DOI:
10.1039/D3TA01373A
(Paper)
J. Mater. Chem. A, 2023,
11, 22922-22940
Unveiling the structure and ion dynamics of amorphous Na3−xOHxCl antiperovskite electrolytes by first-principles molecular dynamics†
Received
6th March 2023
, Accepted 26th September 2023
First published on 27th September 2023
Abstract
Sodium oxyhalide and hydroxyhalide antiperovskites are promising solid-state electrolytes (SSEs) because of their low melting point and rapid synthesis and, as such, they are becoming competitive with respect to other systems. While the structure and the mechanism underlying the ion dynamics are increasingly well understood in crystalline antiperovskites, their amorphous counterpart lacks precise structural characterization, hampering any conclusive insight into their properties. In this work, we resort to first-principles molecular dynamics within the Car–Parrinello scheme to assess the structure and ion dynamics of amorphous Na3−xOHxCl (with x = 0, 0.5, and 1) antiperovskites at a quantitative level. We obtain a detailed structural description of these amorphous systems, unveiling the mechanism inherent to the dynamics of Na ions, the role of H atoms, and the resulting ionic conductivity. Our results demonstrate that the structure of amorphous Na3OCl significantly differs from its crystal phase, showing very limited intermediate-range order and a short-range order mainly driven by four-fold Na atoms. Our results reveal that there is no evidence of phase separation in the amorphous Na3−xOHxCl, unlike the previous conjectured model of glassy Li3OCl. The amorphous structure of Na3OCl features remarkable Na ion dynamics and ionic conductivity, rivaling that of defective crystalline phases and highlighting its potential as a promising solid-state electrolyte. In hydroxylated models, the presence of hydroxyl OH− anions plays a crucial role in the mobility of Na ions. This is facilitated by the rapid rotation of O–H bonds and paddlewheel-type mechanisms, leading to enhanced ion mobility in the amorphous Na3−xOHxCl systems. This work provides unprecedented physical and chemical insight into the interplay between the structure, bonding, and ion transport in amorphous sodium-rich oxyhalide and hydroxyhalide antiperovskites, paving the way to their practical realization in next-generation SSEs.
1 Introduction
Over the past decade, low-melting-point Li- and Na-rich antiperovskite solid electrolytes (SEs) have been targeted worldwide as promising materials for solid-state batteries because of their structural flexibility, high ionic conductivity, wide electrochemical window, and stability.1–5 In particular, sodium oxyhalide and hydroxyhalide antiperovskites hold the potential to be used in the SE domain due to their low melting point (Tm < 300 °C), affordable cost and low environmental impact of the constituent elements (Na, H, O, and Cl), and rapid synthesis.6 By exploiting their versatile structure, expressed as X3OA (X= Li+ or Na+; A = halides (Cl−, Br−, I−) or other anions (BH4− and NO2−)), the properties, performance and ion dynamics mechanisms of antiperovskite SEs can be tailored through chemical doping, defect tuning, and structural manipulation, thereby providing an exploitable multifaceted chemistry.1,7–15 By virtue of aliovalent doping or via mixed hydroxides, Li-rich antiperovskites have been shown to yield “superionic” ion conductivities, i.e. ∼0.1 mS cm−1.6,16 Beyond the stoichiometric X3OA crystalline systems, attention has been drawn to the use of cation- and anion-doped X3OA as well as glassy (or amorphous) antiperovskite electrolytes.7,17,18 Glassy Li3OCl- and Na3OCl-based antiperovskites have been originally exploited by the teams of Braga and Goodenough, showing a glass transition temperature in the range 390–450 K and a conductivity of the order of 10−2 S cm−1 at room temperature.19–21 However, attempts at reproducing the original laboratory synthesis were not conclusive and, to date, the structure and ion conduction mechanism of glassy antiperovskite electrolytes remain unsolved issues.1,17,22–24
The original work of Braga et al.19 was later questioned by Hanghofer et al.,22 who ascribed the high conductivity of Li+ ions to the presence of impurities and the stability of the sample, and the formation of LiCl·H2O to the presence of H2O. The results of ref. 19 have been substantiated by ab initio and classical molecular dynamics (MD) simulations agreeing on the existence of a subnanoscale phase separation of Li3OCl into Li2O and LiCl phases together with an intermixed phase. The formation of such subnanoscale separation was considered to be the promoter of the high conductivity of Li ions as well as a non-negligible Cl ion mobility.25 Choi et al. further characterized by ab initio MD the structural and electrochemical stability of amorphous Li3OCl.26
Successful use of an electrolyte composite was recently reported by Tian et al.27–29 In this application, amorphous Li3OCl was used as a matrix embedding Li6.75La3Zr1.75Ta0.25O12 garnet-type oxide particles exhibiting a high room temperature conductivity of 2.27 × 10−4 S cm−1 and an extremely wide electrochemical stability window up to 10 V. In this particular case, the added value promoted by the amorphous X3OA phase was the ability to act as a binder and filling agent ensuring the formation of an integrated composite SE with a continuous widespread ionic conductive network. The key role of the amorphous phase was also assigned to the excellent affinity to the lithium metal, greatly decreasing the interfacial resistance between the anode and the electrolyte.27–29
A detailed understanding of the atomic structure is a key step to further capture the details of the transport mechanisms behind its conductivity performance. Recent reviews on the use of anti-perovskites for solid-state batteries underlined the limited nature of the available structural characterization and pointed out the severe need for a quantitative structural assessment to avoid any misinterpretation of the correlations between the structure and performances.1,30,31 Overall, the structure and ion dynamics mechanisms are well known for crystalline X3OA compounds. This is not the case for amorphous antiperovskites, especially in both hydrogen-free and hydroxylated phases (e.g., oxyhalide X3OA vs. hydroxyhalide X3−xOHxA).
In view of these considerations, this work relies on predictive atomic-scale modelling, as first-principles molecular dynamics (FPMD), to elucidate the structure and transport properties of amorphous H-free and hydroxylated Na3−xOHxCl systems.32 In terms of structural analysis, we provide a detailed study of both X-ray and neutron structure factors. If the X-ray probe is of high importance, neutron studies are particularly needed in the case of antiperovskite electrolyte investigation, being capable of detecting low-Z elements (or light elements, such as H and Li) that are out of reach of X-ray (synchrotron source) techniques.1,2,17,33
Our paper is organized as follows. Section 2 describes the methodologies and model systems employed within first-principles molecular dynamics. We give details on the production of the amorphous systems allowing for the calculation of static and dynamical properties. A specific subsection is devoted to a set of definitions for structural (disorder degree) and dynamical (ionic diffusion and conductivities) quantities targeted in this work for their potential applications in solid-state electrolytes. Results are presented in Section 3 and separated into two subsections, with the first one (3.1) devoted to structural properties and the second one (3.2) to dynamical properties. The analysis of the structural properties features, firstly, calculated properties in reciprocal and real space (3.1.1 and 3.1.2), namely the structure factors and the pair correlation functions. The following parts are devoted to the analysis of the coordination numbers (3.1.3) and the structural units (3.1.4), with special attention paid to the chemical nature of the various motifs and their variations with the composition. A final part is devoted to the assessment of the structural disorder degree of the studied models (3.1.5).
Subsection 3.2 is organized into three parts, describing respectively the different trends shown by the mean square displacements (3.2.1), a dynamical structural rearrangement involving the hydroxyl group (the “paddlewheel” effects, 3.2.2), and the behavior of the diffusion coefficients (3.2.3) leading to the assessment of the ionic conductivity (3.2.4). Concluding remarks are collected in Section 4.
2 Calculation methodology and models
2.1 Producing Na3−xOHxCl amorphous models at 300 K
The Car–Parrinello (CP) method34 was used to produce dynamical trajectories of the targeted Na3−xOHxCl systems. The exchange–correlation functional selected is the generalized gradient approximation (GGA) proposed by Perdew, Burke and Ernzerhof (PBE).35 The valence–core interaction was described by numerical norm-conserving Troullier–Martins (TM)36 pseudopotentials for all elements (Na, O, H, and Cl). In the case of Na, semi-core states were included to ensure a good description of the energetics and electronic features dependent on its cationic nature. This amounts to electronic configurations He 2s2, 2p6, 3s1 for Na; He 2s2, 2p4 for O; 1s1 for H and Ne 3s2, 3p5 for Cl. Valence electrons are treated explicitly and represented on a plane-wave basis set with the sampling of the Brillouin zone restricted to the Γ point. A fictitious electron mass of 600 a.u. and a time step of 0.12 fs ensured optimal conservation of the constants of motion. FPMD simulations were performed in a canonical NVT ensemble with the ionic temperature controlled with a Nosé–Hoover37–39 thermostat chain.40 For the simulations performed at high temperature (>1000 K) we used a Blöchl–Parrinello electronic thermostat41 with a target kinetic energy of 0.1 a.u. to control the fictitious motion of the electronic degrees of freedom. Our FPMD approach34,42 has been extensively used and benchmarked in the last few decades and contributed to the improvement of our understanding of both the physical and chemical properties of chalcogenide and chalcohalide glasses,43–45 hybrid46,47 and porous48 systems, and nanomaterials.49–52
The Na3OCl glass model was generated by quenching from the melt. The initial configuration consisted of a Na3OCl crystal cubic unit cell replicated 3 × 3 × 3 times to obtain a 135 atom model (81 Na, 27 O, and 27 Cl) in a cubic simulation cell of side length 13.6148 Å.53 The initial box was expanded by 15% with respect to crystal density in order to ease melting at a high temperature. A similar approach was followed for the case of glassy Li3OCl.25 Periodic boundary conditions were applied throughout. The model underwent a thermal cycle via canonical NVT simulations, according to the following protocol: 1.3 ps at T = 300 K, 1.2 ps at T = 1200 K, 3.1 ps at T = 1500 K, 46.5 ps at T = 1800 K, 38.6 ps at T = 1000 K, 33.7 ps at T = 750 K and 35 ps at T = 300 K (see Fig. 1). At the end of the cycle of T = 300 K, the density of the system was set to 2.04 g cm−3 (corresponding to a final volume expansion of +5% with respect to the crystal phase) in order to reduce the stress tensor to values very close to 0 GPa. Along the thermal cycle the energy cutoff for the plane wave expansion was REc = 80 Ry. This value was increased at T = 300 K (REc = 160 Ry) with a reduced time step of 0.072 fs to achieve the convergence of the stress tensor (see Fig. S1 in the ESI†). By using the final configuration of amorphous Na3OCl at T = 300 K, we randomly replaced an appropriate number of Na atoms with H atoms to obtain Na2.5OH0.5Cl and Na2OHCl (corresponding to ∼17% and ∼33% of Na replaced, respectively). These systems underwent a thermal cycle via canonical NVT simulations with the following time schedule: 20 ps at T = 1800 K, 22 ps at T = 1000 K, 19 ps at T = 750 K, and 30 ps at T = 300 K. To obtain vanishing pressure at T = 300 K, the density of the system was set to 1.93 g cm−3 (Na2.5OH0.5Cl) and 1.75 g cm−3 (Na2OHCl) (Fig. 1 inset). In what follows, the results on the atomic structure are presented as time-average values over the last 20 ps of the thermal cycles at T = 300 K. For all the simulations performed here, we used the developer version of the CPMD package.54
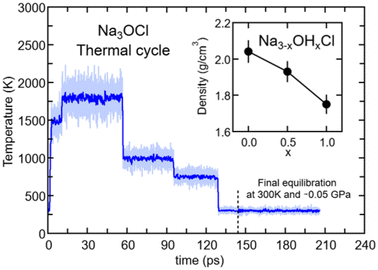 |
| Fig. 1 (Color online) Temperatures along the thermal cycle for amorphous Na3OCl: light blue line, instantaneous T values; blue line, T value sliding average with a window of 0.1 ps. The dashed vertical grey line sets the time at which the optimization of the volume at T = 300 K and 0 GPa was implemented. Inset: final density values optimized at T = 300 K for the three Na3−xOHxCl models. Error bars are estimated with tests at different volumes performed on several final configurations (300 K) to obtain a final stress tensor close to 0 GPa (see Fig. S1 in the ESI†). | |
With respect to the quantification of the disorder degree of the different models, herein we report the main feature of the smooth overlap of the atomic positions (SOAP) descriptor employed. In the SOAP formalism, the density of the neighborhood surrounding the i-th atom is smoothed using descriptor functions that encode regions of atomic geometries with respect to atomic neighbor environments within a finite cutoff radius using a local expansion of a Gaussian smeared atomic density ρi,a(r) with orthonormal functions based on spherical harmonics Yml(
) and radial basis functions Rn(r).55,56 In this study, we computed the average summation p(r) vector for the Cl atoms,57 being the only element present in equal amounts in all three models. The formula for computing the SOAP p(r) vector is given by:
| 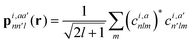 | (1) |
where
ci,anlm is defined by:
|  | (2) |
and
where the sum is over neighbours
j of element
a that is within the cutoff and
fcut(
r) is a cutoff function that smoothly goes to zero at
rcut. The hyperparameter
σa has units of length and determines the regularity (smoothness) of the representation. The absolute value of the SOAP
p(
r) vector depends on the number of species, number of descriptors calculated, and cutoff radius, making it a valuable tool for analyzing specific systems. For this study,
a = Cl and
a′ is one of those elements Cl, Na, O, or H with a cutoff radius of 5.5 Å that was chosen to encompass the next-nearest neighbor environments.
