DOI:
10.1039/D3NR01482G
(Review Article)
Nanoscale, 2023,
15, 11346-11365
RNA-cleaving DNAzymes for accurate biosensing and gene therapy
Received
30th March 2023
, Accepted 30th May 2023
First published on 5th June 2023
Abstract
RNA-cleaving DNAzymes, which are single-stranded catalytic DNA, have attracted considerable attention in bioanalysis and biomedical applications because of their high stability, high catalytic activity, easy synthesis, easy functionalization, and modification. By incorporating these DNAzymes with amplification systems, the sensing platforms can be used to detect a series of targets with high sensitivity and selectivity. In addition, these DNAyzmes possess therapeutic potential by cutting the mRNA in cells and viruses to regulate the expression of the corresponding proteins. This review systematically summarizes the applications of RNA-cleaving DNAzymes in recent years, explaining the uniqueness and superiority of RNA-cleaving DNAzymes in biosensing and gene therapy. Finally, this review extends the discussion to the challenges and perspectives of applying RNA-cleaving DNAzyme as a diagnostic and therapeutic agent. This review provides the researchers with valuable suggestions and promotes the development of DNAzymes for accurate analysis, early diagnosis, and effective therapy in medicine and their broader applications beyond biomedicine.
1. Introduction
Accurate analysis of disease-related markers is critically essential for early diagnosis and making a treatment plan.1,2 In recent years, the appearance of some new biological detection and therapeutic technologies has improved the quality of life of patients and provided a chance for survival. However, some actual obstacles still hinder the practical applications of these technologies. Taking cancer as an example, some existing diagnostic techniques including biopsy,3 molecular detection,4 and bioimaging technology5 have some insurmountable obstacles to providing convincing results for patients. Besides, apart from surgery and chemotherapy, gene therapy is an emerging technology with high security, while low delivery efficiency and poor targeting ability still hinder its widespread application.6 Although researchers have made many attempts to overcome these problems, current progress could not meet the demands for accurate diagnosis and efficient therapy.
DNAzymes, which can integrate diagnosis and therapy, have attracted researchers’ attention to overcome these obstacles. DNAzymes are generated by in vitro screening technologies, and they have specific catalytic functions under the effect of cofactors. Since the first RNA-cleaving DNAzyme was discovered in 1994, an increasing number of DNAzymes with different structures and functions have been reported.7 Among these, the 10–23 type and 8–17 type DNAzymes are the most extensively investigated due to their advantage of cleaving targeted substrate RNA.8–12 Specifically, the recognition arms of DNAzymes combine with their substrates by Watson–Crick base pairing to cleave them by the catalytic core of DNAzymes with the help of cofactors. The working principle of the 10–23 type RNA-cleaving DNAzyme is shown in Fig. 1. With excellent RNA cleavage capability, these DNAzymes have been widely recognized in biosensing and gene therapy.
