DOI:
10.1039/D2TC04148K
(Paper)
J. Mater. Chem. C, 2023,
11, 1696-1703
Mode-locking pulse generation based on lead-free halide perovskite CsCu2I3 micro-rods with high stability†
Received
30th September 2022
, Accepted 28th November 2022
First published on 3rd December 2022
Abstract
The industrialization and commercialization of optoelectronic devices require the exploration of novel materials with high stability, nontoxicity, and large-scale manufacturing. The all-inorganic lead-free halide perovskite nanomaterial CsCu2I3 has been widely reported in applications in light-emitting diodes (LEDs), photodetectors (PDs), photovoltaics, and so on, due to its good environmental stability and unique optoelectronic properties. However, research on ultrafast photonics has rarely been carried out so far. Here, high quality CsCu2I3 micro-rods were fabricated via a solvent evaporation crystallization method. The high thermodynamic stability and the broadband response of CsCu2I3-based optoelectronic devices have been predicted by density functional theory (DFT), revealing their feasibility for exploitation in fiber laser systems. The nonlinear characteristic of CsCu2I3 micro-rods was measured using a balanced twin-detector system, with a modulation depth of 12.04%. Utilizing its remarkable nonlinear optical response and intrinsic stability, a passively mode-locked erbium-doped fiber laser based on a CsCu2I3 saturable absorber was achieved. Significantly, the fiber laser could operate stably for at least five months. These experimental results demonstrate that CsCu2I3 micro-rods are ultra-stable, and can serve as a promising optical modulation material to produce an ultrafast and long-stability pulse in fiber laser applications.
Introduction
Ultrafast fiber lasers have been proven to be indispensable tools for various crucial applications, such as ultrafast spectroscopy, micromachining, next-generation communication, and intelligent health.1–4 A saturable absorber (SA) is an important optical component to trigger pulsed operation.5 Recently, the application of SAs based on novel low-dimensional materials for ultrafast pulse generation has been widely reported, such as graphene,6–8 carbon nanotubes (CNTs),9–11 topological insulators (TIs),12–15 transition metal dichalcogenides (TMDs),16–19 black phosphorus (BP),20 and so on.21–25 Most of them have unique optical and electrical properties. For instance, graphene possesses zero bandgap, leading to broadband absorption but low modulation depth and weak absorption.26 BP is suitable for near-infrared optoelectronic applications due to the layer-dependent bandgap from 0.3 eV to 1.5 eV but has poor stability for its oxidation when exposed to an ambient environment, which will shorten the lifetime of optoelectronic devices.27,28 In addition, to expand the diversity of ultrafast lasers and realize the industrialization applications, we also need to explore new 2D materials with better nonlinear absorption characteristics, facile fabrication, and high stability.
In the past few years, perovskites have also been incorporated into fiber lasers as the SA to generate ultrashort pulses in fiber laser systems, emerging as a promising candidate for novel optoelectronic devices.29–32 As a representative of the perovskite family, lead halide perovskite nanomaterials have attracted tremendous attention for many appealing properties,33–36 such as high carrier mobility,37 tunable bandgaps,38 high photoluminescence quantum yields, and long carrier diffusion length,39 which sprung them into a plethora of optoelectronic applications including LEDs,40,41 solar cells,42 PDs,43 nanolasers,44 and other optoelectronic devices.45 However, the toxicity of lead-based perovskites and instability against moisture, air, heat, and irradiation have caused significant concern to the ecological environment and human health, inhibiting the large-scale commercial and scientific applications of lead halide perovskites. Hence, considerable endeavors have been dedicated in recent years to investigating a stable, non-toxic lead-free perovskite and its light-emitting and photovoltaic applications.46 Recently, ternary copper halide CsCu2I3, as a member of the perovskite family, has been found to overcome the defects of lead halide perovskite nanomaterials,47 due to the superior optoelectronic performance including longer carrier diffusion lengths, lower trap densities, non-toxicity and high thermal and moisture stability, showing great potential for industrialization of optoelectronic applications.48–52 Hence, the research on CsCu2I3 has recently become a focus. Despite significant advances based on CsCu2I3 focused on LEDs, PDs, and solar cells,53–55 the exploration of CsCu2I3 in ultrafast photonics is still rare.
Recently, perovskite-based SAs have been applied in Q-switching and mode-locking fiber lasers.31,56–59 Li et al. found that organic–inorganic halide perovskite CH3NH3PbI3 exhibited a larger nonlinear absorption coefficient and a low saturable intensity, and demonstrated an ultrafast picosecond pulsed fiber laser based on CH3NH3PbI3-SA.56 Similarly, the SA has also been employed in the erbium-doped fiber (EDF) laser cavity.57,58 Based on the metal halide perovskite CsPbBr3 quantum dots, Liu et al. obtained stable dissipative soliton pulses in the EDF laser at 1.6 μm.59 These advances have indicated that perovskite nanomaterials have been developed as an effective SA for ultrafast photonics.