σa = 1,
nmax = 10 and
lmax = 8. The final number of descriptors computed correspond to 4185 for Na
3OCl and 7380 for the two hydroxylated models.
2.2 Dynamical simulations at finite temperatures
FPMD simulations allow the assessment of the transport properties of mobile ions in amorphous materials.58,59 In this work, the ion dynamics and transport properties were studied at different temperatures, specifically T = 300 K, 450 K, 600 K, 800 K, 1000 K, and 1200 K (Na3OCl only). The three systems were equilibrated in a NVT canonical ensemble for a total simulation time of ∼50 ps. Trajectories were collected every 20 steps (1.45 fs). The mean square displacements (MSDs) of all ions were computed according to |  | (3) |
where N is the number of the atoms of interest and
is the MSD averaged on all realizations of eqn (3) for a given value of time t. It should be noted that in its standard definition, the mean square displacement is normalized to the number of particles, say N, so as to appear as an intensive property resulting from an average over the whole system. However, for the sake of convenience, we prefer to take advantage of a definition of the MSD taking the form of eqn (3). To assess the so-called tracer diffusive D* behavior of the ions, the MSD is analysed in log–log plots via the slopes β(t) as a function of time, | 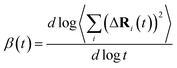 | (4) |
At very short t, a value of β(t) equal to two is expected, corresponding to a free, ideally ballistic motion of the ions, while at very long times, β(t) should reach a value equal to one, corresponding to a real diffusive regime, with the MSD growing linearly with time. We would like to stress here that this analysis is crucial to determine the effective achievement of diffusive behavior and identify the portion of the MSD plot that should be used to extract diffusion coefficients. This is particularly important in the case of disordered materials such as glasses,60 ionic liquids,61 and molten salts.62 In the present work, the lower and upper bounds of the real diffusive regime were defined within the interval delimited by (lower boundary) the t at which β(t) reaches a value of one up to (upper boundary) the value of t corresponding to a length of trajectory 15% shorter than the total one.63 For the systems that reach the diffusive regime at a given temperature, the ion diffusion coefficients (e.g. tracer diffusivity) were calculated by using the Einstein relation given by:64,65
| 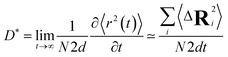 | (5) |
where
d is the dimensionality factor (
d = 3 for three dimensional systems) and we have eliminated the explicit dependence on time in
eqn (5) as a result of the infinite
t limit. When the diffusive regime is reached, the Arrhenius equation can be used to calculate the activation energy
Ea barrier for diffusion (conductivity) by fitting the data of log
D* (log
σ*)
vs. 1/
T as:
| 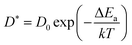 | (6) |
From the tracer diffusivity D*, the idealized ionic conductivity can be calculated based on the Nernst–Einstein relation:
| 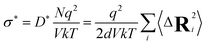 | (7) |
where
V is the total volume of the model system,
q is the charge of mobile-ion species,
T is the temperature, and
k is the Boltzmann constant. A more accurate estimation of the relevant conductivity is instead given by the Einstein formulation of the net charge migration as:
| 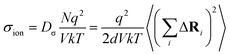 | (8) |
The charge-diffusion coefficient Dσ, which refers to the displacement of the center of mass of all the diffusing ions and is used here to consider possible correlation effects, is defined as:
| 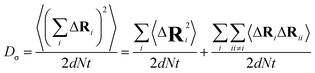 | (9) |
where
d is the dimension of the lattice where diffusion takes place and
N refers to the number of diffusing ions.
66–69 The numerator on the left-hand side was split into a self-diffusion part and a distinct-ion part, with the latter accounting for correlations between the movements of distinct ions of the same type (
i.e. Na ions).
The Haven ratio is a measure of the degree by which the trajectories of different atoms are correlated to each other.66,70 For single ion conductors, it is
|  | (10) |
becoming equal to one in the absence of any correlation between different diffusing atoms. This is asymptotically approached in the dilute limit, where diffusing atoms rarely encounter each other during their motion through a crystal. If distinct ions move preferentially in the same direction (positive correlation), then
HR > 0 and
HR < 1, which is usually observed for single-ion conductors.
69,71–73 At non-dilute concentrations, as in the majority of solid electrolytes, positive correlations between different diffusing ions become particularly relevant, resulting in
HR < 1, whereas negative correlations between different diffusing ions (distinct ions move preferentially in the opposite directions) give
HR > 1. To consider all types of ion correlations (positive and negative) involved in the system, namely cation–cation correlations (
σdist++), anion–anion correlations (
σdist−−) and cation–anion correlations (
σdist+−) contributing to the Haven ratio, the following equation is used:
| 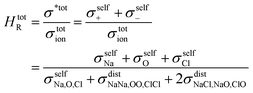 | (11) |
where
| 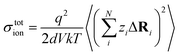 | (12) |
| 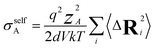 | (13) |
| 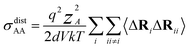 | (14) |
| 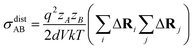 | (15) |
N is the total number of ions in the electrolyte, and zi is the charge number of ion i.61 The sum in eqn (11) can be re-expressed as shown in eqn (12)–(15). HσR is computed by considering correlation effects only promoted by Na ions, whereas with HtotR the contribution of possible correlation effects due to O and Cl ions is also taken into account. These equations are an alternative to the methods based on quantifying collective correlated effects by integration of the ion velocity autocorrelation functions.74–76
3 Results and discussion
3.1 Structural properties
3.1.1 Total X-ray and neutron structure factors.
The total X-ray and neutron structure factors (SXT(k) and SNT(k), respectively) can be directly calculated on the equilibrium trajectory in the reciprocal space.77,78 Alternatively, SXT(k) can be obtained by Fourier transform (FFT) of the real space total pair correlation function gtot(r). We underline that the calculation of ST(k) directly in the k-space is, generally, to be preferred over the Fourier transform of the real-space pair distribution functions, since the former approach avoids the effects of the finite range of integration. However, the FFT procedure is currently adopted in the literature to smooth out the noise affecting the structure factors computed directly in reciprocal space, especially in the case of glassy configurations at room temperature. In the present work, we employed the FFT method and the calculated SXT(k) and SNT(k) are shown in Fig. 2.
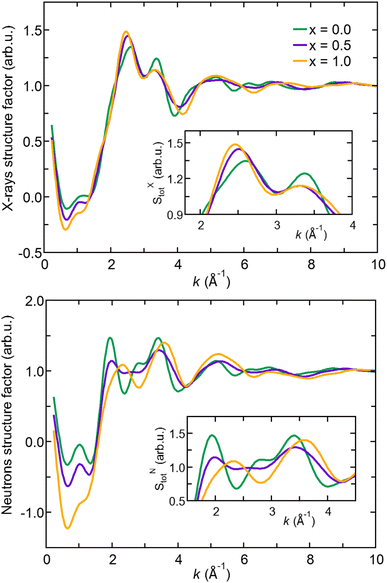 |
| Fig. 2 (Color online) Total X-ray (top) and neutron (bottom) structure factors for amorphous Na3−xOHxCl (x = 0, x = 0.5, and x = 1) obtained through Fourier transform of the total pair correlation functions at T = 300 K. Insets: zoomed-in view of the 1.8–4.0 Å−1 and 1.5–4.5 Å−1 ranges, respectively. | |
In the following, we analyze X-ray and neutron FFT ST(k) in terms of peak positions and intensities comparing the three Na3−xOHxCl systems. We remark that SXT(k) of Na3OCl features a first peak at about k ∼2.5 Å−1 with a shoulder at k ∼2.0 Å−1 and a second peak at k ∼3.5 Å−1. These first two peaks are followed by much broader maxima and minima and rapidly damped oscillations up to k ∼8.0 Å−1. Na2.5OH0.5Cl and Na2OHCl SXT(k) have very similar profiles and minor differences with respect to pristine Na3OCl. In contrast, while the X-ray structure factor SXT(k) exhibits similarities across all three cases, the neutron structure factor SNT(k) displays significant distinctions, indicating that it may be a better diagnostic tool for discerning models containing varying amounts of hydrogen. Higher intensities are found in SNT(k) for Na3OCl. By increasing x, maxima and minima are reduced and different profiles appear in between k ∼2 Å−1 and k ∼4 Å−1 to signify a lower degree of structural organization. The relatively small peak at about k ∼1 Å−1 reveals that the intermediate range order is very limited in these amorphous systems. Overall, Fig. 2 shows modifications taking place within the networks affecting an ordered structural sequence made of distinct shells of neighbors.
3.1.2 Total and partial pair-correlation functions.
The total and partial pair correlation functions for the Na3−xOHxCl (x = 0, x = 0.5, and x = 1) systems are shown in Fig. 3 and 4, respectively. In terms of total pair correlation functions, the H-free Na3OCl one shows a first narrow peak centered at ∼2.28 Å (with a full width at half maximum (FWHM) of 0.28 Å) and a broader second peak at a lower intensity at ∼2.77 Å (FWHM of 0.47 Å). These two peaks are present also in the hydroxylated systems, although with a lower ratio between the intensities for Na2.5OH0.5Cl, whereas similar values are observed in Na2OHCl. As expected, the presence of H leads to a very sharp peak at about ∼0.98 Å, with a FWHM of 0.02 Å. In addition, there is a very small maximum in Na2OHCl at ∼1.5 Å. Analyzing the partial pair correlation functions gαβ(r) (Fig. 4) allows us to trace back the role of each individual pair contribution with respect to the total one. In Table 1 we report the nearest-neighbor distances rij identified by the position of the first maximum of gαβ(r) for the three Na3−xOHxCl systems. For comparison, we also report the nearest-neighbor distances found in synthesized pure crystalline Na3OCl.15,79 The first peak of gtot(r) for amorphous Na3OCl can be ascribed entirely to the Na–O pair (∼2.28 Å), whereas the second peak is mainly attributed to the Na–Cl pair (∼2.75 Å) and only partially to Na–O and Na–Na (∼3.14 Å) gαβ(r) contributions. The first peak of gNaNa(r) is responsible for the shoulder occurring after the second peak (∼2.8–3.1 Å) found in the total pair correlation function. Analyzing the peak positions of gNaO(r) for amorphous Na3OCl, the Na–O bond distance is found slightly larger than the typical ionic bond reported for crystalline Na3OCl (∼2.25 Å), whereas the Na–Cl ionic bond distance is shorter than that of the crystalline one (∼3.18 Å). The partial pair correlation functions gNaO(r), gNaCl(r) and gNaNa(r) of the two hydroxylated systems are quite similar to those of Na3OCl. However, the intensities of the first peaks in gNaO(r) and gNaNa(r) are reduced in accordance with the lower content of Na atoms (in favor of H atoms), whereas the corresponding intensity in gNaCl(r) is increased. Replacing some of the Na atoms with H atoms causes an elongation of the Na–O distance in Na2OHCl (∼2.32 Å for ∼33% of Na atoms replaced), while no effect is found on the Na–O distance for Na2.5OH0.5Cl (∼2.27 Å for ∼17% of Na atoms replaced). In contrast, Na–Cl bond distances found in Na2.5OH0.5Cl and Na2OHCl are the same as those in Na3OCl (∼2.75 Å). gNaNa(r) shows a slight increase of the Na–Na nearest-neighbour interatomic distances with increasing H content (up to ∼3.34 Å when ∼33% of Na is substituted). The two hydroxylated systems are affected by a greater degree of disorder in comparison with Na3OCl as confirmed by a less pronounced minimum separating the peaks in gNaNa(r).
