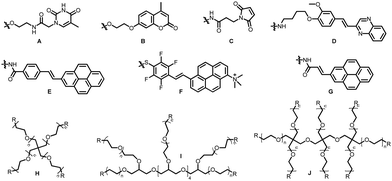 |
| Fig. 1 . Reactive substrates and branched linking polymers. (A) Thymaine moiety; (B) Coumarin moiety; (C) Maleimide moiety; (D) Styrylquinoxaline moiety; (E) Styrylpyrene moiety; (F) 1-trimethylammonium-6-pentafluorstyryl-pyrene moiety; (G) Arylamidylpyrene moiety; (H) Four-arm PEGs; (I) Eight-arm PEGs reported by Frisch, Truong, Kowollik; (J) Eight-arm PEGs reported by Kowollik group. | |
Zhong, Xing and co-workers39 reported a photoreversible self-healing and cytocompatible hydrogel system. The photo active unit is polyacrylate-modified coumarin (Fig. 1B), which induced cycloaddition to generate gels and exhibited excellent self-healing ability at wavelengths of 365 nm and 254 nm. Besides, it possesses high mechanical strength after healing for 60 min with a stress intensity of 2 × 105 Pa, and the elongation at break is over 96%. Furthermore, a poly amidoamine crosslinker is used to enhance cell compatibility and the referred gels would regulate bone marrow stromal cell differentiation.
Schlierf and co-workers40 utilized a two-photon [2+2] cycloaddition reaction of maleimide groups (Fig. 1H, 4R = 4C) to modify surfaces with precision in the submicrometer scale, and optimal conditions of maximizing the structure at minimal energy deposition for potential make it possible to access bottom-up hydrogel structures with fiber dimensions below 350 nm, mimicking aspects of the extra-cellular matrix, which is a promising method from single-cell in situ encapsulation to micrometer precision incorporation of signaling molecules for the differentiation of cells.
Truong, Fosythe and co-workers41 reported photolabile hydrogels utilizing the reversible [2+2] photocycloaddition by 4-arm PEG-SP (Mw = 20
000 g mol−1, SP = styrylpyrene) (Fig. 1H, 4R = 4E), and the cross-linked gel could maintain its morphology up to a concentration of 8 wt% (4 mM) in water with a storage modulus value of 6.8 ± 0.8 kPa. Remarkably, a low concentration (c = 1 mM) does not affect the cross-linking process, and the formed gel gives a G′ value of 0.5 ± 0.1 kPa upon UV light excitation at 340 nm. The network collapses since the cleavage of the cyclobutane unit in the network and lower concentration would cause a higher degradation efficiency and effective repeatable photocuring–photodegradation. However, photodegradation shows less efficiency at subsequent cycles at higher concentrations.
Besides, the obtained gels were compatible in a PBS buffer solution for one week at 37 °C. They can encapsulate human mesenchymal stem cells, which would apply to disease progression and tissue regeneration.
Later, based on the above-mentioned task, Frisch, Kowollik and Truong reported a four-arm PEG-AP (Fig. 1H, 4R = 4G, AP = arylamidylpyrene) and a hybrid with styrylpyrene (Fig. 1H, 4R = 2E + 2G)42 to undergo [2+2] cycloaddition to get the indicated cross-linked polymers under irradiation at 455 mm. The hybrid PEG consisting of two arylamidylpyrene and two styrylpyrene is mainly detected. Then, four-arm-SP-AP-PEG stiffens and shrinks at λmax = 455 nm and λmax = 420 nm one after another but shows no harm to cells.