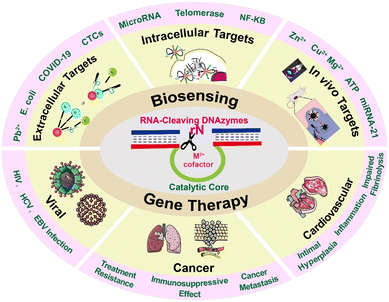 |
| Fig. 1 Schematic illustration of DNAzyme mediated RNA cleavage and its applications for biosensing and gene therapy. | |
The significant advantages of high stability, high catalytic activity, easy synthesis, easy functionalization, and modification endow DNAzymes with enormous potential in disease diagnosis and therapy, especially when they are integrated with nanosystems.13 The catalytic activity of DNAzymes can be activated only with the participation of cofactors, such as Pb2+, Mg2+, and Cu2+.9 By integration into special detection systems, DNAzymes have been widely employed for sensing metal ions, nucleic acids, proteins, and enzymes.14 However, the ligand–receptor interaction mode is not able to satisfy the demands for highly sensitive detection of low concentration targets, where signal amplification technologies are necessary to perform the analysis. Encouragingly, a series of amplification systems were developed to incorporate with DNAzymes for achieving sensitive detection. For example, the simple incorporation of DNAzymes with rolling circle amplification (RCA) technology endows the assay system with a more powerful signal amplification ability, thus greatly lowering the detection limits.15 Besides, based on the RNA cleaving characteristics, these DNAyzmes exhibit therapeutic potential by cutting the mRNA in cells and viruses to regulate the expression of the corresponding proteins. Compared to other gene therapy tools, RNA-cleaving DNAzymes show more stability and silencing effects.16 Based on these points, this review summarized the recent progress of RNA-cleaving DNAzymes in biosensing and gene therapy and systematically introduced the advantages of DNAzymes in intracellular, extracellular, and in vivo sensing, as well as in the gene therapy of cancers, cardiovascular diseases, and viral infections. The wide use of RNA-cleaving DNAzymes proves that these small nucleic acids can serve as efficient tools for the development of biological detection platforms and new gene therapy drugs for viral infections, tumors, and other diseases.
2. DNAzyme-based biosensing
As one functional nucleic acid, DNAzymes with RNA cleaving properties have remarkable advantages, such as low cost, robust stability, and high catalytic efficiency.17 A large number of DNAzyme-based biosensing platforms have been exploited to address the shortcomings of limited sensing capability and sensitivity. DNAzymes can be introduced into the sensing system to detect different targets, such as metal ions,18–20 small molecules, proteins,21,22 microRNAs,23 other biomarkers,24,25etc. These DNAzyme-based biosensors have been applied in different situations such as extracellular, intracellular, and in vivo sensing.26–28Table 1 summarizes the recent progress in RNA-cleaving DNAzyme-based biosensing systems.
Table 1 RNA-cleaving DNAzyme-based biosensing
Application |
Target |
Sample type |
LOD |
Ref. |
Extracellular |
Pb2+ |
All-sealed paper |
0.004 nM |
31
|
Drinking water |
0.96 ppb |
35
|
UO22+ |
Environmental water sample |
2.6 nM |
32
|
Cu2+ |
Serum |
3 nM |
43
|
Hg2+ |
[Fe(CN)6]3−/4− solution |
23 fM |
38
|
Tap water |
3.58 fM |
37
|
Tetracycline |
Milk |
4.411 nM |
45
|
Ca2+ |
Serum |
3.8 × 10−6 M |
44
|
E. coli
|
Milk |
250 CFU mL−1 |
47
|
Apple juice |
1.57 CFU mL−1 |
48
|
Cysteine |
Human urine |
2 nM |
53
|
Thrombin |
Serum |
4.5 pM |
52
|
COVID-19 |
Nasopharyngeal swab |
0.96 copy per μL |
51
|
CTCs |
Peripheral blood |
1 cell per mL |
54
|
Intracellular |
MicroRNA |
MCF-7 and HeLa cells |
19.4 pM |
61
|
HeLa cells |
— |
60
|
A549, H520 cells |