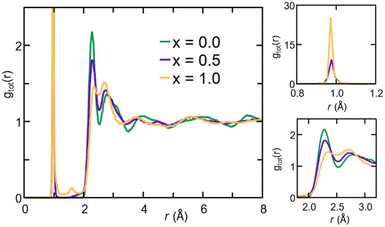 |
| Fig. 3 (Color online) Left: Total pair correlation functions for amorphous Na3−xOHxCl (x = 0, x = 0.5, and x = 1) obtained at T = 300 K. Right: Zoomed-in view of the 0.8–1.2 (top) and 1.8–3.2 (bottom) ranges. | |
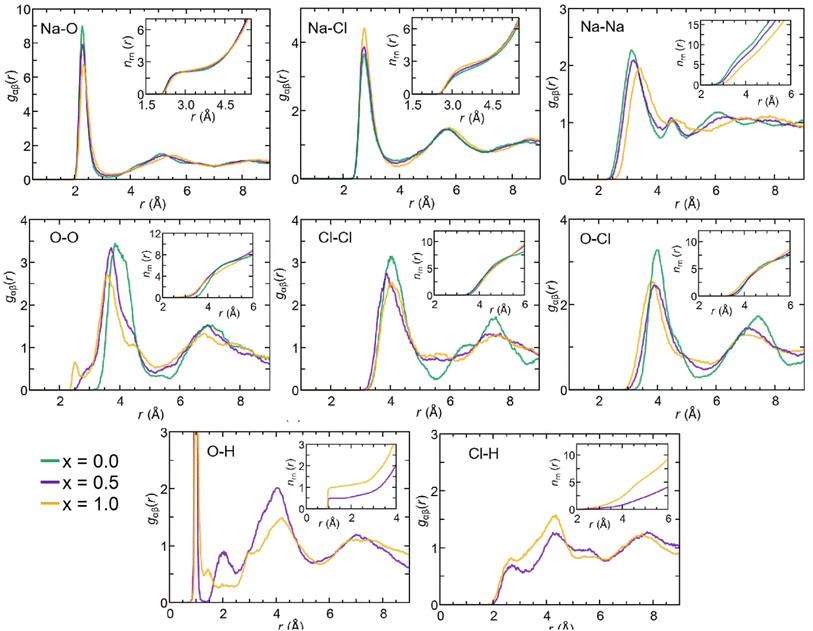 |
| Fig. 4 (Color online) Partial pair correlation functions gαβ(r) for amorphous Na3−xOHxCl (x = 0, x = 0.5, and x = 1) at T = 300 K relative to the pairs Na–O, Na–Cl, Na–Na, O–O, Cl–Cl, O–Cl, O–H, and Cl–H. The running integrals over the interatomic distance are shown. | |
Table 1 Upper part: nearest-neighbour interatomic distances rij (in Å) identified by the position of the first maximum of the pair correlation functions gij(r). We also give the first peak full width at half maximum (FWHM). For comparison, we report the values of the crystalline Na3OCl phase obtained by experiments.53,79 Lower part: total and pair coordination numbers as defined by taking different cutoff radii for the definition of a total or a “cation–anion” shell of interactions (see Section 3.1.3). Values in parentheses correspond to the statistical uncertainty on the last reported digits
|
Exp.53,79 |
FPMD, this work |
Crystal |
Na3OCl |
Na2.5OH0.5Cl |
Na2OHCl |
r
NaO
|
2.25 |
2.28(2) |
2.27(2) |
2.32(2) |
FWHM |
|
0.28 |
0.31 |
0.33 |
r
NaCl
|
3.18 |
2.75(2) |
2.75(3) |
2.74(4) |
FWHM |
|
0.47 |
0.46 |
0.52 |
r
NaNa
|
3.18 |
3.14(3) |
3.22(4) |
3.34(4) |
FWHM |
|
0.92 |
1.01 |
1.02 |
r
OO
|
4.50 |
3.85(1) |
3.72(4) |
3.61(4) |
FWHM |
|
0.86 |
0.77 |
0.72 |
r
OCl
|
3.89 |
4.00(4) |
3.91(2) |
3.78(4) |
FWHM |
|
0.79 |
1.00 |
0.93 |
r
ClCl
|
4.50 |
4.06(3) |
3.87(3) |
4.05(3) |
FWHM |
|
0.82 |
1.04 |
1.01 |
r
OH
|
— |
— |
0.98(2) |
0.97(2) |
FWHM |
— |
— |
0.02 |
0.02 |
n
t
Na
|
14 |
8.01(3) |
7.26(2) |
6.04(2) |
n
p
Na
|
6 |
4.0(1) |
4.30(1) |
4.58(2) |
n
t
O
|
6 |
6.30 (2) |
6.44(2) |
6.09(4) |
n
p
Na
|
6 |
6.29 (3) |
5.94(2) |
5.40(2) |
n
t
Cl
|
12 |
5.73(3) |
5.45(2) |
4.93(3) |
n
p
Cl
|
12 |
5.72(1) |
5.37(1) |
4.78(3) |
n
t
H
|
— |
— |
1.00(2) |
1.02(3) |
The gOH(r) partial pair correlation functions of Na2.5OH0.5Cl and Na2OHCl show that all H atoms are bonded to O atoms, forming OH hydroxyl groups with a typical O–H bond distance equal to ∼0.98 Å. This observation is further supported by the analysis of gClH(r), for which the Cl–H interatomic distance lies at values higher than ∼2.0 Å for both the hydroxylated phases, much larger than the typical Cl–H bond distance, i.e. ∼1.3 Å. For what concerns gOH(r), a few differences can be pointed out between the two hydroxylated phases. In Na2.5OH0.5Cl, a second peak exists at about ∼2.0 Å, which reflects the presence of a H-bonding network promoted between neighboring hydroxyl groups or free oxygen atoms in the form of OH⋯OH and OH⋯O interactions, respectively. In Na2OHCl, such a peak is minimally visible but a small peak at a shorter distance (∼1.5 Å) is discernible. This latter peak is the one that generally identifies H atoms (or protons) hopping between neighboring hydroxyl groups and giving rise to stable or metastable water molecules.80–85 In terms of anion–anion pair correlation functions, all three gOO(r), gClCl(r) and gOCl(r) are characterized by a first main peak centered at around ∼3.7–4.0 Å. There are interesting differences to be pointed out when comparing gOO(r) for the three Na3−xOHxCl models. In particular, Na3OCl exhibits a relatively broad first peak at ∼3.85 Å (FWHM of ∼0.86 Å), whereas the two hydroxylated models feature intense peaks at ∼3.72 Å and ∼3.61 Å (FWHM of ∼0.77 Å and ∼0.72 Å, respectively), followed by a shoulder centered at about ∼4.5 Å. Also, a small peak at ∼2.5 Å for Na2.5OH0.5Cl and a shoulder at about ∼2.9 Å for Na3−xOHxCl are worth noticing, while in the case of Na3OCl gOO(r) goes to zero for distances <3.2 Å. In terms of gClCl(r) and gOCl(r), the three models show very similar profiles, although the intensity of the main peak of the two hydroxylated systems is smaller for a higher degree of disorder with respect to Na3OCl.
3.1.3 Total and partial coordination numbers.
More insights into the Na3−xOHxCl networks can be obtained by looking at the total and partial coordination numbers (ni and nij, respectively), obtained by relying on the pair correlation functions. More precisely, nij is obtained by integrating the first peak of gij(r) up to a given cutoff distance corresponding to the position of the first minimum. The total Na, O, Cl, and H coordination numbers are then obtained as follows: nNa = nNaO + nNaCl + nNaNa + nNaH, nO = nONa + nOH + nOCl + nOO, nCl = nClNa + nClH + nOCl + nClCl and nH = nHO + nHCl + nHNa + nHH. When the first peak of the corresponding gij(r) is followed by a clear minimum well separated from a second maximum, nij does not suffer from any ambiguities for its definition, since the running coordination number exhibits a clear plateau (see the behavior in the case of Na–O interactions, inset of Fig. 4). In the opposite case, the determination of nij is less clearcut (see the Na–Na case in the inset of Fig. 4) and nij is much more sensitive to the choice of the cutoff. For these reasons, in addition to the individual pair cutoffs taken as the minima after the first peak of gij(r), we adopted a second cutoff (a “total” one) defined by the minimum after the second peak of gtot(r). The value found (3.3 Å) is determined as the average between the three amorphous models and it allows us to consider within the first coordination shell of Na atoms all the counter-ions (O and Cl) and a number of neighboring Na atoms. With this procedure, we can compare the coordination numbers of amorphous Na3−xOHxCl models to those of the crystalline phase of Na3OCl. We remind that crystalline Na3OCl (cubic phase, stable at T = 300 K15,53,79) is characterized by Na atoms coordinated by six counter-ions (two oxygen and four chlorine atoms) within the nearest-neighbor shell, and O atoms are coordinated by six Na atoms and Cl atoms are coordinated by twelve Na atoms. Within the cutoff employed above (3.3 Å) each Na atom has also eight neighboring Na atoms, leading to a “total” Na coordination of 14.53,79 Therefore, the local environment of Na in crystalline Na3OC can be thought of as made up of a total of 14 neighboring atoms, with neighboring Na interacting through O atoms via Na–O–Na linkages (see Fig. 5a). On the basis of these considerations, we performed the analysis of the coordination numbers nij of amorphous Na3−xOHxCl systems by considering the “partial” coordination number npij (definition 1) well adapted to describe the so-called “cation–anion” (i.e. counter-ions) interactions and the “total” coordination number ntij (definition 2) that is most appropriate to describe the whole set of Na neighbors. The same distinction has been introduced to obtain the distribution of the structural coordination units characterizing the environment of each atom. For a given number of neighbors l and a given atomic species, one can extract from each configuration the chemical nature of the neighbors, providing a detailed description of the network organization. It is important to underline that a coordination number gives an average behavior stemming from all neighbors with no insight into the detailed chemical nature of bonding, while the information provided by the coordination structural units shows how each atomic species organizes itself when connecting to atoms of the same or of a different kind. To help understanding these definitions, Fig. 5 contains snapshots of the local coordinations of the Na, O, and Cl atoms found in crystalline Na3OCl as well as typical structural units in amorphous Na3−xOHxCl systems issued from our calculations. Also, the insets of Fig. 4 show the running integrals of the individual partial correlation function gij(r) from which we extracted the coordination numbers ntij and npij given in Table 1. Focusing on the structural units, Fig. 6 provides their distribution in terms of the number l neighbors for Na, on the basis of definition 2. In terms of the total number of neighbours of Na atoms (within 3.3 Å), we observe that amorphous Na3OCl exhibits a markedly distinct behavior when compared to its crystalline counterpart. Specifically, the unit distribution for Na atoms is remarkably wide (from 4 to 11) and centered at l = 8.0, with the number of neighboring counter-ions decreasing from six to four, and the number of Na neighbors halved from eight to four. The partial replacement of Na with H atoms induces a shift of the l unit distribution surrounding Na atoms to lower values, centered at ∼7.3 and ∼6.0 for Na2.5OH0.5Cl and Na2OHCl, respectively. By using definition 1, the distributions of structural units for Na, O, and Cl that account for what we termed counter-ion interactions are reported in Table 2, together with the breakdown of the chemical composition of each structural unit for a given l.