In 2020, Walther and coworkers in collaboration with Frisch and Kowollik designed a new kind of photodynamic crosslinking unit, 1-trimethylammonium-6-pentafluorstyryl-pyrene,43 which can perform [2+2] photocycloaddition upon visible light irradiation at 470 nm in water. Besides, A pentafluoro moiety makes molecule modular and easy access to the functionalization by thiolated macromolecules via nucleophilic aromatic substitution. Thus, a four-arm-PEG-SH-modified qStyPy, named sPEG-qStyPy (Mn = 23
110 Da) (Fig. 1H, 4R = 4F) in the original article, was synthesized after brief investigation of qStyPy in water. The association of given sPEG-qStyPy is based on reversible supramolecular interactions via hydrophilic ammonium and hydrophobic styrylpyrene. The former enables homogeneous distribution upon irradiation of a fiber-coupled 470 mm LED. The cross-linked hydrogels are elastic and viscous in accordance with the storage value G′ and the loss modulus G′′, and the stiffness of the hydrogels increased smoothly as the irradiation time reached 20 h. The amplitude sweep shows an elastically cross-linked network with a yield point at a strain amplitude of γy ≈ 250% and a flow point at γy ≈ 590%, and the frequency sweep suggests sacrificial supramolecular cross-links via sticky-π–π interactions playing a key role in the network apart from the covalent crosslinks by [2+2] cycloaddition. However, the positive charge and extended π-system make it possible to intercalate into DNA. The potential toxicity needs further investigation.
Frisch, Truong, Kowollik and co-workers reported [2+2] photocycloaddition upon the green light illumination of a halochromic system dependent on a styrylquinoxaline unit,44 and the “on” and “off” state of the process could be switched by adjusting the pH of the system. Further investigations indicated that the water solution gives higher conversion and a longer activation wavelength than acetonitrile under indicated conditions. Then, eight-arm PEG-functionalized styrylquinoxaline (20
000 g mol−1) (Fig. 1I, 8R = 8D) was synthesized to undergo [2+2] cycloaddition between 400 nm and 510 nm, in which hydrogels were formed with a range of mechanical properties and pH responsiveness.
The light of λ = 420 nm and 450 nm present the most effective gelation rate among multiple irradiation processes at different wavelengths under the same irradiation intensity. If one of the two parallel tubes of polymer solution (c = 5 mM, thickness = ca. 1 cm) was irradiated at 425 nm or 405 nm, and the other at 455 nm, the thickness of the obtained gels is 0.2 cm and 1 cm after 1 h of irradiation respectively. Cell viability studies of hydrogels containing encapsulation fibroblasts (L929) highlight the possibility of the gel in cell biology.
Besides, the decreased pH would slow down the crosslinking rate and could entirely suppress the process at pH = 1, yet the reactivity recovered when the pH was adjusted to neutral or basic. In addition, an enlarged concentration of the polymer brings an exponential increase in stiffness from 1 mM to 10 mM, giving the modulus values from 0.5 kPa to 40 kPa.
On the basis of the above-mentioned achievement, the Kowollik group intensively developed a λ-orthogonal photochemical system45 for the design of hydrogels, based on a halochromic styrylpyrido[2,3-b]pyrazine moiety (SPP) and a non-halochromic acrylamidylpyrene function (AAP), and its stiffness can be independently adjusted by the green light or blue light (Fig. 1J, 8R = 8D and 8R = 4D + 4G).
The photoreactivity of SPP is pH dependent, thus the acidic environment will inhibit cycloaddition. By using a spiropyran-based photoacid generator with an appropriate absorption wavelength, the activation wavelength of the SPP moiety was limited to the green light region (520–550 nm), thereby realizing the photoactivation of the AAP group.
Above all, [2+2] cycloaddition processes are indispensable in each published work, and play a key role in cross-linking via the formation of multiple cyclobutane units; otherwise, it is unable to form gel networks.
Summary and outlooks
In the synthesis of natural products and some cage-like compounds, [2+2] photocycloaddition chemistry has achieved great success for decades. Because of its convenience to construct two bonds concurrently with high efficiency, [2+2] photocycloaddition is increasingly emerging as a versatile tool for the construction of gel networks. The reversible process makes it photoresponsive with promising applications of self-healing materials, actuators, drug release, and extracellular matrices, though drawbacks accompanied the merits. For example, most of the gelation processes of cycloaddition are conducted upon UV light excitation, which is harmful to human bodies. However, the reaction conditions were not invariable; it all depends on the excited-state energy of distinct olefins, some π-extended and heteroatom-modified molecules could react at a red-shift wavelength. Though these subsequently developed strategies can fill the gap, the functional groups might be limited and toxic to tissues and the reversible reaction upon directly irradiation might be inefficient since the cleavage of carbon–carbon single bonds need high energies, which made the other functional groups fragile. In addition, the isomers of Head-to-Head, Head-to-Tail, syn, anti, cis and trans would bring difficulties to characterizations and poor reproducibilities to the transformations.