102–103 cells per mL |
58
|
A549 cells |
— |
59
|
Survivin mRNA |
HeLa cells |
1 pM |
62
|
ATP |
MCF-7 cells |
— |
64 and 65 |
4T1 cells |
— |
66
|
NF-κB |
HeLa cells |
1.8 pM |
69
|
Telomerase |
HeLa cells |
7 cells per mL |
67
|
UDG |
HeLa cells |
8.71 × 10−6 (U mL−1) |
72
|
UDG |
LO2, MCF-7, and HepG2 |
0.23 (mU mL−1) |
25
|
In vivo
|
Zn2+ |
Zebrafish |
— |
74
|
Zn2+ |
MCF-7 tumor-bearing mice |
— |
36, 73 and 75 |
MicroRNA |
MCF-7 tumor-bearing mice |
1.8 × 10−11 M |
80
|
— |
32 and 81 |
2.1 Extracellular biosensing
DNAzyme-based biosensors have been widely used to detect food contaminants,29 pathogens,18 metal ions,30–32 and various small molecules.33,34 Compared with conventional detection methods, RNA-cleaving DNAzyme-based biosensors have demonstrated superior stability and accuracy. Due to the diversity of molecular targets, DNAzyme-based sensing systems could be applied to different areas, such as food safety, environmental monitoring,35 and clinical diagnostics.36 For example, Yuan et al. reported a DNA nanomachine as a super signal amplifier with a simple and efficient “Crab Claw” shape for the ultra-sensitive electrochemical detection of mercury ions (Hg2+)37 (Fig. 2A). The electrochemical sensor has a low detection limit of 3.58 fM for Hg2+. It provided a simple, high-detection sensitivity, and rapid method for detecting heavy metal ions in environmental pollutants. Similarly, Chai et al. designed a bifunctional DNAzyme-based electrochemical biosensor with two detection pathways to test Hg2+ in the environment.38 Compared with the conventional single pathway, this strategy was effective at avoiding false positive signals, and providing highly reliable and sensitive data, thus successfully circumventing the shortcomings of previous proportional electrochemical biosensors (Fig. 2B). Lead ions (Pb2+), as one of the most toxic metallic pollutants, can cause serious damage to the human body when lead ion contaminated water is consumed. However, the detection methods developed in the past few decades were limited to well-trained operators.39 Thus, Guo et al. developed a fast and precise Pb2+ sensing nanoplatform based on DNAzyme–AuNP probes for highly selective and sensitive detection of Pb2+.40 Huang et al. reported an electrochemical DNAzyme based biosensing platform to detect Pb2+ in spiked real-world samples.41 In brief, these methods are simple, fast, and highly sensitive and offer potential platforms for detecting metal ions in real water samples.
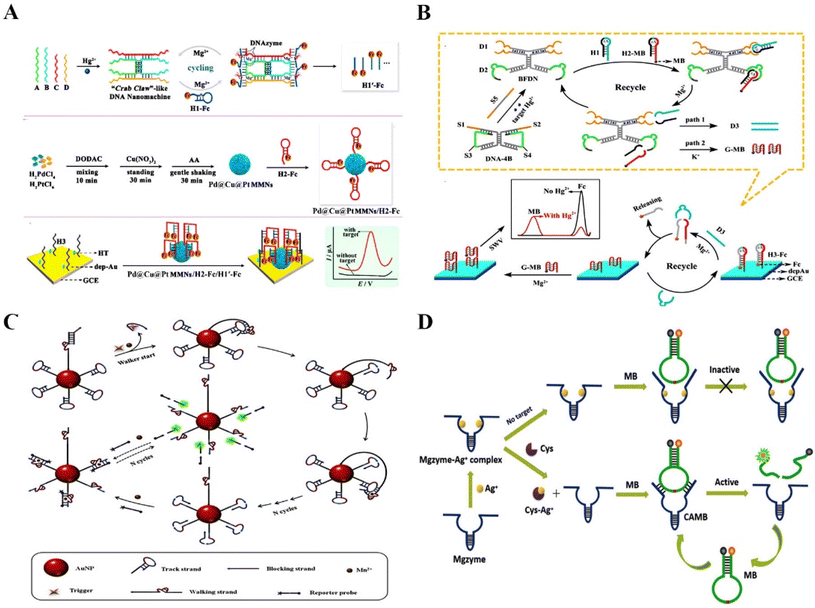 |
| Fig. 2 The application of RNA-cleaving DNAzymes in extracellular sensing. (A) A DNA nanomachine as a super signal amplifier with a “Crab Claw” shape for the ultra-sensitive electrochemical detection of Hg2+.37 This figure has been reproduced from ref. 37 with permission from American Chemical Society, copyright 2021. (B) A bifunctional DNAzyme electrochemical biosensor with two detection pathways for the detection of Hg2+ in the environment.38 This figure has been reproduced from ref. 38 with permission from American Chemical Society, copyright 2019. (C) Accurate identification of mutant pathogens based on programmable DNAzymes and targeted nucleic acid sequences.52 This figure has been reproduced from ref. 52 with permission from Elsevier B.V. All rights reserved, copyright 2021. (D) A turn-on fluorescent biosensor based on the competitively binding silver ion on DNAzymes for amplified Cys detection in human urine samples.53 This figure has been reproduced from ref. 53 with permission from Royal Society of Chemistry, copyright 2019. | |