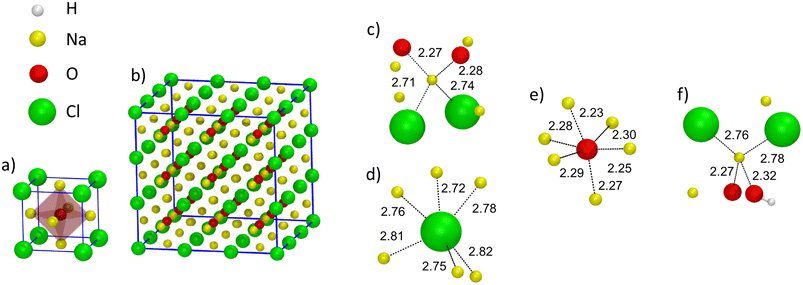 |
| Fig. 5 (Color online) (a) Representation of the unit cell of crystalline Na3OCl. The red polyhedra identify the octahedron O site. (b) Crystalline supercell of 135 atoms (unit cell replicated 3 × 3 × 3). (c–e) Coordination units for Na, O, and Cl found in amorphous Na3OCl at T = 300 K: a fourfold Na atom (O2Cl2) (c), a sixfold Cl atom (d), and a sixfold O atom (e). (f) A Na atom found in amorphous Na2.5OH0.5Cl coordinated by at least an O atom as a hydroxyl group. Counter-ion interatomic bonds are identified by a dashed line with the corresponding values. We show the atoms within a radial cutoff of 3.3 Å. Color legend: Na, yellow; O, red; Cl, green; H, white. | |
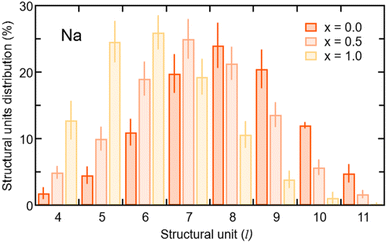 |
| Fig. 6 Distributions of the structural units for Na of amorphous Na3−xOHxCl (x = 0, x = 0.5, and x = 1) as a function of the number of neighbors in each unit (l). Values obtained by using the definition of “total” coordination ntij, given in Section 3.1.3. | |
Table 2 Distribution of the individual cation–anion nα(l) structural units, where an atom of species α (Na, Cl or O) is l-fold coordinated to a counter ion computed for glassy Na3OCl (see definition 1, section “total and partial coordination numbers”). In bold are reported the total percentages determined for each l-fold coordination. These quantities have been calculated including neighbours separated by a cutoff corresponding to the first minimum in gαβ(r). For the present work, the individual pair cutoffs used are 3.30, 3.94, 4.10, 5.06, 5.51, and 5.39 Å for, respectively, the Na–O, Na–Cl, Na–Na, O–O, Cl–Cl and O–Cl distances. A total cutoff of 3.30 Å was defined from the total g(r). Error bars are given in parentheses
|
Proportions α(l) (%) |
x = 0 |
x = 0.5 |
x = 1 |
Na
|
l = 3 |
|
14.6(1.9) |
8.4(1.0) |
3.5(1.0) |
O2Cl1 |
10.4 |
4.4 |
1.3 |
O3 |
3.6 |
2.5 |
0.6 |
l = 4 |
|
70.0(2.9) |
56.9(2.2) |
43.8(2.1) |
O2Cl2 |
36.6 |
23.8 |
13.1 |
O3Cl1 |
18.1 |
13.9 |
14.5 |
O1Cl3 |
9.9 |
11.8 |
11.0 |
O4 |
3.7 |
5.7 |
2.6 |
l = 5 |
|
13.8(1.5) |
30.4(1.6) |
43.7(2.3) |
O2Cl3 |
5.4 |
9.7 |
10.7 |
O1Cl4 |
4.4 |
6.7 |
11.6 |
O3Cl2 |
0.4 |
7.1 |
13.2 |
Cl5 |
1.5 |
2.5 |
1.5 |
l = 6 |
|
3.3(0.5) |
5.5(0.7) |
11.1(1.2) |
O4Cl2 |
— |
0.3 |
2.3 |
O3Cl3 |
— |
1.3 |
2.3 |
O2Cl4 |
0.5 |
1.0 |
1.8 |
O1Cl5 |
0.2 |
1.1 |
1.7 |
![[thin space (1/6-em)]](https://www.rsc.org/images/entities/char_2009.gif) |
O
|
l = 4 |
|
— |
<0.5 |
8.6(1.8) |
Na2H2 |
— |
— |
2.0 |
Na3H1 |
— |
0.4 |
6.6 |
l = 5 |
|
2.1(2.0) |
20.8(3.9) |
44.4(6.6) |
Na3H2 |
— |
— |
5.7 |
Na4H1 |
— |
16.5 |
38.6 |
Na5 |
1.9 |
4.3 |
0.1 |
l = 6 |
|
69.1(6.6) |
63.6(4.9) |
44.7(6.9) |
Na5H1 |
— |
24.9 |
36.0 |
Na6 |
69.1 |
38.6 |
7.3 |
l = 7 |
|
27.6(6.1) |
15.0(3.8) |
2.1(1.9) |
Na6H1 |
— |
6.1 |
2.0 |
Na7 |
27.6 |
8.9 |
— |
![[thin space (1/6-em)]](https://www.rsc.org/images/entities/char_2009.gif) |
Cl
|
l = 3 |
Na3H0 |
— |
1.2(1.0) |
4.8(3.8) |
l = 4 |
Na4H0 |
8.2(4.6) |
14.2(8.7) |
31.5(7.3) |
l = 5 |
Na5H0 |
31.6(8.0) |
40.0(8.6) |
45.4(7.4) |
l = 6 |
Na6H0 |
39.8(9.0) |
36.4(7.7) |
16.9(6.1) |
l = 7 |
Na7H0 |
17.1(6.4) |
7.7(4.4) |
— |
l = 8 |
Na8H0 |
2.4(1.9) |
— |
— |
3.1.4 Chemical identification of the structural units for the three compositions.
In the case of Na3OCl, the coordination units given in Table 2 are indicative of a first coordination shell for Na essentially fourfold (∼70.0%) in terms of cations–anions (i.e. counterions) according to definition 1 (see Section 3.1.3). The decomposition in terms of chemical species gives in decreasing order of importance the following units: O2Cl2 (∼36.6%), O3Cl1 (∼18.1%), O1Cl3 (∼9.9%) and O4 (∼3.7%). Threefold and fivefold units correspond to ∼14.6% and ∼13.8%, respectively, with a larger presence of two or one oxygen atom (O2Cl1 ∼10.4%, O2Cl3 ∼5.4% and O1Cl4 ∼4.4%). Our results demonstrate that the structure of amorphous Na3OCl is significantly different from the molecular dynamics model conjectured for glassy Li3OCl, based on nanophase segregation of regions rich in Li2O and LiCl.25 Indeed, by looking at Na atoms fourfold or even fivefold coordinated, in amorphous Na3OCl only ∼7.3% of them are exclusively coordinated to O atoms (O3 and O4) and ∼1.5% are exclusively coordinated to Cl atoms (Cl5). The value of ntO in amorphous Na3OCl is about ∼6.3 (Table 1), close to the one in crystalline Na3OCl.15,53,79 We find ∼69.1% of O atoms coordinated by six Na atoms and ∼27.6% by seven Na atoms (Table 2). For Cl, ntCl is equal to ∼5.7, almost half of that found in the crystalline phase (12). Several structural units connected to Cl are noticeable in Table 2. In fact, ∼39.8% of Cl atoms are coordinated by six, ∼31.6% by five, ∼17.1% by seven and ∼8.2% by four Na atoms. Having established the main features of the structural units in Na3OCl, we can turn to the analogous description for the case of the hydroxylated amorphous materials. In Na3−xOHxCl we observe a decrease of ntNa (from ∼8.0 to ∼6.0) from the value in Na3OCl. However, npNa does the opposite (from ∼4.0 to ∼4.6). The decrease in ntNa is due to the partial substitution of Na by H, with all the H atoms found coordinated to O atoms, forming in majority hydroxyl OH groups (ntH ∼1.0). This is confirmed by the visual inspection of the snapshots relative to Na2.5OH0.5Cl (Fig. 4e), where H is bonded to O atoms in the form of a hydroxyl group (O–H bonding distance ∼0.98 Å), with no H–Na bond formation.
In terms of the cation–anion partial coordination, we observe a slight increase of npNa, the values being ∼4.0 for Na3OCl, ∼4.3 for Na2.5OH0.5Cl and ∼4.6 Na2OHCl. Correspondingly, the distribution of the structural units given in Table 2 reveals that the percentage of Na atoms fourfold coordinated decreases (from ∼70.0% to ∼56.9% and ∼43.8%, respectively) with a concomitant increase of the fivefold ones (from ∼13.8% up to ∼30.4% and ∼43.7%). The larger number of fivefold Na-centered units at the expense of fourfold ones can be traced back to a concomitant decrease of the number of O2Cl2 units (from ∼36.6% to ∼23.8% and ∼13.1%) and a net increase of the O2Cl3, O1Cl4 and O3Cl2 units (the latter from ∼0.4% to ∼7.1% and ∼13.2%).
The behavior of the total and partial coordination numbers of O atoms is worth noticing. npO decreases from ∼6.3 in Na3OCl to ∼5.9 and ∼5.4 for Na2.5OH0.5Cl and Na2OHCl, respectively. This decrease is due to a clear increase of lower coordinated units of O at the expense of the higher-coordinated units. Na2OHCl shows a minimal content of l = 7 units and a comparable content of l = 5 and l = 6 units (∼44% versus ∼45%). The trend in the local environment of O in the hydroxylated phases results from the fact that in Na2.5OH0.5Cl ∼52% of O atoms are coordinated only by Na atoms with the remaining having, in addition to Na, one H atom as a neighbor within hydroxyl OH groups. These percentages change in Na2OHCl to accommodate more H atoms. Regarding Cl atoms the behavior is similar to the one of O atoms after partial replacement of Na by H atoms. In particular, npCl decreases from ∼5.7 in Na3OHCl to ∼5.4 and ∼4.8, for Na2.5OH0.5Cl and Na2OHCl, respectively. The amorphous nature of the network favors relatively short Na–Cl distances (∼2.75 Å) when compared to those found in the crystalline phase, where each Cl atom is surrounded by twelve Na atoms at a distance of ∼3.18 Å.
3.1.5 Structural disorder degree.
A deeper insight into the assessment of the structural disorder degree of the three Na3−xOHxCl compounds is provided by employing the smooth overlap of the atomic positions (SOAP) descriptor.55,86 SOAP is a well-established mathematical scheme widely used for analyzing the disorder degree in various materials such as amorphous graphene87 and chalcogenide-based phase-change materials.88,89Fig. 7a shows a schematic representation of the SOAP descriptor and Fig. 7b presents the distributions of the absolute value of the SOAP pCl(r) vector computed for Na3OCl from 300 K to 1200 K, along with the comparison to the crystalline phase, and from 300 K to 1000 K for Na2.5OH0.5Cl and Na2OHCl. Additionally, we provide the average SOAP
Cl(r) value and its standard deviation. For Na3OCl, the crystalline phase at 300 K exhibits a narrow SOAP pCl(r) distribution with
Cl = 0.55, with a standard deviation of 0.04. Conversely, amorphous Na3OCl at 300 K shows a more spread bimodal distribution with two main peaks (approximately 0.45 and 0.52), an average SOAP
Cl(r) value of 0.5, and a standard deviation of 0.07. As the temperature increases from 300 K to 450 K and up to 800 K, the pCl(r) value distributions transition from a bimodal to a symmetrical distribution, while the average SOAP
Cl(r) remains relatively constant up to 800 K (around 0.5). However, at higher temperatures, a noticeable shift towards lower values is observed (approximately 0.45 and 0.40 at 1000 K and 1200 K, respectively), indicating a clear increase in the disordering degree of the system, potentially leading to a phase change (e.g., from solid to liquid). Furthermore, the amorphous Na2.5OH0.5Cl model also exhibits a bimodal SOAP pCl(r) distribution at 300 K, although less structured compared to amorphous Na3OCl, whereas Na2OHCl shows a more spread SOAP pCl(r) distribution. Both Na2.5OH0.5Cl and Na2OHCl models demonstrate a symmetrical pCl(r) distributions from 450 K to 600 K, with a clear shift towards lower averaged
Cl(r) values at temperatures T ≥ 800 K.
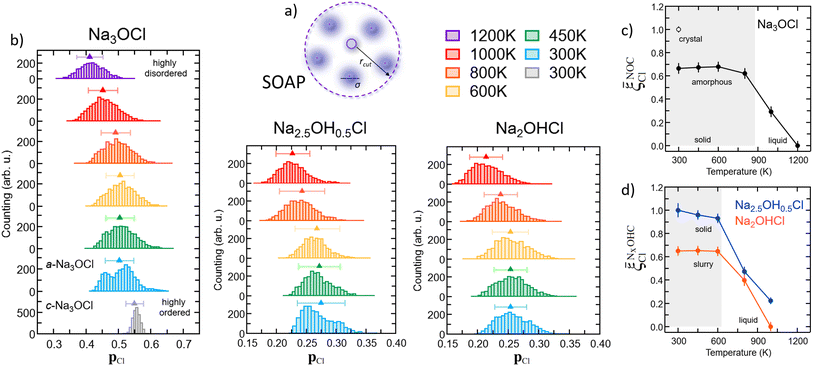 |
| Fig. 7 (Color online) (a) Schematic representation of the SOAP descriptor. (b) Na3OCl, Na2.5OH0.5Cl, and Na2OHCl SOAP pCl distributions computed at different temperatures. The data computed for crystalline Na3OCl are also reported. Structural disorder degree of the three models framed as SOAP similarity to crystalline Na3OCl ( NOCCl, (c)) and to the amorphous Na2.5OH0.5Cl phase, ( NClxOHC, (d)), respectively. | |
Capitalizing on these insights, for Na3OCl we have developed similarity metrics (on a
Cl scale from 0 to 1) to quantify the degree of disorder with reference to the highly ordered crystalline phase and the highly disordered liquid phase. In Fig. 7c and d, we report the average
Cl(r) as a function of temperature, normalized with respect to the average value of the crystalline phase for Na3OCl (Fig. 7c), and normalized with respect to the amorphous phase at 300 K for the two hydroxylated phases (Fig. 7d) such as:
| 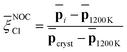 | (16) |
and
| 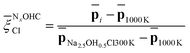 | (17) |
respectively. For the hydroxylated phases the upper bound 1 of the
Cl metric is defined by the average
Cl(
r) value of Na
2.5OH
0.5Cl at 300 K, not having at disposal any information related to their crystalline phases and consistently on the basis of the same number of elements and total number of descriptors computed (see Section 2.1). These metrics have enabled us to identify ranges of structural disorder corresponding to its crystalline (
NOCCl = 1), amorphous solid (
NOCCl ∼ 0.68,
NCl2.5OH0.5C ∼ 0.95 and
NCl2OHC ∼ 0.65) and liquid phases (
NOCCl ≤ 0.3,
NCl2.5OH0.5C ≤ 0.5 and
NCl2OHC ≤ 0.4). It is pertinent to highlight that, at 300 K, the partial substitution of Na ions with H ions leads to heightened disordering, concurrent with a lower temperature threshold for the transition from a solid to a liquid phase (at
T > 800 K for Na
3OCl and at
T > 600 K for Na
3−xOH
xCl). Furthermore, at 300 K, the disordering degree exhibited by Na
2OHCl is described as a ‘slurry’ solid, indicating a significantly lower value when compared to Na
2.5OH
0.5Cl.