With the development of photochemistry, [2+2] cycloaddition upon visible light catalysis46–51 of two olefins can occur mildly and smoothly via single-electron transfer and energy transfer.52–71 Compared with the traditional [2+2] photocycloaddition, visible light-induced photocatalytic reactions occur under extremely mild conditions, with most reactions proceeding at room temperature and highly reactive radical initiators were hardly used. The light source is typically a commercial household light bulb rather than the specialized equipment required for processes employing high-energy UV light. Additionally, since most organic molecules generally do not absorb visible light, there are little potential possibilities for deleterious side reactions that might arise from the direct photoexcitation of the substrate itself. Finally, catalyst loadings in photocatalysis may be at a very low level.
Under the circumstances, the biocompatible and bio-applicable photocatalysis process was further investigated in vitro and in cellulo.72–82 However, photocatalyzed [2+2] reactions were not developed in this field, nor the formation of gel network, probably due to the difficulties in the removal of the photocatalyst and some potential additives. As a result, the inductions of bio-compatible catalysis and leaving groups in the system would be crucial and green process of visible light photocatalyzed [2+2] will take a good effect in the construction of gel networks and the applications in biomedical science.
Conflicts of interest
There are no conflicts to declare.
Acknowledgements
This research was financially supported by Guangdong Basic and Applied Basic Research Foundation (No. 2019A1515110216) and Post-doctorate Scientific Research Fund for staying at Shenzhen (K21217531).
Notes and references
- M. T. Crimmins, Chem. Rev., 1988, 88, 1453–1473 CrossRef CAS.
- F. Mueller and J. Mattay, Chem. Rev., 1993, 93, 99–117 CrossRef CAS.
- D. I. Schuster, G. Lem and N. A. Kaprinidis, Chem. Rev., 1993, 93, 3–22 CrossRef CAS.
- E. Lee-Ruff and G. Mladenova, Chem. Rev., 2003, 103, 1449–1484 CrossRef CAS PubMed.
- J. C. Namyslo and D. E. Kaufmann, Chem. Rev., 2003, 103, 1485–1538 CrossRef CAS PubMed.
- S. Poplata, A. Tröster, Y.-Q. Zou and T. Bach, Chem. Rev., 2016, 116, 9748–9815 CrossRef CAS PubMed.
- V. Ramamurthy and J. Sivaguru, Chem. Rev., 2016, 116, 9914–9993 CrossRef CAS PubMed.
- M. Demuth, Pure Appl. Chem., 1986, 58, 1233–1238 CrossRef CAS.
- H. D. Roth, Angew. Chem., Int. Ed. Engl., 1989, 28, 1193–1207 CrossRef.
- D. De Keukeleire and S. L. He, Chem. Rev., 1993, 93, 359–380 CrossRef CAS.
- N. Hoffmann, Chem. Rev., 2008, 108, 1052–1103 CrossRef CAS PubMed.
- T. Bach and J. P. Hehn, Angew. Chem., Int. Ed., 2011, 50, 1000–1045 CrossRef CAS PubMed.
- L. Li, J. M. Scheiger and P. A. Levkin, Adv. Mater., 2019, 31, 1807333 CrossRef PubMed.
- M. R. A. Bhatti, A. Kernin, M. Tausif, H. Zhang, D. Papageorgiou, E. Bilotti, T. Peijs and C. W. M. Bastiaansen, Adv. Opt. Mater., 2022, 10, 2102186 CrossRef CAS.
- J. Cao, D. Zhang, Y. Zhou, Q. Zhang and S. Wu, Macromol. Rapid Commun., 2022, 43, 2100703 CrossRef CAS PubMed.