Moreover, the fabrication of CsCu2I3 micro-rods is a low cost, simple process, and is environmentally friendly, which is more favorable for practical applications. Compared with bulk materials and large films, the morphology of micro-nano columns has unique advantages in optical fiber coupling. Therefore, based on its exceptional properties, CsCu2I3 can be a good choice as a SA to improve the stability of the fiber laser. Here, a CsCu2I3-based SA was fabricated by a solvent evaporation crystallization method. The CsCu2I3 micro-rods as an optoelectronic device are simulated by DFT calculations, which elucidated their superior thermodynamic stability and broadband response. Meanwhile, the CsCu2I3-based nonlinear test showed a modulation depth of 12.04%. These results demonstrate the feasibility of considering CsCu2I3 micro-rods as a SA in fiber laser systems. A passively mode-locked fiber laser operating at the communication band is successfully demonstrated. It is worth noting that the fiber laser can operate steadily for at least five months, indicating that the device has ultra-high stability. This research will potentially open up a new pathway for ultrafast photonic applications based on lead-free halide perovskites and provide the possibility for industrial and scientific applications.
Synthesis and characterization of CsCu2I3 micro-rods
Synthesis of CsCu2I3 micro-rods
Preparation of CsCu2I3 precursor solution: the precursor solution was prepared by dissolving 0.13 g CuI (99.9%, Aladdin) and 0.19 g CsI (99.95%, Aladdin) in 1 mL N,N-dimethylformamide (DMF, 99.8%, Aladdin). After heating the mixed solution to 50 °C and holding it for about 12 hours, the precursor solution was filtered by a polytetrafluoroethylene (PTFE) filter (0.22 μm) to get rid of the undissolved residuum.
Preparation of the CsCu2I3 micro-rods: at first, a silicon slice was used as a substrate (as shown in Fig. S1, ESI†), which was cleaned by the ultrasonic method with acetone, ethanol, and deionized water, successively. A drop of CsCu2I3 precursor solution (5 μL) was dropped onto the silicon substrate through a pipette. Then, this substrate was placed on a heating table at ∼50 °C. During the heating process, with the gradual evaporation of the solvent, the solution gradually reached the supersaturated state and the CsCu2I3 crystals precipitated. After 20–30 min, the solvent evaporated completely and a large number of rod-like structures appeared on the silicon substrate. As schematically shown in Fig. S1 (ESI†), the crystal structure of CsCu2I3 is formed with the one-dimensional in-line double chains of [Cu2I3]− along the c axis in which the octahedron [CuI4]3− tetrahedral shared common edges. These chain-like structures are surrounded and isolated by Cs+ cations.
Characterization of CsCu2I3 micro-rods
The crystallinity of the samples was confirmed by the X-ray diffraction (XRD) measurements (see Fig. 1(a)). The XRD pattern shows the diffraction peaks of (110), (020), (220), (040), (330), (060), (350), (242), and (080), which match well with the orthorhombic CsCu2I3 (space group Cmcm, JCPDS, No. 77-0069), indicating that the sample was pure. Additionally, the relatively narrow diffraction peaks also suggest the high crystallization quality of the sample. X-Ray photoelectron spectroscopy (XPS) measurements were performed to verify the elemental composition of the obtained sample. The presence of cesium, copper, and iodine is clearly discernible in the survey XPS spectra (as shown in Fig. 1(b)). The high-resolution XPS spectra of the three elements are displayed in Fig. 1(c–e). There are two peaks at 724.8 and 738.8 eV corresponding to Cs 3d5/2 and Cs 3d3/2, and the difference between the two peaks is also consistent with the spin–orbit components (14 eV). From the XPS of Cu 2p, the two spin–orbit peaks located at 932.8 and 952.7 eV without any satellite peaks distinctly reveal the presence of Cu+ rather than Cu2+ in CsCu2I3. For the I 3d spectrum, the two spin–orbit peaks of I at 619.7 and 631.2 eV correspond to I 3d5/2 and I 3d3/2, respectively, which agrees well with other iodide perovskites previously reported. Fig. 1(f) shows the high magnification scanning electron microscopic (SEM) image of CsCu2I3 micro-rods, demonstrating a relatively smooth surface. Transmission electron microscopy (TEM) was utilized to further investigate the crystal structure of the CsCu2I3 micro-rods. Fig. 1(g) displays a low-magnification TEM image of a fragment of CsCu2I3 micro-rods transferred onto a copper grid. The corresponding selected area electron diffraction (SAED) patterns in Fig. 1(h) depict an unambiguous diffraction spot which indicates the single-crystalline properties of the CsCu2I3 micro-rods. The diffraction spots are confirmed as (200) and (021), which further verify the orthorhombic structure of CsCu2I3 rods. The SEM image and energy-dispersive X-ray spectroscopy (EDS) test of the CsCu2I3 micro-rods are shown in Fig. S2 (ESI†). The Raman spectrum of the CsCu2I3 micro-rods shows a characteristic peak at ∼116 cm−1, which corresponds to the phonon mode of the I–Cu–I vibration (shown in Fig. S3, ESI†). All these results above demonstrate that the as-grown CsCu2I3 micro-rods prepared by the solvent evaporation crystallization method are of good quality.