3.2 Dynamical properties
3.2.1 Mean square displacements.
A first insight into the dynamical properties of amorphous Na3−xOHxCl is provided by the mean square displacement (defined in Section 2.2 and labeled MSD) of the different ions shown in Fig. 8 at T = 300 K. Additional details can be found in Fig. S2 of the ESI.† At T = 300 K, Na ions in Na3OCl are mobile, while the MSD of both Cl and O remains very low. This trend holds at higher temperatures (Fig. S2 of the ESI†), with Na ions reaching over the span of ∼50 ps MSD values of ∼2.5 Å2 at T = 300 K, ∼4 Å2 at and 450 K, ∼15 Å2 at T = 600 K, ∼48 Å2 at T = 800 K and ∼130 Å2 at T = 1000 K. These results indicate that amorphous Na3OCl behaves differently from its corresponding defect-free crystalline phase, where Na/Li diffusion is absent due to the lack of disorder induced by the presence of vacancies, as found in crystalline antiperovskites via Schottky defect pairs.90,91 Regarding Cl and O ions, the mobility for both ions at T = 300 K is lower than ∼1 Å2, and increases significantly only at very higher temperature (T ≥ 1000 K) indicating a motion beyond a mere local rearrangement. Heenen and coworkers25 obtained analogous results for both Cl and O ions; yet, the Cl higher mobility was interpreted as not-negligible and later also termed ‘sluggish’.92
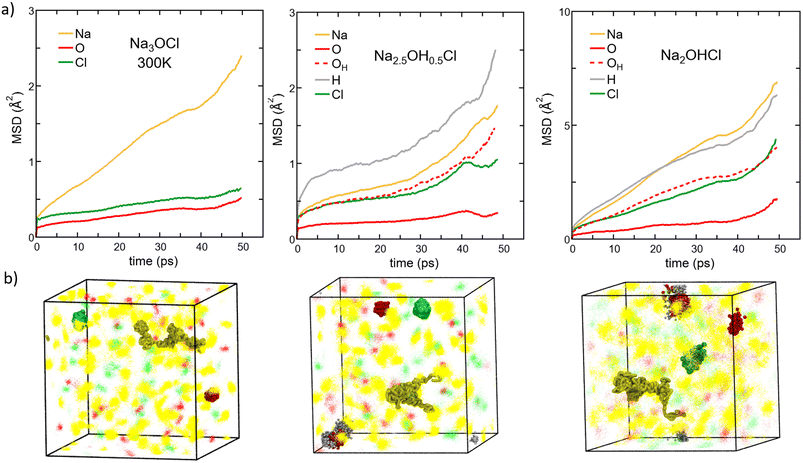 |
| Fig. 8 (Color online) (a) MSD versus time for each element in amorphous Na3−xOHxCl (x = 0, x = 0.5, and x = 1) at T = 300 K. Colors legend: Na, yellow; O non-bonded to H atoms, red (solid line); O bonded to H atoms, red (dashed line); H, grey; Cl, green. Note that the MSD calculated at the temperatures T = 300 K, T = 450 K, T = 600 K, T = 800 K, and T = 1000 K are reported in the ESI (Fig. S1†). (b) Density plots of ∼50 ps trajectories of all elements are shown as dots for the three models simulated at 300 K. Na, O, and Cl atom positions are given in yellow, red, and green, respectively. H atoms are omitted for clarity. Examples of an individual local Na migration path and Cl, O and OH local vibrations are highlighted in spheres. | |
Different behaviors are recorded in the case of the hydroxylated systems. For both the hydroxylated phases, we reported the MSD data of individual oxygen atoms and those bonded to H atoms (as OH− anions) separately (corresponding to about ∼50% of oxygen atoms in Na2.5OH0.5Cl and only to a small % for Na2OHCl). At T = 300 K, we found that Na and individual O ions in Na2.5OH0.5Cl have MSD values similar to those found in Na3OCl. The MSD of Cl reaches ∼0.9 Å2 over ∼40 ps followed by stabilization, and O atoms involved in OH groups show a MSD value of about ∼1 Å2, whereas H atoms show a steady time increase of the MSD. The enhanced mobility of H atoms can be ascribed to the high rotational dynamical disorder of H atoms around an O atom of a hydroxyl group (see Fig. S3 in the ESI†). Individual O atoms show the least mobility of all, with a MSD stable at ≤0.5 Å2. This trend is clearly supported by the MSD of Na2.5OH0.5Cl as a function of temperature. Focusing on Na ions at higher temperature, their MSD after ∼50 ps is ∼1.4 and ∼2.0 times larger than the one found in Na3OCl at 450 K and 600 K, respectively. Therefore, it appears that the presence of H atoms promotes an increase in Na ion mobility at temperatures higher than T = 300 K. This occurs also for Cl ions and both O and H involved in OH groups. In contrast, individual O ions (i.e. non-bonded to H) are found somewhat less mobile.
To complete our case study and extract a global picture from the behaviour of the mean square displacement, we focus on Na2OHCl. A first observation from Fig. 8 (rightmost part) reveals that similar trends for the MSD are found. However, OH− anions boost the MSD of the other species significantly already at room temperature. This effect can be fully appreciated by noting that in Na2OHCl ∼92% of O atoms bind at least one H atom either as OH− anions or in water molecules. At T = 300 K and after ∼50 ps, Na ions in Na2OHCl reached a value of MSD ∼3.2 times larger than the one found in Na3OCl, and with H equally highly mobile and with Cl and O involved in OH groups showing a certain degree of mobility although lower than for Na ions. Individual O atoms show a stable MSD value of ≤3.5 Å2, indicating mere local vibrations. At 300 K, by considering density plots spanning the 50 picosecond simulation period (Fig. 8b), we observe that atoms other than Na ions only exhibit localized vibrations around central positions. This vibration's extension increases in the order Na3OCl < Na2.5OH0.5Cl < Na2OHCl, with Na ions showcasing extended migration paths. To enhance comprehension of atom dynamics throughout the simulation timeframe, we highlighted in Fig. 8b individual local migration paths for Na, Cl, O, and OH in the density plot visualizations. These visualizations further corroborate our MSD-based observations, with Na ions demonstrating extended migration paths. Meanwhile, individual O ions (not involved in OH groups) exhibit highly localized vibrations, and Cl and OH ions show slightly extended vibrations, though consistently localized.
We conclude that all three models at 300 K exhibit solid-state dynamical characteristics. At higher temperature, in Na2OHCl Na the MSD after ∼50 ps is about ∼4.5 times larger than the one found in Na3OCl at 450 K and 600 K. These results indicate, by all means, that the mobility of Na ions is further boosted by the presence of H atoms. Overall, it is evident that Na3OCl exhibits a consistent profile from 300 K to 800 K, wherein Na ions remain highly mobile with their MSD progressively increasing. In contrast, Cl and O ions stay localized around their initial positions. Notably, these latter two ions begin steady migration only from T = 1000 K. In the hydroxylated phases, Na2.5OH0.5Cl and Na2OHCl, the profiles remain akin to those observed at 300 K up to 600 K. Both Na and H ions experience escalating MSD values, whereas individual O ions (not involved in OH groups) maintain a constant position with minimal vibrations. Remarkably, as Na2OHCl features only a minor concentration of individual O atoms, we can assert that only a small portion of the model exhibits solid-like behavior up to 600 K. Beyond this temperature, no individual O atoms ‘live’, as all O atoms are either bonded or interact with H atoms, and the systems behave as a liquid phase. These results are fully consistent with the previous structural analysis based on the SOAP descriptor.
The Na MSD value obtained for Na2OHCl at 450 K (∼21 Å2) is remarkably larger than the one reported by Dawson et al.12 for crystalline Li2OHCl (∼6 Å2 after ∼50 ps at T = 800 K). What we found for Na2OHCl differs drastically from what has been reported for crystalline Li2OHCl antiperovskites, where only rotational dynamics were highlighted for the hydroxyl OH− groups and no long-range mobility.12 In this crystalline phase, H atoms are characterized by a constant MSD of ∼2 Å2 at T = 600 K on a time scale of ∼50 ps, whereas the present Na2OHCl model features an increase of the MSD to a value of ∼60 Å2 after ∼50 ps at T = 600 K (Fig. S2 in the ESI†).
3.2.2 The paddlewheel effect.
We have found that the mobility of Na ions in Na3−xOHxCl is strictly related to the presence of hydroxyl OH− anions. An important relationship between the Na-ion transport mechanism and O–H rotation effects in these systems has been reported by Song et al.93 and Howard et al.94 These studies demonstrate that the rapid rotation of O–H bonds leads to the creation of empty space promoting the formation of defects, which, in turn, are crucial for achieving fast, correlated Na-ion transport. The rotational disorder due to the hydroxyl OH− anions, along with other anions, is a feature common to other solid electrolytes, such as crystalline Na3OBH413 and Na3−xO1−x(NH2)x(BH4).95 Ion migration occurs via a mechanism that combines the concerted motion of ions with large quasi-permanent reorientations of the surrounding hydroxyl groups. This latter effect, known as the paddlewheel mechanism,30,96,97 is typically observed in high-temperature crystalline polymorphs.98
Unlike in crystalline materials, the paddlewheel dynamics in glassy matrices can contribute to the ion mobility also at room temperature,30via the strong coupling between the rotational motion of the anions and the translational motion of the alkaline cations.30,96,98 Based on the above analyses, we propose that H-containing amorphous Na3−xOHxCl compounds show three distinct features that facilitate the occurrence of paddlewheel dynamics. The degree to which these dynamics manifest themselves is temperature-dependent. First, these systems contain hydroxyl groups which are free to rotate, impacting the neighbouring cations. Second, the amorphous matrix has a lower density than its crystalline counterpart that makes available additional free volume for O–H rotations, facilitating anion reorientations. Third, while it is typical for most glasses to exhibit a lower density compared to their crystalline form, amorphous Na3−xOHxCl systems have a potentially unusual characteristic – the absence of covalent bonding in their atomic structure. These features are found in strong accordance with the features of paddlewheel dynamics reported for 75Li2S-25P2S5 glass.30
A more detailed view of the dynamical mechanism is shown in Fig. 9a–d. These panels illustrate the rotation of two hydroxyl (OH) groups nearest neighbor to one sodium (Na) ion during Na migration. Throughout the entire process, O(OH) and Na maintain their coordination of six cations and four-to-five anions, respectively. The coordination of Na is preserved by means of rotational displacements of the anion through a paddlewheel-type mechanism. Another salient feature observed in these migration events is the dissociation or undocking of Na from a subset of its neighboring anions at the onset of the process, followed by its association or docking to new anions towards the end.30
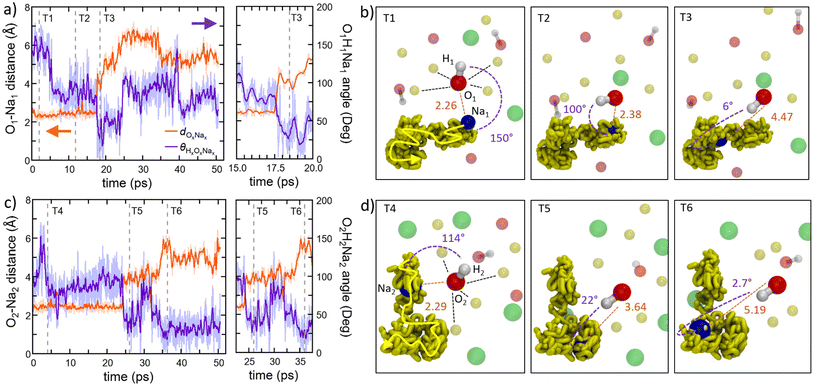 |
| Fig. 9 (Color online) Proposed paddlewheel dynamics monitored in amorphous Na2.5OH0.5Cl at 300 K. We show the temporal evolutions of two distinct scenarios involving different atoms. (a and c) The interatomic distance between an O atom (O1 and O2) of one hydroxyl group and a coordinated Na atom (Na1 and Na2) and the HONa angles (H1O1Na1 and H2O2Na2) along the ∼50 ps trajectory. Light orange and purple lines correspond to the instantaneous interatomic distance dOxNax and θHxOxNax angle, whereas the strong lines correspond to the sliding average with a window of 0.1 ps. (b and d) Atomistic views of the temporal frames Ty (y = 1–6) indicated in (a) and (b). In (b), between 0 and 5 ps, a sixfold coordinated O1 atom of one hydroxyl group shows a bonding distance of 2.26 Å with the Na atom labelled Na1 and an HONa angle of ∼150° (T1). At 5 ps, the hydroxyl OH bond is rotating with respect to Na1 (from ∼150° to ∼100°) while maintaining a distance O1–Na1 equal to 2.38 Å. This scenario lasts up to 17 ps (T2). After 17.5 ps and up to 25 ps a further rotation of the OH group bringing the corresponding angle from ∼100° to ≤50° (∼6° at T3) induces the displacement of the Na1 atom to a larger distance (>4.5 Å) escaping the first coordination shell of the O1 atom. In (d) between 4 and 23 ps, a sixfold coordinated O2 atom of one hydroxyl group shows a bonding distance of 2.29 Å with the Na atom labelled Na2 and an HONa angle of 90°–120° (T4). At 26 ps, the hydroxyl OH bond is rotating with respect to Na2 (to ≤50°) inducing the displacement of the Na2 atom to a larger distance (>3.6 Å) escaping the first coordination shell of the O2 atom (T5). A further rotation to 3° is accompanied by a displacement of the Na2 atom to a larger distance (>5 Å, T6). The surrounding atoms of O1 and O2 at a distance lower than >5 Å are shown in transparent color. Dashed lines indicate the atoms coordinated within the first coordination shell of O1 and O2 (<3.3 Å). The concerted migration pathways of Na1 and Na2 atoms along the ∼50 ps trajectory are shown in transparent dark yellow. As a guide, an arrow is added to indicate the direction of the pathway. Color legend: Na atoms, yellow; O atoms, red; Cl atoms, green; H atoms, white; Na1 and Na2 atoms at Ty points are shown in blue. | |
Former experimental and computational results have also stirred a debate about the formation of water molecules in antiperovskites. Based on the analysis presented above, Na2.5OH0.5Cl appears not to show any water formation since all H atoms are bound in OH groups, while our Na2OHCl model system does, with ∼9.3% of O atoms involved in H2O molecules. The formation of these water molecules can be elucidated by , as shown in Fig. 10a, a typical H hopping process involving OH groups in Na2OHCl at T = 300 K. We first show the temporal evolution of two representative distances. The first is the one between the H atom termed H1 belonging to a water molecule, previously formed, and the O atom termed O1 atom to which it is bonded (dO1H1, initially at ∼0.98 Å). The second one concerns the same H1 atom and a second O atom termed O2 atom belonging to a neighbouring OH group (dO2H1, initially at ∼3.8 Å; Fig. 10b). After about ∼15 ps, H1 starts to form a strong hydrogen bond (H-bond) with the neighbouring O2 atom of one OH hydroxyl group at a distance of ∼1.9 Å. The mean lifetime of this transient H-bond is ∼2.5 ps. Then, H1 is shared between O1 and O2via forming and breaking O–H bonds showing an average dO1,2H1 distance of ∼1.6 Å and forming eventually a water molecule upon H transfer (Fig. 10c and d). Its transition rate, defined as the number of inter-unit transitions per ps observed within a given process (H-bond or water molecule formation), is of about 1.0 before the formation of a stable new H2O molecule. In Fig. 10 panels (e–f) exemplify the dynamical evolution of the previous environment toward H transfer to a third hydroxyl group (from O2 to O3), resulting in the formation of a new water molecule. The presented mechanism is very similar to the known Grotthuss one,80–85 in which the H atom switches between a σ bond and a hydrogen bond to propagate and eventually stabilize in a newly formed water molecule, as in proton-conducting solid-oxide materials.