- F. Khan, M. Atif, M. Haseen, S. Kamal, M. S. Khan, S. Shahid and S. A. A. Nami, J. Mater. Chem. B, 2022, 10, 170–203 RSC.
- K. Peng, L. Zheng, T. Zhou, C. Zhang and H. Li, Acta Biomater., 2022, 137, 20–43 CrossRef CAS PubMed.
- Z. Yuan, J. Ding, Y. Zhang, B. Huang, Z. Song, X. Meng, X. Ma, X. Gong, Z. Huang, S. Ma, S. Xiang and W. Xu, Eur. Polym. J., 2022, 177, 111473 CrossRef CAS.
-
M. Montalti, A. Credi, L. Prodi and M. T. Gandolfi, Handbook of Photochemistry, Taylor & Francis, Boca Raton, 2006 Search PubMed.
-
N. J. Turro, V. Ramamurthy and J. C. Scaiano, Modern Molecular Photochemistry of Organic Molecules, University Science Books, Sausalito, CA, 2010 Search PubMed.
- A. Garcia, M. Marquez, T. Cai, R. Rosario, Z. Hu, D. Gust, M. Hayes, S. A. Vail and C.-D. Park, Langmuir, 2007, 23, 224–229 CrossRef CAS PubMed.
- M. C. Roberts, M. C. Hanson, A. P. Massey, E. A. Karren and P. F. Kiser, Adv. Mater., 2007, 19, 2503–2507 CrossRef CAS.
- G. Deng, C. Tang, F. Li, H. Jiang and Y. Chen, Macromolecules, 2010, 43, 1191–1194 CrossRef CAS.
- M. Nakahata, Y. Takashima, H. Yamaguchi and A. Harada, Nat. Commun., 2011, 2, 511 CrossRef PubMed.
- G. Deng, F. Li, H. Yu, F. Liu, C. Liu, W. Sun, H. Jiang and Y. Chen, ACS Macro Lett., 2012, 1, 275–279 CrossRef CAS PubMed.
- J.-Y. Sun, X. Zhao, W. R. K. Illeperuma, O. Chaudhuri, K. H. Oh, D. J. Mooney, J. J. Vlassak and Z. Suo, Nature, 2012, 489, 133 CrossRef CAS PubMed.
- Y. Jin, C. Yu, R. J. Denman and W. Zhang, Chem. Soc. Rev., 2013, 42, 6634–6654 RSC.
- C. Katsuno, A. Konda, K. Urayama, T. Takigawa, M. Kidowaki and K. Ito, Adv. Mater., 2013, 25, 4636–4640 CrossRef CAS PubMed.
- Z. Wei, J. H. Yang, X. J. Du, F. Xu, M. Zrinyi, Y. Osada, F. Li and Y. M. Chen, Macromol. Rapid Commun., 2013, 34, 1464–1470 CrossRef CAS PubMed.
- W. Xi, T. F. Scott, C. J. Kloxin and C. N. Bowman, Adv. Funct. Mater., 2014, 24, 2572–2590 CrossRef CAS.
- J. Yang, R. Bai and Z. Suo, Adv. Mater., 2018, 30, 1800671 CrossRef PubMed.
- R. Eelkema and A. Pich, Adv. Mater., 2020, 32, 1906012 CrossRef CAS PubMed.
- M. Hamidi, A. Azadi and P. Rafiei, Adv. Drug Delivery Rev., 2008, 60, 1638–1649 CrossRef CAS PubMed.
- C. A. DeForest and K. S. Anseth, Nat. Chem., 2011, 3, 925–931 CrossRef CAS PubMed.
- A. S. Hoffman, Adv. Drug Delivery Rev., 2012, 64, 18–23 CrossRef.
- X. Ma and Y. Zhao, Chem. Rev., 2015, 115, 7794–7839 CrossRef CAS PubMed.
- S. Li, S. Dong, W. Xu, S. Tu, L. Yan, C. Zhao, J. Ding and X. Chen, Adv. Sci., 2018, 5, 1700527 CrossRef PubMed.
- K. Yang and M. Zeng, New J. Chem., 2013, 37, 920–926 RSC.