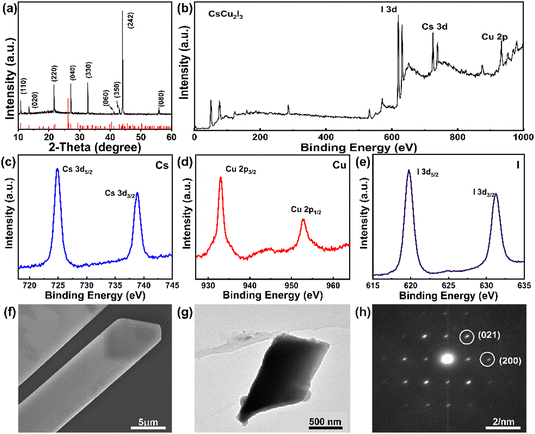 |
| Fig. 1 Characteristics of CsCu2I3 crystals. (a) XRD pattern. (b) Total XPS spectrum. (c–e) High-resolution XPS spectra of Cs, Cu, and I, respectively. (f) High magnification SEM image. (g) TEM image. (h) Corresponding SEAD image. | |
Characteristic evaluation using DFT calculations
In the fiber laser system, as the light passes through the SA, the process of saturating the material with absorbing photons inevitably produces thermal effects. Therefore, the thermodynamic stability of CsCu2I3 at 300–1500 K is first investigated as shown in Fig. 2(a). It's obvious that the average potential energy of the system within 6 ps maintains −48
000 eV at 300 K-600 K, and there is a dramatic decrease in potential energy at 700 K and it levels off at −74
000 eV as the temperature increases. Although the system's potential energy is certainly affected by the environmental temperature, the standard deviation of the potential energy variation of CsCu2I3 within 6 ps at different temperatures remained between 0 and 0.5, demonstrating the stability of CsCu2I3 as a SA (for more details see Fig. S5, ESI†).
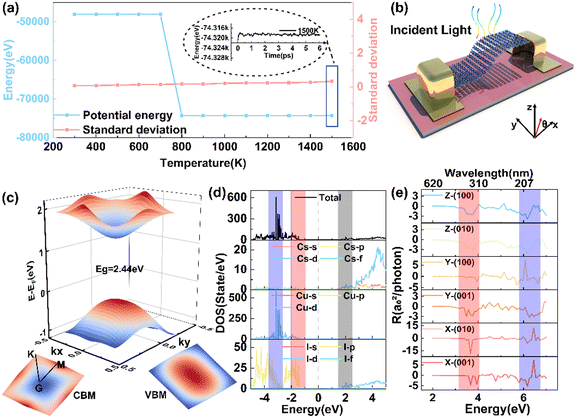 |
| Fig. 2 Thermodynamic stability and electronic band structure of CsCu2I3. (a) Average system potential energy and standard deviation of energy change within 6 ps for CsCu2I3 at different temperatures. Right inset: The variation of the potential energy of the system at 1500 K. (b) CsCu2I3-based optoelectronic device model. (c) 3D views of the CBM and VBM. (d) The density of states of CsCu2I3 and the contribution of different atomic electron orbitals to the density of states. (e) Photocurrents in different directions (X, Y, and Z) for CsCu2I3 with different polarization light ((001), (010)). | |
In the meantime, the performance of CsCu2I3 as an optoelectronic device needs further assessment. The constructed model of the CsCu2I3-based optoelectronic device is shown in Fig. 2(b). The CsCu2I3 optoelectronic device model consists of three parts, including the left electrode, the central area, and the right electrode. An external bias is applied through the two metal electrodes and the current is measured. When light is irradiated on a material, photons excite the electrons in the valence band to leap to the conduction band, forming a photocurrent. The photocurrent in each direction under different surface irradiation is studied as shown in Fig. 2(e). It can be seen that there is a continuous absorption peak from the visible to the ultraviolet band, indicating that CsCu2I3 as a photovoltaic material is capable of a broadband response. And to explain the cause of this phenomenon, the electron leap process behind it is analyzed. The electronic band structure of CsCu2I3 indicates that the conduction band minimum (CBM) and valence band maximum (VBM) are both located in the G point as shown in Fig. 2(c) and Fig. S6 (ESI†), and the direct gap promotes the effective electron leap. Furthermore, large photocurrents are generated under photon excitation at around 4 eV (red shaded zone) and 6 eV (blue shaded zone) in Fig. 2(e), which can be interpreted in terms of electron transitions between different electron orbitals as shown in Fig. 2(d). The photocurrent peaks in the low energy region are derived from a leap where the electronic states in the red-shaded zone are excited to the ones in the grey-shaded zone. Whereas the photocurrent peaks in the high energy region originate from a transition where the electronic states in the blue-shaded region are excited to the one in the grey-shaded region. The electrons in the blue-shaded zone are significantly more than in the red-shaded zone leading to a larger photocurrent in the high-energy region. The excited electronic state at the valence band is dominated by the d orbital of Cu and the p orbital of I, while the electronic state occupied at the conduction band originates from the d orbital of Cs. Due to the selection rule for the electromagnetic transitions, electrons in the p orbitals are more likely to leap to the d orbitals thus enhancing the absorption of photons. On the other hand, in fiber laser systems, there are more electronic states at the valence band of CsCu2I3 than at the conduction band, which leads to rapid saturation of CsCu2I3 as the SA, favoring the output of the pulse.
Experimental setup and results
Nonlinear optical properties
Considering that the synthesis method of high-quality CsCu2I3 micro-rods mentioned above is efficient and simple, we apply it to the SA device. As related to the previous method and the schematic diagram (Fig. S1, ESI†), a drop of precursor solution (0.5 μL was dropped onto the fiber end-facet through a pipette. Then, the whole device was dried at 50 °C for 20 min, and the CsCu2I3 micro-rods appeared on the fiber end-facet of the optic patch cable (SMJ-1315-0.9-0.5m-FU/FU, insertion loss <0.15 dB, return loss >50 dB). After that, the patch cable with CsCu2I3 micro-rods was connected with a similarly clean one by a terminated fiber adapter. Finally, the SA used in the laser system was successfully prepared. To guarantee that all light in the optical fiber passes through micro-rods, and adjust the mode-locked state conveniently, the material prepared SA must completely cover the core. Fig. S7 (ESI†) indicated that the core of the fiber cable was covered by the CsCu2I3 micro-rods completely.