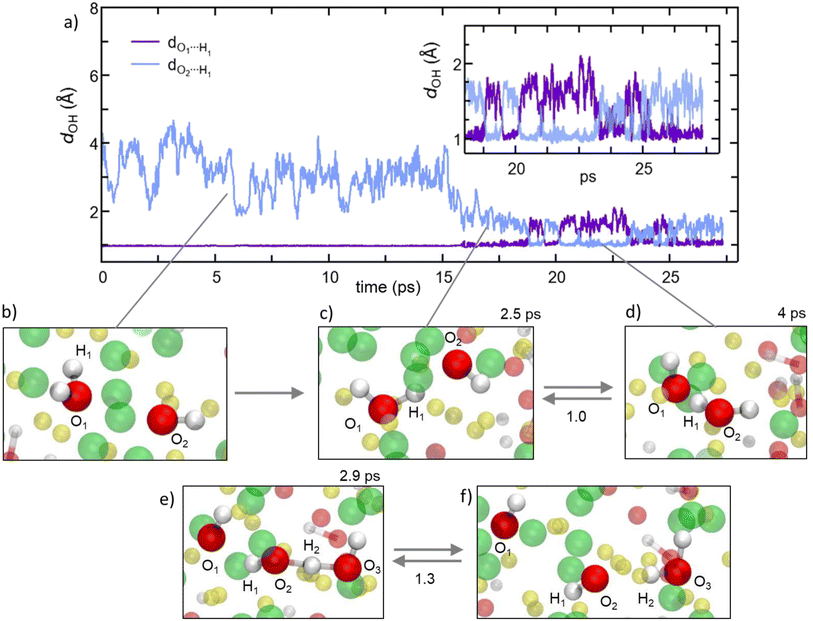 |
| Fig. 10 (Color online) For the Na2OHCl system: the proton hopping process by which H atoms migrate through a dynamical switch between σ and hydrogen bonds giving rise to the formation of metastable water molecules (i.e. Grotthuss-like mechanism).80–85 (a) Temporal evolution of the interatomic distance between one H atom labelled H1 atom, belonging to a water molecule initially formed, and its bonded O atom labelled O1. Also shown is the temporal evolution of the distance between H1 and a second O atom labelled O2 that is part of a neighboring hydroxyl group. Inset: zoomed-in view of the temporal window along which H1 is shared between two O1 and O2, leading to the transient formation and breaking of the OH bond and water molecules. (b–f) Inter-unit transitions between structural units in amorphous Na2OHCl at 300 K. Indicatively, the mean lifetimes of the transient H-bonds and water molecules are reported on top of the panels, whereas the transition rates are reported between the panels. Here, the transition rate is defined as the number of inter-unit transitions per ps observed within a given process (H-bond or water molecule formation). Color legend: Na, yellow; O, red; Cl, green. | |
3.2.3 Ions diffusion.
The long-range mobility of alkaline ions in a solid-state electrolyte can be assessed by considering the log–log plot of the MSD (eqn (3)vs. t for the temperatures of interest (see Fig. 11). Several regimes are noticeable as a function of time, especially at T = 300 K in our case. The initial part consists of a ballistic regime with the MSD showing a quadratic dependence on time. With increasing t, a plateau is attained corresponding to the so-called caging regime with Na ions trapped in a very limited portion of space by the nearest neighbors. In Na3OCl, the cage regime clearly persists up to ∼1 ps at T = 300 K and ∼0.8 ps at T = 450 K, while it does not manifest itself at higher temperatures. Then, the diffusive regime for Na atoms sets in at longer times with Na ions moving along preferential paths. Under these conditions, the MSD takes a linear dependence on time and the ion self-diffusion coefficients (D*) can be obtained via the Einstein equation (eqn (5)) at different temperatures.
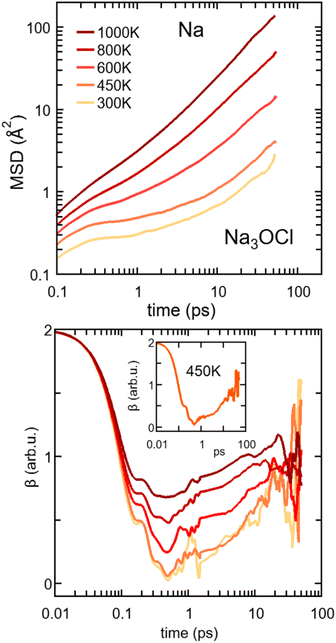 |
| Fig. 11 (Color online) Top: log–log plot of MSD vs. time for Na in Na3OCl at temperatures in between T = 300 K and 1200 K. Bottom: corresponding values of β. Inset: β value as a function of time at T = 800 K. Note that the log–log plots of MSD vs. time data for the Na3−xOHxCl (x = 0, x = 0.5, and x = 1) systems are reported in the ESI (Fig. S2†). | |
The onset of the diffusive regime can be monitored via the time behaviour of β (eqn (4)), given in Fig. 11 for Na3OCl. As expected, the higher the temperature the faster the β approaches ∼1, i.e. at T = 450 K, 600 K, 800 K, and 100 K and this target value is reached after ∼33 ps, ∼23 ps, ∼15 ps, and ∼8 ps respectively. At 300 K, the diffusive regime is not reached within ∼50 ps, the behaviour of β being affected by statistical noise for increasing time. Analogous information on the MSD of the two hydroxylated systems is given in Fig. S2, ESI† (linear plot) and Fig. S4, ESI† (log–log plot, as in Fig. 11). Remarkably, for T ≤ 450 K, the cage regime disappears for Na2.5OH0.5Cl and Na2OHCl. This is consistent with the boosted Na ion dynamics due to the OH anion rotational mechanism discussed before. Also, the MSD trend of H atoms features high values at short times (<∼1 ps) for any given temperature, again due to the rotational disordered of OH anions. At longer times, the MSD profiles of H and Na atoms do superpose as a result of a fully enhanced diffusive behavior concerning all Na and OH groups.
Having identified the temperatures at which Na ions reached the diffusive regime (range 450 K–800 K for Na3OCl and 300 K–600 K for Na2.5OH0.5Cl and Na2OHCl), we calculated for the three systems the self-diffusion coefficients (tracer, D*) for Na by using the Einstein equation (eqn (5)) and then converted them into Na-ion conductivities with eqn (7). By plotting these data according to the relationship logD* vs. 1000/T (Fig. 12) and fitting it in the Arrhenius form, we obtain by extrapolation the uncorrelated tracer conductivity σ* of Na ions at T = 300 K, as customarily done for crystalline solid-state electrolytes.7,12D* and σ* values are reported in Table 3, together with the calculated activation energy (Ea) of Na ion transport. We have also computed the tracer diffusion coefficient (D*) for the least mobile element, namely individual O atoms, at 300 K for all three models. The calculated values are 1.7 × 10−7 cm2 s−1, 1.5 × 10−7 cm2 s−1, and 3.9 × 10−7 cm2 s−1 for the three respective models. Notably, these values are lower by two orders of magnitude than the typical diffusion coefficients observed in liquids (∼10−5 cm2 s−1),42 thus providing additional reinforcement to the previous observations that underline the solid-like nature of the three models at 300 K.
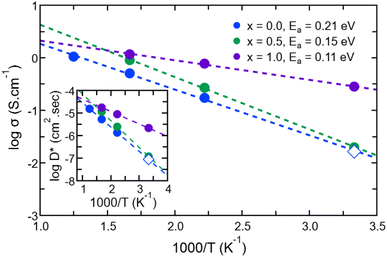 |
| Fig. 12 (Color online) Na-ion self-diffusion coefficients (inset) and conductivities of amorphous Na3−xOHxCl (x = 0, x = 0.5, and x = 1). We also report the activation energies of Na ionic conduction obtained via a linear Arrhenius fit. Closed circles correspond to FPMD data and open circles correspond to data at T = 300 K extrapolated by using the linear Arrhenius fit. | |
Table 3 Na tracer diffusion coefficients (D*), Na ion tracer (σ*), charge (σσ) and total conductivities (σtot), activation energies (Ea) and Haven ratio (HJ,totR) extrapolated at T = 300 K and directly computed at T = 450 K on the basis of FPMD simulations. We report the values for the three Na3−xOHxCl (x = 0, x = 0.5, and x = 1) models
|
T (K) |
x = 0 |
x = 0.5 |
x = 1.0 |
Data extrapolated at T = 300 K.
|
D* (cm2 s−1) |
300 Ka |
8.65 × 10−8 |
1.20 × 10−7 |
2.13 × 10−6 |
σ* (S cm−1) |
300 Ka |
0.016 |
0.08 |
0.28 |
E
a (eV) |
300 K |
0.21 |
0.15 |
0.11 |
D* (cm2 s−1) |
450 K |
1.36 × 10−6 |
2.47 × 10−6 |
8.74 × 10−6 |
σ* (S cm−1) |
450 K |
0.17 |
0.27 |
0.77 |
σ
ion (S cm−1) |
450 K |
0.08 |
0.10 |
0.22 |
σ
totion (S cm−1) |
450 K |
0.67 |
0.75 |
0.99 |
H
σR
|
450 K |
2.03 |
2.67 |
3.50 |
H
totR
|
450 K |
0.89 |
1.84 |
2.51 |
3.2.4 Ionic conductivity and correlation effects.
In terms of conductivity σ*, we obtained at 300 K a value of ∼16 mS cm−1 for Na3OCl and a Ea value of Na ion transport of 0.21 eV. The σ* value obtained is two order of magnitude larger of the ∼0.2 mS cm−1 obtained by Dawson et al. at 500 K (ref. 7), showing a similar Ea calculated for defected crystalline Na3OCl.1,17 For this model, we also computed the energy barrier Ea of Na ions during migration through distinct anion pairs, namely OO, ClCl, and OCl, within the Na3OCl model through static transition calculations, as commonly performed for crystalline systems.15 The outcomes of our computations reveal energy barrier values of 0.23 eV, 0.41 eV, and 0.73 eV, respectively, for the aforementioned anion pairs (Fig. S5 within the ESI†). This analysis underscores a significantly higher favorability for migration via the OO pair, in contrast to the ClCl and OCl pairs. It is noteworthy that the minimum energy barrier obtained, which corresponds closely to the value of 0.21 eV obtained from Arrhenius fitting of self-diffusion and conductivity data, supports the consistency and validity of our findings. While we acknowledge that the static calculations do not take into account the influence of thermal motion and collective dynamics, their alignment with the Arrhenius fitting outcomes adds weight to the support they provide. It is worth mentioning that, due to the intricate nature of OH rotational dynamics as the main promoter of Na migration in the hydroxylated models, similar static calculations have not been performed for these two compounds as these aspects are better assessed by following the Arrhenius fitting of self-diffusion and conductivity processes extracted from FPMD trajectories.