- L. Yu, K. Xu, L. Ge, W. Wan, A. Darabi, M. Xing and W. Zhong, Macromol. Biosci., 2016, 16, 1381–1390 CrossRef CAS PubMed.
- C. Jungnickel, M. V. Tsurkan, K. Wogan, C. Werner and M. Schlierf, Adv. Mater., 2017, 29, 1603327 CrossRef PubMed.
- V. X. Truong, F. Li, F. Ercole and J. S. Forsythe, ACS Macro Lett., 2018, 7, 464–469 CrossRef CAS PubMed.
- K. Kalayci, H. Frisch, C. Barner-Kowollik and V. X. Truong, Adv. Funct. Mater., 2020, 30, 1908171 CrossRef CAS.
- S. Ludwanowski, D. Hoenders, K. Kalayci, H. Frisch, C. Barner-Kowollik and A. Walther, Chem. Commun., 2021, 57, 805–808 RSC.
- K. Kalayci, H. Frisch, V. X. Truong and C. Barner-Kowollik, Nat. Commun., 2020, 11, 4193 CrossRef PubMed.
- V. X. Truong, J. Bachmann, A.-N. Unterreiner, J. P. Blinco and C. Barner-Kowollik, Angew. Chem., Int. Ed., 2022, 61, e202113076 CrossRef CAS PubMed.
- J. Xuan and W.-J. Xiao, Angew. Chem., Int. Ed., 2012, 51, 6828–6838 CrossRef CAS PubMed.
- C. K. Prier, D. A. Rankic and D. W. C. MacMillan, Chem. Rev., 2013, 113, 5322–5363 CrossRef CAS PubMed.
- J. M. R. Narayanam and C. R. J. Stephenson, Chem. Soc. Rev., 2011, 40, 102–113 RSC.
- Q. Liu and L.-Z. Wu, Natl. Sci. Rev., 2017, 4, 359–380 CrossRef CAS.
- K. L. Skubi, T. R. Blum and T. P. Yoon, Chem. Rev., 2016, 116, 10035–10074 CrossRef CAS PubMed.
- D. M. Schultz and T. P. Yoon, Science, 2014, 343, 1239176 CrossRef PubMed.
- M. A. Ischay, M. E. Anzovino, J. Du and T. P. Yoon, J. Am. Chem. Soc., 2008, 130, 12886–12887 CrossRef CAS PubMed.
- J. Du and T. P. Yoon, J. Am. Chem. Soc., 2009, 131, 14604–14605 CrossRef CAS PubMed.
- M. A. Ischay, Z. Lu and T. P. Yoon, J. Am. Chem. Soc., 2010, 132, 8572–8574 CrossRef CAS PubMed.
- M. A. Ischay, M. S. Ament and T. P. Yoon, Chem. Sci., 2012, 3, 2807–2811 RSC.
- Z. Lu and T. P. Yoon, Angew. Chem., Int. Ed., 2012, 51, 10329–10332 CrossRef CAS PubMed.
- J. Du, K. L. Skubi, D. M. Schultz and T. P. Yoon, Science, 2014, 344, 392–396 CrossRef CAS PubMed.
- A. E. Hurtley, Z. Lu and T. P. Yoon, Angew. Chem., Int. Ed., 2014, 53, 8991–8994 CrossRef CAS PubMed.
- Z. D. Miller, B. J. Lee and T. P. Yoon, Angew. Chem., Int. Ed., 2017, 56, 11891–11895 CrossRef CAS PubMed.
- M. E. Daub, H. Jung, B. J. Lee, J. Won, M.-H. Baik and T. P. Yoon, J. Am. Chem. Soc., 2019, 141, 9543–9547 CrossRef CAS PubMed.
- J. Zheng, W. B. Swords, H. Jung, K. L. Skubi, J. B. Kidd, G. J. Meyer, M.-H. Baik and T. P. Yoon, J. Am. Chem. Soc., 2019, 141, 13625–13634 CrossRef CAS PubMed.
- C. S. Gravatt, L. Melecio-Zambrano and T. P. Yoon, Angew. Chem., Int. Ed., 2021, 60, 3989–3993 CrossRef CAS PubMed.