The nonlinear optical characteristics of the CsCu2I3 micro-rods were investigated at 1550 nm (photon energy smaller than the bandgap of CsCu2I3) by the balanced twin-detector technique, and the experimental device is shown in Fig. S8(a) (ESI†), where the laser source operated at 1550 nm with a repetition rate of 16.6 MHz and a pulse duration of 448 fs. The corresponding optical transmittance curve of the CsCu2I3 micro-rods was acquired by the following formula:
| 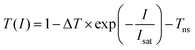 | (1) |
Here, T(I) refers to the transmittance regarding the input intensity (I), ΔT represents the modulation depth, Isat represents the non-saturable intensity, and Tns represents the non-saturable loss. The experimental data is displayed in Fig. 3(a), showing that as the incident intensity increases, the transmittance increases gradually and then tends to be saturated, showing a typical saturated absorption response. This might be associated with the susceptibility of materials to lattice defects, leading to optical transition, and band filling of intermediate or defective states.60,61 Hence, the electronic band structures of CsCu2I3 with Cs, Cu, and I atomic defects are simulated by DFT (as shown in Fig. S9 and S10, ESI†). It's obvious that the VBM of CsCu2I3 shifts upwards and crosses the Fermi energy level at high solubility defects, and the electronic structure undergoes a transition from semiconductor to metallic behavior. This also supports the application of CsCu2I3 as SA cooperated into 1.5 μm fiber laser systems. According to eqn (1), by fitting the experimental data in Fig. 3(a), the values of modulation depth, saturated intensity, and non-saturable loss were ∼ 12.04%, ∼0.19 MW cm−2, and 84.31%, respectively. Therefore, based on the experimental data, the maximum peak power intensity passing through the CsCu2I3 SA was about 4.089 GW cm−2, and we did not find any optical damage on the CsCu2I3 SA, which indicates that the damage threshold of CsCu2I3 for an ultrafast laser should be larger than 4.089 GW cm−2. Besides, we further investigated the nonlinear optical properties of CsCu2I3 micro-rods at the wavelengths of 1060 nm and 1950 nm by the same method. The corresponding results are shown in Fig. S8(b and c) (ESI†). The modulation depth is about 7.58% at 1 μm and 7.07% at 2 μm, which illustrates that the CsCu2I3 micro-rods have great potential for ultrafast photonics.
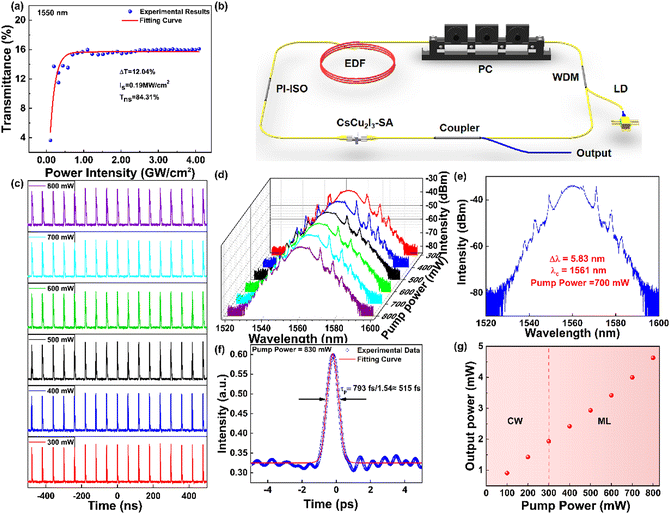 |
| Fig. 3 Characterization of a mode-locked laser based on the CsCu2I3-SA. (a) The nonlinear test and fitting results. (b) Configuration of the mode-locking fiber laser. (c and d) Pulse trains and spectrum under different pump powers. (e) Single spectrum under a pump power of 700 mW. (f) Typical autocorrelation trajectory of output pulse at a pump power of 830 mW. (g) Average output power versus pump power. | |
Fiber laser configuration
The experimental setup of the femtosecond pulse EDF laser based on the CsCu2I3-SA is shown in Fig. 3(b). The total length of the cavity is about 12 m with 2.14 m of EDF as the gain medium and 9.86 m of standard single-mode fiber (SMF). The adopted pump source was a 976 nm laser diode (LD, maximum output power of 830 mW), then coupled to the EDF via a 980/1550 nm wavelength-division multiplexer (WDM). The polarization controller (PC) was placed between the EDF and the WDM, which can be utilized to manipulate the polarization state in the cavity. A polarization-insensitive isolator (PI-ISO) was inserted after the EDF to ensure unidirectional propagation in case of the backward light's damage. The CsCu2I3-SA was placed between the ISO and an optical coupler (OC). Besides, the OC (a 20
:
80 ratio) was employed to extract the intra-cavity energy with an 80% portion of laser passed through the CsCu2I3-SA and a 20% portion of laser coupled out from the cavity to measure the characteristics of the mode-locked fiber laser. The dispersion coefficients of SMF and EDF are −22 ps2 km−1 and 28 ps2 km−1, respectively, so the total dispersion is about −0.157 ps2.