For Na2.5OH0.5Cl and Na2OHCl, we obtained at 300 K Ea = 0.15 eV, Ea = 0.11 eV and a Na tracer conductivity equal to ∼80 mS cm−1 and ∼280 mS cm−1, respectively. These data demonstrate that Na ion transport is promoted by the addition of H atoms. These values correspond to the ideal case where no collective effects are taken into account, as those due to alike-Na ions or different ions. However, since these effects are present in experiments, it is appropriate to account for them by considering the so-called Haven ratio (HR) which can be obtained viaeqn (9) and (10) in order to quantify the contribution of alike-Na ions (HσR)66–68,71 or a total contribution coming from all positive and negative ions (HtotR, eqn (11)).61
Often, previous studies on crystalline Li/Na antiperovskites assumed a Haven ratio equal to 1 disregarding collective effects.7,12 While single-ion conducting glasses typically exhibit Haven ratios of ∼0.1–0.6 indicative of positive correlating effects,60,99 ionic liquids61 and molten salts62 behave oppositely with HR values larger than 1. For comparison, in Table 3 we report the values of HσR, HtotR and the corresponding Na and total conductivities obtained at T = 450 K. We obtained HσR values larger than 1, indicating a strong negative effect of the collective correlations of alike-Na ions. Therefore, at this temperature, the behaviour of amorphous Na3−xOHxCl systems in terms of conductivity is closer to ionic liquids and molten salts than to a glassy matrix. We also remark that this specific effect is more important when Na is partially replaced by H (from 2.0 for Na3OCl to 2.7 and 3.5 for the two hydroxylated phases). Finally, the resulting Na charge conductivities (σion) are lower than the uncorrelated Na tracer conductivity σ* for the three systems.
In terms of HtotR, we obtained for Na3OCl a much lower value than HσR (0.89 vs. 2.03), which indicates significant positive collective correlated effects when the mobility of all ions is taken into consideration. The value obtained for Na3OCl is found close to the typical values found for single-ion conducting glasses.60,99 This is not the case for the two hydroxylated models, which showed values always larger than 1 for HtotR meaning that in the presence of H (OH hydroxyl groups) negative effects are more important. The corresponding final total ionic conductivities (σtotion) are moderately larger to the Na ions uncorrelated tracer ionic conductivity (σ*) estimation for the three models Na3OCl (0.67 vs. 0.17 S cm−1), Na2.5OH0.5Cl (0.75 vs. 0.27 S cm−1), and Na2OHCl (0.99 vs. 0.77 S cm−1). The σtotion obtained here at 450 K is finally found 1.5 times higher in Na-deficient Na2OHCl than Na2OHCl, whereas in the case of Na2OHI/Na2OHI an increase of 102 times at about 550 K was reported.100
Therefore, the Na ionic conductivity of disordered Na3−xOHxCl systems can be a viable, effective alternative to the crystalline counterparts, with the partial replacement of Na with H enhancing ion transport. However, the mobility of the other ions (Cl, O, and H) is concomitantly boosted which might be detrimental to practical electrolyte applications.
4 Conclusions
X3OA-based antiperovskites have shown promising potential as solid-state electrolytes for ion batteries due to their structural and dynamical properties. However, while the structure and ion dynamics mechanisms of crystalline X3OA compounds are well-known, there is a lack of quantitative structural characterization for amorphous antiperovskites. In this study, we used first-principles molecular dynamics to quantitatively assess the structure and ion dynamics mechanisms of three amorphous Na3−xOHxCl (with x = 0, 0.5, and 1) Na-rich antiperovskites. We found that amorphous Na3OCl shows a substantially different structure compared to its crystalline counterpart, with a disordered structure exhibiting limited intermediate range order and short-range order driven by a majority of four-fold Na atoms (∼70%), in terms of counter-ion coordination by means of ionic bonds. Na–O bond distances are close to those reported in the crystalline phase, while Na–Cl distances are significantly shorter. As a result, a reduced number of neighboring counter-ions and Na atoms are favored in the local surrounding of Na atoms in the amorphous phase. Na is found primarily coordinated to both anions (O and Cl), with only a small percentage of Na atoms coordinated exclusively to O or Cl atoms. These results unveil contrasting differences in the structure of amorphous Na3OCl compared to previous models conjectured for glassy Li3OCl, which was based on the segregation of Li2O and LiCl-rich regions.
The partial replacement of Na atoms with H in the two hydroxylated phases studied (Na2.5OH0.5Cl and Na2OHCl) gives rise to a lower degree of structural organization when compared to H-free Na3OCl, with all H atoms bonded to O atoms. At 300 K, the partial substitution of Na ions with H precipitates a heightened disordering degree concurrent with a lower temperature threshold for the solid-to-liquid transition. Particularly, Na2OHCl exhibits a disordering degree we characterize as ‘slurry’, owing to a degree to be significantly greater with respect to Na2.5OH0.5Cl. Amorphous Na3OCl has a unique microscopic structure that enables remarkable Na ion dynamics and ionic conductivity, challenging that of defective crystalline phases. The presence of hydroxyl OH− anions in the hydroxylated models is crucial for the mobility of Na ions, which is enhanced by rapid rotation of O–H bonds and paddlewheel-type mechanisms. This promotes the formation of available space, which is essential for achieving fast Na-ion transport. Interestingly, the paddlewheel dynamics in the hydroxylated models of glassy matrices can contribute to ion mobility even at room temperature, owing to the strong coupling between the rotational motion of the anions and the translational motion of the alkaline cations. In terms of correlated dynamical effects, the behaviour of amorphous Na3OCl is found closer to that of single-ion conducting glasses, whereas the two hydroxylated models are found closer to ionic liquids and molten salts than to a glassy matrix. We also observe a noticeable shift in favor of positive correlated effects for Na3OCl, whereas for the two hydroxylated phases negative effects are more important.
Concerning the presence of water molecules in antiperovskites, our study proves that the presence of H atoms promotes the formation of H-bonds in Na2.5OH0.5Cl, while only in the case of Na2OHCl the formation of a small amount of water is observed.
Overall, the results obtained in this work add to the growing evidence that disordered Na-rich antiperovskites provide a viable and effective alternative to their crystalline counterparts as solid state electrolytes. Our results underscore the significance of conducting quantitative structural assessments to better comprehend the impact of the structure on the performances of these systems.
Data availability
Representative trajectory files of the three Na3−xOHxCl models are available at the European Center of Excellence Novel Materials Discovery (CoE-NOMAD) repository.101
Author contributions
Tan-Lien Pham: investigation, writing, and formal analysis. Mohammed Guerboub: investigation, writing, and formal analysis. Assil Bouzid: methodology and writing. Carlo Massobrio: methodology and writing. Mauro Boero: methodology and writing. Young-Han Shin: conceptualization, supervision, investigation, and writing. Guido Ori: conceptualization, supervision, investigation, and writing.
Conflicts of interest
There are no conflicts to declare.
Acknowledgements
This work was supported by the National Research Foundation of Korea (NRF) grants funded by the Ministry of Science and ICT (MSIT), government of South Korea (grant no. 2021R1F1A1048555). We acknowledge financial support from the Agence National de la Recherche (ANR) within the framework of the project AMSES No. ANR-20-CE08-0021, the Seed Money program (project MEDIA) of Eucor-The European Campus, the Interdisciplinary Thematic Institute QMat, as part of the ITI 2021–2028 program of the University of Strasbourg, CNRS and Inserm. This project has also received funding from the European Union’s Horizon 2020 research and innovation programme under the Marie Skłodowska-Curie grant agreement number 847471 (QUSTEC). Calculations were performed by using resources from GENCI (Grand Equipement National de Calcul Intensif, grant no. A0100807670, A0120807670, and A0140807670) and the Pole HPC Équipe@Meso of the Université de Strasbourg.
References
- J. Dawson, T. Famprikis and K. Johnston, J. Mater. Chem., 2021, 9, 18746–18772 RSC.
- W. Xia, Z. Yang, Z. Feipeng, A. Keegan, Z. Ruo, L. Shuai, Z. Ruqiang, Z. Yusheng and S. Xueliang, Chem. Rev., 2022, 122, 3763–3819 CrossRef CAS PubMed.
- T. Shao, C. Liu, W. Deng, C. Li, X. Wang, M. Xue and R. Li, Batteries Supercaps, 2019, 2, 403–427 CrossRef.
- K. Kim, Y. Li, P. Tsai, F. Wang, S. Son, Y. Chiang and D. Siegel, Chem. Mater., 2022, 34, 947–958 CrossRef CAS.
- K. Yang, D. Liu, Z. Qian, D. Jiang and R. Wang, ACS Nano, 2021, 15, 17232–17246 CrossRef CAS PubMed.
- Y. Zhao and L. L. Daemen, J. Am. Chem. Soc., 2012, 134, 15042–15047 CrossRef CAS PubMed.
- B. Goldmann, M. Clarke, J. Dawson and M. Islam, J. Mater. Chem. A, 2022, 10, 2249–2255 RSC.
- H. Fang and P. Jena, Proc. Natl. Acad. Sci. U.S.A., 2017, 114, 11046–11051 CrossRef CAS PubMed.
- Z. Deng, D. Ni, D. Chen, Y. Bian, S. Li, Z. Wang and Y. Zhao, InfoMat, 2022, 4, 2567–3165 CrossRef.
- W. Yonggang, Q. Wang, Z. Liu, Z. Zhou, S. Li, J. Zhu, R. Zou, Y. Wang, J. Lin and Y. Zhao, J. Power Sources, 2015, 293, 735–740 CrossRef.
- J. Oh, L. He, B. Chua, K. Zeng and L. Lu, Energy Stor. Mater., 2021, 34, 28–44 Search PubMed.
- J. Dawson, H. Chen and M. Islam, J. Phys. Chem. C, 2020, 122, 23978–23984 CrossRef.
- E. Ahiavi, J. Dawson, U. Kudu, M. Courty, M. Islam, O. Clemens, C. Masquelier and T. Famprikis, J. Power Sources, 2020, 471, 228489 CrossRef CAS.
- X. Xu, K. S. Hui, K. N. Hui, H. Wang and J. Liu, Mater. Horiz., 2020, 7, 1246–1278 RSC.
- T.-L. Pham, W. I. Choi, A. Shafique, H. J. Kim, M. Shim, K. Min, W.-J. Son, I. Jang, D. S. Kim, M. Boero, C. Massobrio, G. Ori, H. S. Lee and Y.-H. Shin, Phys. Rev. Appl., 2023, 19, 034004 CrossRef CAS.
- S. Gao, C. Tassel, S. Fujii, H. Ubukata, T. Zhu, D. Zhang, T. Broux, T. Saito, C. Zhong, E. Yoruk and K. Yamamoto, Chem. Mater., 2022, 34, 6815–6823 CrossRef CAS.
- J. Zheng, B. Perry and Y. Wu, ACS Mater. Au, 2021, 1, 92–106 CrossRef CAS PubMed.
- M. H. Braga, N. S. Grundish, A. J. Murchison and J. B. Goodenough, Energy Environ. Sci., 2017, 10, 331–336 RSC.
- M. H. Braga, J. A. Ferreira, V. Stockhausen, J. E. Oliveira and A. El-Azab, J. Mater. Chem. A, 2014, 2, 5470–5480 RSC.
- M. H. Braga, A. J. Murchison, J. A. Ferreira, P. Singh and J. B. Goodenough, Energy Environ. Sci., 2016, 9, 948–954 RSC.
- M. H. Braga, J. A. Ferreira, A. J. Murchison and J. B. Goodenough, J. Electrochem. Soc., 2017, 164, A207–A213 CrossRef CAS.
- I. Hanghofer, G. J. Redhammer, S. Rohde, I. Hanzu, A. Senyshyn, H. M. R. Wilkening and D. Rettenwander, Chem. Mater., 2018, 30, 8134–8144 CrossRef CAS.
- D. A. Steingart and V. Viswanathan, Energy Environ. Sci., 2018, 11, 221–222 RSC.
- J. T. Frith, M. J. Lacey and U. Ulissi, Nat. Commun., 2023, 14(1), 420 CrossRef CAS PubMed.
- H. H. Heenen, J. Voss, C. Scheurer, K. Reuter and A. C. Luntz, J. Phys. Chem. Lett., 2019, 10, 2264–2269 CrossRef CAS PubMed.
- Y. W. Choi, C. M. Araujo and R. Lizarraga, J. Power Sources, 2022, 521, 230916 CrossRef CAS.
- Y. Tian, F. Ding, H. Zhong, C. Liu, Y.-B. He, J. Liu, X. Liu and Q. Xu, Energy Stor. Mater., 2018, 14, 49–57 Search PubMed.
- Y. Tian, F. Ding, L. Sang, Y. He, X. Liu and Q. Xu, Int. J. Electrochem. Sci., 2019, 14, 4781–4798 CrossRef CAS.