Food-borne diseases have a significant impact on human health worldwide. Bacterial contamination and excessive metal ion-containing edibles are the main culprits, which means that developing rapid and reliable detection strategies is essential to prevent the occurrence of such diseases.42,43 Xiong et al. proposed an electrochemical biosensor using glass carbon electrodes modified with nitrogen-doped graphene, gold nanoparticles, and DNAzymes to detect the concentration of Ca2+ in the serum of dairy cows.44 The developed biosensor exhibited an ultrasensitive sensing ability with a detection limit of 3.8 × 10−6 M, which presented a high potential to diagnose subclinical hypocalcemia. Sun et al. developed a simple and low-cost fluorescence signal-on sensing strategy based on DNAzymes to detect tetracycline in milk (detection limit of 4.411 nM).45Escherichia coli (E. coli) is a food-borne pathogen with widespread sources and low infectious doses from contaminated milk, water, and other routes, which seriously threatens human health.46 Nonspecific binding is one of the severe challenges in food detection. Didar et al. reported a species-specific protein activatable DNAzyme probe to detect E. coli in milk.47 The probe significantly improved the performance of DNAzyme, showing excellent detection ability for E. coli in milk, with a detection limit as low as 250 CFU mL−1 in less than one hour. Xu et al. also constructed a fluorescence sensor based on the E. coli-specific protein activatable RNA-cleaving DNAzymes to detect E. coli in drinking water and apple juice samples (detection limit of 1.57 CFU mL−1).48 All of the above reports have proved the potential of the RNA-cleaving DNAzyme-based sensing platforms in detecting different analytes with high selectivity and sensitivity. In addition to analyzing food contaminants, rapid, high-speed, and acute detection of disease-related biomarkers in biological samples is vital for identifying and diagnosing.49 However, current Watson–Crick hybridization-based assays have false positives because of the cross-reactivity of wild-type sequences.50 Huang et al. developed a method for the accurate identification of mutant pathogens based on a programmable DNAzyme and targeted nucleic acid sequences.51 The DNAzyme has site-specific cleavage capability, enabling the highly specific identification of single nucleotide variants (SNVs). To increase the detection sensitivity, T7 RNA polymerase-mediated transcriptional amplification was designed. This rapid, simple, highly sensitive biosensor could detect SNVs and COVID-19 variants with a detection limit of 0.96 copy per μL. For another disease sensing, Jiang et al. reported a DNAzyme walker-induced cascade signal amplification strategy for sensitively detecting disease-associated protein thrombin with a detection limit of 4.5 pM52 (Fig. 2C). Furthermore, Xie et al. presented a turn-on fluorescent biosensor for amplified cysteine (Cys) detection in human urine samples based on the competitive binding of silver ions with DNAzymes53 (Fig. 2D). Song et al. proposed a ratiometric surface-enhanced Raman spectroscopy (SERS)-based method through the circulating tumor cell (CTC)-triggered DNA walker with the assistance of Zn2+-specific DNAzymes for highly sensitive detection of CTCs.54 Hence, the biosensor has a very low background fluorescence and significantly increased fluorescence intensity, with a detection limit of 2 nM. In fact, RNA-cleaving DNAzymes are suitable to serve as sensing tools for specific testing of a large number of analytes, thus DNAzyme-based sensing strategies have been applied to overcome the deficiencies of clinical detection.