Results and discussion
On the basis of the aforementioned experimental device, the pulse experiment platform was established. First, the ultrashort pulse was not produced without the CsCu2I3-SA, when adjusting the LD pump power from 0 to 830 mW and PC in the whole range. Hence, this clearly indicates that CsCu2I3-SA is the crucial component for pulse generation, and the nonlinear polarization rotation (NPR) effect in the resonator is not enough to generate the ultrashort pulse, only the continuous-wave emission, regardless of the pump power and the PC orientation. When the fabricated CsCu2I3-SA was inserted into the cavity, mode-locked pulses were acquired when adjusting the pump power in the range of 300–800 mW at an appropriate polarization state. Fig. 3(c) displays the pulse trains under different pump powers, and the adjacent pulse train interval is about 59.8 ns, corresponding to the ring cavity length of 12 m, and a repetition rate of ∼16.7 MHz. The optical spectrum in the range of 300 mW to 800 mW is shown in Fig. 3(d). We can see the spectrum is quite stable at different pump powers. The corresponding spectrum in stable mode-locking operation shows multistage Kelly sidebands, indicating the pulse is a dispersion-managed soliton. A typical spectrum (as shown in Fig. 3(e)) under a pump power of 700 mW, displays a central wavelength at about 1561 nm and the 3 dB bandwidth at about 5.83 nm. The autocorrelation trace in Fig. 3(f) indicates that single soliton is generated inside the cavity, and the FWHM is about 515 fs, in the case of the incident power set at 830 mW. Fig. 3(g) depicts the output power related to the incident pump power increased monotonically and linearly, implying that the mode-locking fiber laser was stable, and the continuous wave and mode-locking operation are divided by a red dotted line. Hence, according to the data mentioned above, the maximum pulse energy was about 0.28 nJ.
Detailed characteristics of the output pulses are summarized in Fig. 4 with respect to the pump power of 700 mW. The wide-band radio frequency (RF) spectrum is shown in Fig. 4(a), without spurious sidebands in the range of 0–500 MHz, which further manifests the relatively high spectral purity of the mode-locking fiber laser. Moreover, within 0–50 MHz, Fig. 4(b) reveals that the SNR is ∼49 dB measured at 16.7 MHz with a resolution bandwidth of 500 Hz. Fig. 4(c) displays the pump power-dependent central wavelength (CW) and 3 dB bandwidth (Δλ). The central wavelength decreases slightly from 1562 nm to 1561 nm with the pump power increasing, indicating the CW has a blue shift. Simultaneously, the 3 dB bandwidth increases from 5.72 nm to 6.01 nm. Fig. 4(d) illustrates the repetition rate is 16.7 MHz and the pulse train interval is 59.8 ns, indicating there were almost no changes with different pump power. Hence, the foregoing data demonstrates that the laser has good performance.
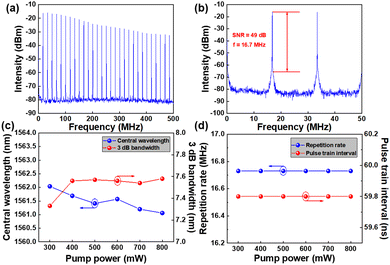 |
| Fig. 4 Performance of the mode-locking laser based on the CsCu2I3-SA. (a) Recorded RF with a wide span. (b) Narrowband RF Spectrum. (c) Central wavelength and 3 dB bandwidth and (d) repetition rate and pulse train interval as a function of pump power. | |
The long-term operational performance of a fiber laser is particularly significant for industrial and scientific applications. Therefore, we have examined the stability of the CsCu2I3-based mode-locked laser for over 164 days. Fig. 5 displays the general stability test results under a pump power of 700 mW or 800 mW. In the laboratory environment, the pulse laser could run steadily for over five months. As shown in Fig. 5(a), the laser spectrum was relatively stable, as the central wavelength varied slowly and the 3 dB bandwidth fluctuated slightly around 5.8 nm, showing a slow rise tendency over time. In Fig. 5(b), the repetition rate has remained unchanged at 16.7 MHz for over five months. The variation of output power and pulse train interval from the average values (Fig. 5(c and d)) were less than 2.6%, and 0.33%, respectively, which shows the laser was very stable within five months. The stability of a SA in ambient air or moisture plays a vital role in the long-life span fiber laser system. Here, the main reasons for the improvement of stability are as follows. On the one hand, according to the reported results,49,62 Roccanova and Yang et al. have demonstrated the CsCu2I3 film exhibited good ambient air stability for at least 60 days. Meanwhile, by DFT calculation, we have evaluated the thermal stability at 300–1500 K. Consequently, CsCu2I3 micro-rods possess good moisture, thermal, and long-term stability. CsCu2I3-SA, on the other hand, was encapsulated in two fiber optic patch cables, weakening the interaction between CsCu2I3-SA and air or moisture, and further enhancing its long-term stability. So far, as we know, our pulse laser based on CsCu2I3-SA shows super stability compared with related results previously reported.