- E. M. Sherbrook, M. J. Genzink, B. Park, I. A. Guzei, M.-H. Baik and T. P. Yoon, Nat. Commun., 2021, 12, 5735 CrossRef CAS PubMed.
- S. J. Chapman, W. B. Swords, C. M. Le, I. A. Guzei, F. D. Toste and T. P. Yoon, J. Am. Chem. Soc., 2022, 144, 4206–4213 CrossRef CAS PubMed.
- Z. C. Girvin, L. F. Cotter, H. Yoon, S. J. Chapman, J. M. Mayer, T. P. Yoon and S. J. Miller, J. Am. Chem. Soc., 2022, 144, 20109–20117 CrossRef CAS PubMed.
- T. Lei, C. Zhou, M.-Y. Huang, L.-M. Zhao, B. Yang, C. Ye, H. Xiao, Q.-Y. Meng, V. Ramamurthy, C.-H. Tung and L.-Z. Wu, Angew. Chem., Int. Ed., 2017, 56, 15407–15410 CrossRef CAS PubMed.
- L.-M. Zhao, T. Lei, R.-Z. Liao, H. Xiao, B. Chen, V. Ramamurthy, C.-H. Tung and L.-Z. Wu, J. Org. Chem., 2019, 84, 9257–9269 CrossRef CAS PubMed.
- Z. Liu, C. Zhou, T. Lei, X.-L. Nan, B. Chen, C.-H. Tung and L.-Z. Wu, CCS Chem., 2021, 2, 582–588 CrossRef.
- C.-J. Wu, W.-X. Cao, B. Chen, C.-H. Tung and L.-Z. Wu, Org. Lett., 2021, 23, 2135–2139 CrossRef CAS PubMed.
- Y. Jiang, C. Wang, C. R. Rogers, M. S. Kodaimati and E. A. Weiss, Nat. Chem., 2019, 11, 1034–1040 CrossRef CAS PubMed.
- Y. Jiang, M. Yang, Y. Wu, R. López-Arteaga, C. R. Rogers and E. A. Weiss, Chem. Catal., 2021, 1, 106–116 CrossRef PubMed.
- D. A. Fancy and T. Kodadek, Proc. Natl. Acad. Sci. U. S. A., 1999, 96, 6020–6024 CrossRef CAS PubMed.
- Y. Chen, A. S. Kamlet, J. B. Steinman and D. R. Liu, Nat. Chem., 2011, 3, 146–153 CrossRef CAS PubMed.
- K. K. Sadhu, T. Eierhoff, W. Römer and N. Winssinger, J. Am. Chem. Soc., 2012, 134, 20013–20016 CrossRef CAS PubMed.
- S. Sato and H. Nakamura, Angew. Chem., Int. Ed., 2013, 52, 8681–8684 CrossRef CAS PubMed.
- H. Huang, G. Zhang, L. Gong, S. Zhang and Y. Chen, J. Am. Chem. Soc., 2014, 136, 2280–2283 CrossRef CAS PubMed.
- C. Hu and Y. Chen, Tetrahedron Lett., 2015, 56, 884–888 CrossRef CAS.
- H. Wang, W.-G. Li, K. Zeng, Y.-J. Wu, Y. Zhang, T.-L. Xu and Y. Chen, Angew. Chem., Int. Ed., 2019, 58, 561–565 CrossRef CAS PubMed.
- Y. Chen, ChemPhotoChem, 2020, 4, 319–320 CrossRef CAS.
- H. Wang, Y. Zhang, K. Zeng, J. Qiang, Y. Cao, Y. Li, Y. Fang, Y. Zhang and Y. Chen, JACS Au, 2021, 1, 1066–1075 CrossRef CAS PubMed.
- K. Zeng, L. Han and Y. Chen, ChemBioChem, 2022, 23, e202200244 CrossRef CAS PubMed.
- Y. Zhang, L. Han, X. Tian, C. Peng and Y. Chen, Angew. Chem., Int. Ed., 2022, 61, e202115472 CAS.
|
This journal is © The Royal Society of Chemistry 2023 |