- Y. Gao, S. Sun, X. Zhang, Y. Liu, J. Hu, Z. Huang, M. Gao and H. Pan, Adv. Funct. Mater., 2021, 31, 2009692 CrossRef CAS.
- J. G. Smith and D. J. Siegel, Nat. Commun., 2020, 11, 1483 CrossRef CAS PubMed.
- W. Xia, Y. Zhao, F. Zhao, K. Adair, R. Zhao, S. Li, R. Zou, Y. Zhao and X. Sun, Chem. Rev., 2022, 112, 3763–3819 CrossRef PubMed.
- In the present manuscript we use the formula Na3−xOHxCl to denote the hydroxylated phases in accordance with the widely accepted and commonly used in the community and literature to describe this type of compound (see for instance ref. 1, 2, 12, 16 and 33).
- L. Gao, P. Jiangyang, D. Longbang, Z. Jinlong, W. Liping, G. Song, Z. Ruqiang, K. Le, H. Songbai and Y. Zhao, Chin. J. Chem., 2023, 42, 100048 Search PubMed.
- R. Car and M. Parrinello, Phys. Rev. Lett., 1985, 55, 2471–2474 CrossRef CAS PubMed.
- J. Perdew, K. Burke and M. Ernzerhof, Phys. Rev. Lett., 1996, 77, 3865 CrossRef CAS PubMed.
- N. Troullier and J. L. Martins, Phys. Rev. B: Condens. Matter Mater. Phys., 1991, 43, 1993–2006 CrossRef CAS PubMed.
- S. Nosé, Mol. Phys., 1984, 52, 255–268 CrossRef.
- S. Nosé, J. Chem. Phys., 1984, 81, 511–519 CrossRef.
- W. G. Hoover, Phys. Rev. A, 1985, 31, 1695–1697 CrossRef PubMed.
- G. J. Martyna, M. L. Klein and M. Tuckerman, J. Chem. Phys., 1992, 97, 2635–2643 CrossRef.
- P. Blochl and M. Parrinello, Phys. Rev. B: Condens. Matter Mater. Phys., 1992, 45, 9413–9416 CrossRef PubMed.
-
C. Massobrio, The Structure of Amorphous Materials Using Molecular Dynamics, IOP Publishing, 2022 Search PubMed.
- A. Bouzid, T. Pham, Z. Chaker, M. Boero, C. Massobrio, Y. Shin and G. Ori, Phys. Rev. B, 2021, 103, 094204 CrossRef CAS.
- G. Ori, A. Bouzid, E. Martin, C. Massobrio, S. L. Roux and M. Boero, Solid State Sci., 2019, 95, 105925 CrossRef CAS.
- G. Ori, C. Massobrio, A. B. M. Boero and B. Coasne, Phys. Rev. B: Condens. Matter Mater. Phys., 2014, 90, 045423 CrossRef.
- Q. Evrard, Z. Chaker, M. Roger, C. M. Sevrain, E. Delahaye, E. Gallart, M. Gilliot, P. C. Leuvrey, J.-M. Rueff, P. Rabu, M. Boero, C. Massobrio, G. Ori and G. Rogez, Adv. Funct. Mater., 2017, 27, 1703576 CrossRef.
- Z. Chaker, G. Ori, M. Boero and C. Massobrio, Beilstein J. Nanotechnol., 2018, 8, 857–860 CrossRef PubMed.
- Z. Chaker, A. Bouzid, B. Coasne, C. Massobrio, M. Boero and G. Ori, J. Non-Cryst. Solids, 2018, 498, 288–293 CrossRef CAS.
- G. Cotin, C. Kiefer, F. Perton, M. Boero, B. Ozdamar, A. Bouzid, G. Ori, C. Massobrio, D. Begin, B. Pichon, D. Mertz and S. Begin-Colin, ACS Appl. Nano Mater., 2018, 1, 4306–4316 CrossRef CAS.
- B. Ozdamar, A. Bouzid, G. Ori, C. Massobrio and M. Boero, J. Chem. Theory Comput., 2018, 14, 225–235 CrossRef CAS PubMed.
- D. Gentili and G. Ori, Nanoscale, 2022, 14, 14385–14432 RSC.
- D. Gentili, G. Ori, L. Ortolani, V. Morandi and M. Cavallini, ChemNanoMat, 2017, 3, 874–878 CrossRef CAS.
- K. Hippler, S. Sitta, P. Vogt and H. Sabrowsky, Acta Crystallogr., 1990, 463, 736–738 Search PubMed.
-
CPMD, Copyright 1990–2023 by IBM Corp. and 1994–2001 by Max Planck Institute, Stuttgart, https://github.com/CPMD-code Search PubMed.
- S. De, A. P. Bartók, G. Csányi and M. Ceriotti, Phys. Chem. Chem. Phys., 2016, 18, 13754–13769 RSC.
- V. L. Deringer, A. P. Bartók, N. Bernstein, D. M. Wilkins, M. Ceriotti and G. Csányi, Chem. Rev., 2021, 121, 10073–10141 CrossRef CAS PubMed.
- L. Himanen, M. O. Jäger, E. V. Morooka, F. F. Canova, Y. S. Ranawat, D. Z. Gao, P. Rinke and A. S. Foster, Comput. Phys. Commun., 2020, 247, 106949 CrossRef CAS.
-
Molecular Dynamics Simulations of Disordered Materials, ed. C. Massobrio, J. Du, M. Bernasconi and P. Salmon, Springer International Publishing, Cham, 2015 Search PubMed.
-
Theory and Simulation in Physics for Materials Applications: Cutting-Edge Techniques in Theoretical and Computational Materials Science, ed. E. Levchenko, Y. Dappe and G. Ori, Springer International Publishing, Cham, 2020 Search PubMed.
- J. Habasaki, Int. J. Appl. Glass Sci., 2020, 11, 421–431 CrossRef CAS.
- N. M. Vargas-Barbosa and B. Roling, ChemElectroChem, 2020, 7, 367–385 CrossRef CAS.
- A. Gheribi, K. Machado, D. Zanghi, C. Bessada, M. Salanne and P. P. Chartrand, Electrochim. Acta, 2018, 274, 266–273 CrossRef CAS.
- H. Fanf and P. Jena, Nat. Commun., 2022, 13, 2078 CrossRef PubMed.
-
D. Frenkel and B. Smit, Understanding Molecular Simulation, from Algorithms to Applications, Computational Science, from Theory to Applications (Academic), 2001 Search PubMed.
-
M. Allen and D. J. Tildesley, Computer Simulation of Liquids, Oxford University Press, USA, 1989 Search PubMed.
- A. V. der Ven, G. Ceder, M. Asta and P. Tepesch, Phys. Rev. B: Condens. Matter Mater. Phys., 2001, 64, 184307 CrossRef.
- L. Haarmann and K. Albe, Solid State Ionics, 2021, 363, 115604 CrossRef CAS.
- S. Sicolo, C. Kalcher, S. Sedlmaier, J. Janek and K. Albe, Solid State Ionics, 2018, 319, 83–91 CrossRef CAS.
- Y. Gao, A. Nolan, P. Du, Y. Wun, C. Yang, Q. Chen, Y. Mo and S. Bo, Chem. Rev., 2020, 120, 5954–6008 CrossRef CAS PubMed.
- G. Murch, Solid State Ionics, 1982, 7, 177–198 CrossRef CAS.
- W. Richards, T. Tsujimura, L. Miara, Y. Wang, J. Kim, S. Ong, I. Uechi, N. Suzuki and G. Ceder, Nat. Commun., 2016, 7, 1–8 Search PubMed.
- Z. Zhu, I. Chu, Z. Deng and S. Ong, Chem. Mater., 2015, 27, 8318–8325 CrossRef CAS.
- Y. Lin, A. Yong, W. Gustafson, C. Reedy, E. Ertekin, J. Krogstad and N. Perry, Curr. Opin. Solid State Mater. Sci., 2020, 24, 100875 CrossRef CAS.
- A. Marcolongo and N. Marzari, Phys. Rev. Mater., 2017, 1, 025402 CrossRef.
- N. Molinari, Y. Xie, I. Leifer, A. Marcolongo, M. Kornblutha and B. Kozinsky, Phys. Rev. Lett., 2021, 217, 025901 CrossRef PubMed.
- M. Mottet, A. Marcolongo, T. Laino and I. Tavernelli, Phys. Rev. Mater., 2019, 3, 035403 CrossRef CAS.
- P. Salmon and A. Zeidler, J. Condens. Matter Phys., 2015, 27, 133201 CrossRef PubMed.
- A. Zeidler, P. Salmon, T. Usuki, S. Kohara, H. Fischer and M. Wilson, J. Chem. Phys., 2022, 157, 094504 CrossRef CAS PubMed.
- T. Pham, A. Samad, H. Kim and Y. Shin, J. Appl. Phys., 2018, 124, 164106 CrossRef.
- D. Marx, ChemPhysChem, 2006, 7, 1848–1870 CrossRef CAS PubMed.
- M. Tuckerman, D. Marx and M. Parrinello, Nature, 2002, 417, 925–929 CrossRef CAS PubMed.
- M. Boero, T. Ikeshoji and K. Terakura, ChemPhysChem, 2005, 6, 1775–1779 CrossRef CAS PubMed.
- K. Kamiya, M. Boero, M. Tateno, K. Shiraishi and A. Oshiyama, J. Am. Chem. Soc., 2007, 129, 9663–9673 CrossRef CAS PubMed.
- H. Ali, F. Giberti, J. Cuny, T. D. Kühne and M. Parrinello, Proc. Natl. Acad. Sci. U.S.A., 2013, 110, 13723–13728 CrossRef PubMed.
- M. Hellstrom, M. Ceriotti and J. Behler, J. Phys. Chem. B, 2018, 122, 10158–10171 CrossRef PubMed.
- A. P. Bartók, R. A. Kondor and G. Csányi, Phys. Rev. B: Condens. Matter Mater. Phys., 2013, 87, 184115 CrossRef.
- Z. El-Machachi, M. Wilson and V. L. Deringer, Chem. Sci., 2022, 13, 13720–13731 RSC.
- N. Bernstein, B. Bhattarai, G. Csányi, D. A. Drabold, S. R. Elliott and V. L. Deringer, Angew. Chem., 2019, 131, 7131–7135 CrossRef.
- L. Sun, Y.-X. Zhou, X.-D. Wang, Y.-H. Chen, V. L. Deringer, R. Mazzarello and W. Zhang, npj Comput. Mater., 2021, 7, 29 CrossRef CAS.
- Z. Lu, C. Chen, Z. M. Baiyee, X. Chen, C. Niu and F. Ciucci, Phys. Chem. Chem. Phys., 2015, 17, 32547–32555 RSC.
- X. L. J. Howard, A. Chen, J. Zhu, S. Li, G. Wu, P. Dowden, H. Xu, Y. Zhao and Q. Jia, Adv. Sci., 2016, 3, 1500359 CrossRef PubMed.
- J. Serejo, J. Pereira, R. Mouta and L. Rego, Phys. Chem. Chem. Phys., 2021, 23, 6964–6973 RSC.
- A. Song, Y. Xiao, K. Turcheniuk, P. Upadhya, A. Ramanujapuram, J. Benson, A. Magasinski, M. Olguin, L. Meda, O. Borodin and G. Yushin, Adv. Energy Mater., 2018, 8, 1700971 CrossRef.
- J. Howard, Z. Hood and N. Holzwarth, Phys. Rev. Mater., 2017, 1, 075406 CrossRef.
- P.-C. Tsai, S. Mair, J. Smith, D. M. Halat, P.-H. Chien, K. Kim, D. Zhang, Y. Li, L. Yin, J. Liu, S. H. Lapidus, J. A. Reimer, N. P. Balsara, D. J. Siegel and Y.-M. Chiang, Adv. Energy Mater., 2023, 13, 2203284 CrossRef CAS.
- H. Fang and P. Jena, Nat. Commun., 2022, 13, 2078 CrossRef CAS PubMed.
- F. Fu, X. Chen, N. Yao, X. Shen, X. Ma, S. Feng, S. Wang, R. Zhang, L. Zhang and Q. Zhang, J. Energy Chem., 2022, 70, 59–66 CrossRef.
- Z. Zhang and L. Nazar, Nat. Rev. Mater., 2022, 7, 389–405 CrossRef.
-
Molecular Dynamics of Nanostructures and Nanoionics, Simulations in Complex Systems, ed. J. Habasaki, eBook, Imprint Jenny Stanford Publishing, New York, 2020 Search PubMed.
- F. Wang, H. A. Evans, K. Kim, L. Yin, Y. Li, P.-C. Tsai, J. Liu, S. H. Lapidus, C. M. Brown, D. J. Siegel and Y.-M. Chiang, Chem. Mater., 2020, 32, 8481–8491 CrossRef CAS.
-
Data Deposited and Available at NOMAD, https://doi.org/10.17172/NOMAD/2023.03.06-1 Search PubMed.
|
This journal is © The Royal Society of Chemistry 2023 |