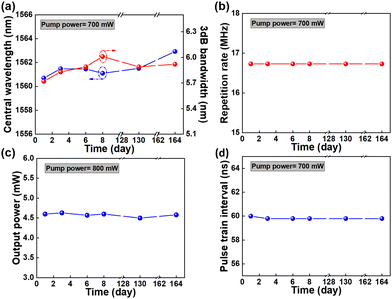 |
| Fig. 5 The robust performance of the mode-locked laser based on CsCu2I3-SA. The changes of (a) the central wavelength and 3 dB bandwidth, (b) the repetition rate, (c) the output power, and (d) the pulse train interval within 164 days under the same pump power. | |
Conclusion
In conclusion, we obtained lead-free perovskite CsCu2I3 micro-rods by a solvent evaporation crystallization method. By first-principles calculations, we have theoretically demonstrated excellent thermal stability and elucidated the performance of lead-free perovskite CsCu2I3 micro-rods as an optoelectronic device. As a SA in the fiber laser system, the CsCu2I3 micro-rods exhibited remarkable nonlinear optical properties with an estimated modulation depth of 12.04%. Hence, a passively mode-locked erbium-doped fiber laser operating at the communication band was proposed with a FWHM of 515 fs at a repetition rate of 16.7 MHz and a SNR of 49 dB. Then we experimentally demonstrated that the fiber laser possessed high stability, and can operate steadily for at least five months. This research will potentially unlock the pathways for ultrafast photonic and optoelectronic devices based on lead-free halide perovskites.
Author contributions
Haiqin Deng performed the experiments and data processing and wrote the manuscript. Xing Xu synthesized the materials, analysed the TEM, and drew the schematic diagram. Fangqi Liu demonstrated excellent thermal stability and the electronic band structure of CsCu2I3 by first-principles calculations. Qiang Yu carried out the characterization and analysis of SEM and EDS. Bowang Shu and Zixing Yang revised a part of the manuscript. Sicong Zhu and Jian Wu proposed the idea. Qinglin Zhang and Pu Zhou supervised the project.
Conflicts of interest
The authors have no conflicts to disclose.
Acknowledgements
This work was financially supported by the National Natural Science Foundation of China (Grant No. 51772088). The first three authors H. Q. D., X. X., and F. Q. L. contributed equally to this work.
Notes and references
- X. Hong, J. Kim, S. Shi, Y. Zhang, C. Jin, Y. Sun, S. Tongay, J. Wu, Y. Zhang and F. Wang, Nat. Nanotechnol., 2014, 9, 682–686 CrossRef CAS
.
- C. Kerse, H. Kalaycıoğlu, P. Elahi, B. Çetin, D. K. Kesim, Ö. Akçaalan, S. Yavaş, M. D. Aşık, B. Öktem, H. Hoogland, R. Holzwarth and F. Ö. Ilday, Nature, 2016, 537, 84–88 CrossRef CAS
.
- N. G. Horton, K. Wang, D. Kobat, C. G. Clark, F. W. Wise, C. B. Schaffer and C. Xu, Nat. Photonics, 2013, 7, 205–209 CrossRef CAS
.
- E. Agrell, M. Karlsson, A. R. Chraplyvy, D. J. Richardson, P. M. Krummrich, P. Winzer, K. Roberts, J. K. Fischer, S. J. Savory, B. J. Eggleton, M. Secondini, F. R. Kschischang, A. Lord, J. Prat, I. Tomkos, J. E. Bowers, S. Srinivasan, M. Brandt-Pearce and N. Gisin, J. Opt., 2016, 18, 063002 CrossRef
.
- D. A. Stoliarov, P. A. Itrin, D. A. Korobko, V. A. Ribenek, L. V. Tabulina, A. V. Sysa and Y. P. Shaman, Opt. Fiber Technol., 2021, 63, 102524 CrossRef CAS
.
- C. Jiang, X. Wang, H. Wang, S. Wang, L. Qin, J. Liu and Z. Zhang, Adv. Photonics Res., 2022, 3, 2100183 CrossRef
.
- J. Lan, J. Qiao, W. Sung, C. Chen, R. Jhang, S. Lin, L. Ng, G. Liang, M. Wu, L. Tu, C. Cheng, H. Liu and C. Lee, Nanoscale, 2020, 12, 16956–16966 RSC
.
- J. Lan, C. Yang, C. Chen, C. Cheng, H. Liu and C. Lee, J. Mater. Chem. C, 2022, 10, 9017–9024 RSC
.
- M. Pawliszewska, A. Dużyńska, M. Zdrojek and J. Sotor, Opt. Express, 2019, 27, 11361–11369 CrossRef CAS PubMed
.
- J. Wang, Y. Chen and W. J. Blau, J. Mater. Chem., 2009, 19, 7425–7443 RSC
.
- K. K. Chow, S. Yamashita and Y. W. Song, Opt. Express, 2009, 17, 7664–7669 CrossRef CAS
.
- P. Tang, X. Zhang, C. Zhao, Y. Wang, H. Zhang, D. Shen, S. Wen, D. Tang and D. Fan, IEEE Photonics J., 2013, 5, 1500707 Search PubMed
.
- T. Wang, Q. Yu, K. Guo, X. Shi, X. Kan, Y. Xu, J. Wu, K. Zhang and P. Zhou, Front. Inf. Technol. Electron. Eng., 2021, 22, 287–295 CrossRef
.
- B. Guo, Y. Yao, Y. Yang, Y. Yuan, L. Jin, B. Yan and J. Zhang, Photonics Res., 2015, 3, 94–99 CrossRef CAS
.
- W. Zhou, X. Pang, H. Zhang, Q. Yu, F. Liu, W. Wang, Y. Zhao, Y. Lu and Z. Yang, Materials, 2022, 15, 6761 CrossRef CAS
.
- S. Lv, X. Liu, X. Li, W. Luo, W. Xu, Z. Shi, Y. Ren, C. Zhang and K. Zhang, ACS Appl. Mater. Interfaces, 2020, 12, 43049–43057 CrossRef CAS PubMed
.
- Q. Yu, S. Wang, Y. Zhang, Z. Dong, H. Deng, K. Guo, T. Wang, X. Shi, F. Liu, T. Xian, S. Zhu, J. Wu, Z. Zhang, K. Zhang and L. Zhan, Nanoscale, 2021, 13, 20471–20480 RSC
.
- X. Li, J. Feng, W. Mao, F. Yin and J. Jiang, J. Mater. Chem. C, 2020, 8, 14386–14392 RSC
.
- Y. Feng, L. Du, Y. He, Q. Yi, J. Li, Q. Duan, S. Chen, L. Miao and C. Zhao, J. Mater. Chem. C, 2021, 9, 6445–6451 RSC
.
- Q. Yu, K. Guo, Y. Dai, H. Deng, T. Wang, H. Wu, Y. Xu, X. Shi, J. Wu, K. Zhang and P. Zhou, J. Phys.: Condens. Matter, 2021, 33, 503001 CrossRef CAS
.
- B. Guo, Q. L. Xiao, S. H. Wang and H. Zhang, Laser Photonics Rev., 2019, 13, 1800327 CrossRef CAS
.
- Z. Ma, H. Zhang, Z. Hu, J. Gan, C. Yang, Z. Luo, T. Qiao, M. Peng, G. Dong, Z. Yang, F. W. Wise and J. Qiu, J. Mater. Chem. C, 2018, 6, 1126–1135 RSC
.
- B. Guo, S. Wang, Z. Wu, Z. Wang, D.-H. Wang, H. Huang, F. Zhang, Y. Ge and H. Zhang, Opt. Express, 2018, 26, 22750–22760 CrossRef CAS
.
- J. Wang, T. Wang, X. Shi, J. Wu, Y. Xu, X. Ding, Q. Yu, K. Zhang, P. Zhou and Z. Jiang, J. Mater. Chem. C, 2019, 7, 14625–14631 RSC
.
- T. Chai, X. Li, T. Feng, P. Guo, Y. Song, Y. Chen and H. Zhang, Nanoscale, 2018, 10, 17617–17622 RSC
.
- B. Zhang, J. Liu, C. Wang, K. Yang, C. Lee, H. Zhang and J. He, Laser Photonics Rev., 2020, 14, 1900240 CrossRef CAS
.
- M. Tuo, C. Xu, H. Mu, X. Bao, Y. Wang, S. Xiao, W. Ma, L. Li, D. Tang, H. Zhang, M. Premaratne, B. Sun, H.-M. Cheng, S. Li, W. Ren and Q. Bao, ACS Photonics, 2018, 5, 1808–1816 CrossRef CAS
.
- Z. Xie, F. Zhang, Z. Liang, T. Fan, Z. Li, X. Jiang, H. Chen, J. Li and H. Zhang, Photonics Res., 2019, 7, 494–502 CrossRef CAS
.
- B. Huang, J. Yi, G. Jiang, L. Miao, W. Hu, C. Zhao and S. Wen, Opt. Mater. Express, 2017, 7, 1220–1227 CrossRef CAS
.
- J. Wang, Y. Wang, Z. Chen, X. Yang and R. Lv, J. Mater. Chem. C, 2019, 7, 5047–5050 RSC
.
- S. Yang, J. Li, L. Li, L. Zhang and X. Zhang, J. Mater. Chem. C, 2022, 10, 7504–7510 RSC
.
- S. Hong, F. Lédée, J. Park, S. Song, H. Lee, Y. S. Lee, B. Kim, D.-I. Yeom, E. Deleporte and K. Oh, Laser Photonics Rev., 2018, 12, 1800118 CrossRef
.
- M. L. Aubrey, A. Saldivar Valdes, M. R. Filip, B. A. Connor, K. P. Lindquist, J. B. Neaton and H. I. Karunadasa, Nature, 2021, 597, 355–359 CrossRef CAS
.
- M. Liu, Z. Wei, A. Luo, W. Xu and Z. Luo, Nanophotonics, 2020, 9, 2641–2671 CrossRef
.
- K. Sun, D. Tan, X. Fang, X. Xia, D. Lin, J. Song, Y. Lin, Z. Liu, M. Gu, Y. Yue and J. Qiu, Science, 2022, 375, 307–310 CrossRef CAS
.
- D. Marongiu, M. Saba, F. Quochi, A. Mura and G. Bongiovanni, J. Mater. Chem. C, 2019, 7, 12006–12018 RSC
.
- F. Yang, A. Wang, S. Yue, W. Du, S. Wang, X. Zhang and X. Liu, Sci. China Mater., 2021, 64, 2889–2914 CrossRef CAS
.
- G. Xing, N. Mathews, S. S. Lim, N. Yantara, X. Liu, D. Sabba, M. Grätzel, S. Mhaisalkar and T. C. Sum, Nat. Mater., 2014, 13, 476–480 CrossRef CAS
.
- K. Wei, T. Jiang, Z. Xu, J. Zhou, J. You, Y. Tang, H. Li, R. Chen, X. Zheng, S. Wang, K. Yin, Z. Wang, J. Wang and X. Cheng, Laser Photonics Rev., 2018, 12, 1800128 CrossRef
.
- S. Liu, Y. Yue, X. Zhang, C. Wang, G. Yang and D. Zhu, J. Mater. Chem. C, 2020, 8, 8374–8379 RSC
.
- X. Luo, T. Zheng, Z. Luo, J. Liu, S. Deng, R. Chen, M. Zhang, H. S. Kwok, J. Zhang and G. Li, ACS Photonics, 2021, 8, 3337–3345 CrossRef CAS
.
- S. Tombe, G. Adam, H. Heilbrunner, D. H. Apaydin, C. Ulbricht, N. S. Sariciftci, C. J. Arendse, E. Iwuoha and M. C. Scharber, J. Mater. Chem. C, 2017, 5, 1714–1723 RSC
.
- C. Peng, Q. Wei, L. Chen, R. Zeng, Q. Zhang, Q. Hu and B. Zou, J. Mater. Chem. C, 2021, 9, 15522–15529 RSC
.
- S. Li, D. Lei, W. Ren, X. Guo, S. Wu, Y. Zhu, A. L. Rogach, M. Chhowalla and A. K. Y. Jen, Nat. Commun., 2020, 11, 1192 CrossRef CAS PubMed
.
- T. Song, Q. Ma, Q. Wang and H. Zhang, Mater. Adv., 2022, 3, 756–778 RSC
.
- R. Khazaeinezhad, S. Hosseinzadeh Kassani, B. Paulson, H. Jeong, J. Gwak, F. Rotermund, D. Yeom and K. Oh, Sci. Rep., 2017, 7, 41480 CrossRef CAS PubMed
.
- X. Xu, C. Fan, Z. Qi, S. Jiang, Q. Xiao, H. Liang, H. Duan and Q. Zhang, ACS Appl. Nano Mater., 2021, 4, 9625–9634 CrossRef CAS
.
- C. Zhou, T. Zhang, C. Zhang, X. Liu, J. Wang, J. Lin and X. Chen, Adv. Sci., 2022, 9, 2103491 CrossRef CAS
.
- R. Roccanova, A. Yangui, G. Seo, T. D. Creason, Y. Wu, D. Y. Kim, M. Du and B. Saparov, ACS Mater. Lett., 2019, 1, 459–465 CrossRef CAS
.
- X. Cheng, S. Yang, B. Cao, X. Tao and Z. Chen, Adv. Funct. Mater., 2020, 30, 1905021 CrossRef CAS
.
- X. Wang, T. Zhang, Y. Lou and Y. Zhao, Mater. Chem. Front., 2019, 3, 365–375 RSC
.
- R. Lin, Q. Guo, Q. Zhu, Y. Zhu, W. Zheng and F. Huang, Adv. Mater., 2019, 31, 1905079 CrossRef CAS
.
- F. Sun, T. Liu, P. Ran, X. Chen, T. Jiang, W. Shen, X. Liu and Y. M. Yang, J. Phys. Chem. Lett., 2022, 13, 3431–3437 CrossRef CAS PubMed
.
- X. Xu, S. Jiang, C. Fan, Q. Deng, L. Shen and Q. Zhang, Adv. Opt. Mater., 2022, 2201107 CrossRef CAS
.
- Y. Gu, X. Yao, H. Geng, G. Guan, M. Hu and M. Han, ACS Appl. Mater. Interfaces, 2021, 13, 40798–40805 CrossRef
.
- P. Li, Y. Chen, T. Yang, Z. Wang, H. Lin, Y. Xu, L. Li, H. Mu, B. N. Shivananju, Y. Zhang, Q. Zhang, A. Pan, S. Li, D. Tang, B. Jia, H. Zhang and Q. Bao, ACS Appl. Mater. Interfaces, 2017, 9, 12759–12765 CrossRef CAS
.
- L. Miao, G. Jiang, L. Du, B. Huang, W. Hu, C. Zhao and S. Wen, IEEE Photonics Technol. Lett., 2018, 30, 1 Search PubMed
.
- G. Jiang, L. Miao, J. Yi, B. Huang, W. Peng, Y. Zou, H. Huang, W. Hu, C. Zhao and S. Wen, Appl. Phys. Lett., 2017, 110, 161111 CrossRef
.
- B. Liu, L. Gao, W. W. Cheng, X. S. Tang, C. Gao, Y. L. Cao, Y. J. Li and T. Zhu, Opt. Express, 2018, 26, 7155–7162 CrossRef CAS
.
- S. Das, Y. Wang, Y. Dai, S. Li and Z. Sun, Light: Sci. Appl., 2021, 10, 27 CrossRef CAS
.
- X. Zhao, X. Yin, D. Liu, C. Huo, G. Dong, S. Chen, Z. Liu, X. Yan and J. Tian, J. Phys. Chem. C, 2022, 126, 6837–6846 CrossRef CAS
.
- J. Yang, W. Kang, Z. Liu, M. Pi, L. Luo, C. Li, H. Lin, Z. Luo, J. Du, M. Zhou and X. Tang, J. Phys. Chem. Lett., 2020, 11, 6880–6886 CrossRef CAS PubMed
.
|
This journal is © The Royal Society of Chemistry 2023 |