2.2 Intracellular biosensing
DNAzymes are ideal for metal-ion binding because of their vital role in the formation of the catalytic core in single-stranded DNA.14 Although RNA-cleaving DNAzymes have been revealed to be promising tools for sensing extensive analytes, the lack of metal cofactors is an important challenge for intracellular analysis.55 Technically, intrinsic intracellular cofactors are not sufficient for the efficient formation of the catalytic structural domain for the cleavage of substrates. It has been shown that metal cofactor-doped nanoparticles can be used to activate DNAzymes and increase their intracellular catalytic activity.56
Several vital reviews have confirmed the functions of miRNAs during disease progression, and the potential to serve as a reliable biomarker for disease diagnosis.57,58 To overcome the above barriers, Chu et al. reported DNAzyme logic circuits using Cu/ZIF-8 nanoparticles (NPs) to image multiple microRNAs (miRNAs) in living cells59 (Fig. 3A). Cu2+ could be efficiently released from the pH-responsive Cu/ZIF-8 NPs to serve as a cofactor, which enabled the Cu2+–DNAzyme logic circuits to respond to multiple endogenous miRNAs. Fig. 3B shows great potential of the DNAzyme logic circuits to detect microRNAs. Dong et al. also reported a DNAzyme-based self-driven intracellular sensor consisting of ZIF-8 and DNAzymes for microRNA detection (Fig. 3C).60 The DNAzymes are driven by Zn2+ to cleave the substrate strand for fluorescently imaging intercellular miRNA. Similarly, Li et al. investigated a light-activated walker consisting of a dissociable ZIF-8 for ensuring sufficient internal cofactors (Zn2+) to facilitate the detection of miRNA in living cells.61 Li et al. presented a photo-cage and cofactor self-supported DNAzyme biosensing platform to detect intracellular mRNA with high selectivity and sensitivity.62 The newly proposed biosensor has good analytical performance for a model tumor biomarker (survivin mRNA) with a low detection limit in the pM level (Fig. 3D). Thus, RNA-cleaving DNAzymes have high sensitivity, excellent specificity, and high biostability in sensing miRNAs in living cells by introducing additional cofactors.
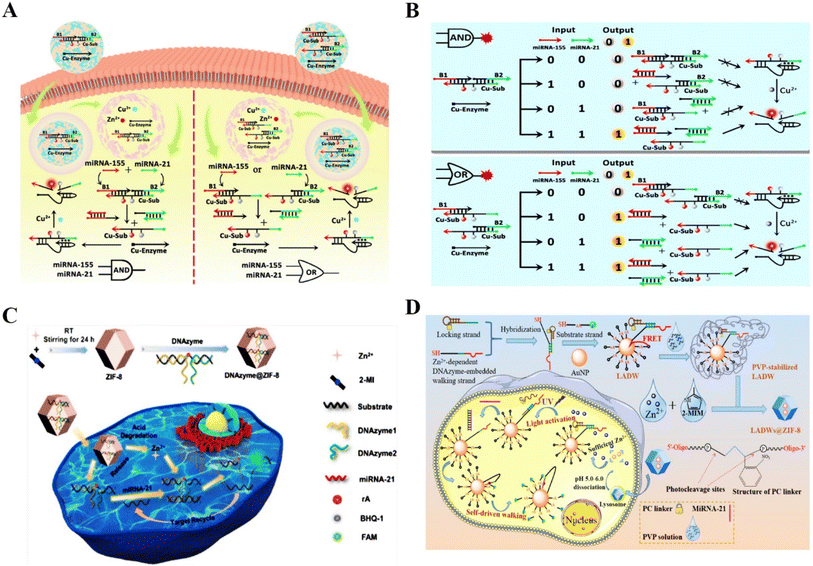 |
| Fig. 3 The application of RNA-cleaving DNAzymes in intracellular sensing. (A) DNAzyme logic circuits for the detection of multiple miRNAs in living cells.59 This figure has been reproduced from ref. 59 with permission from American Chemical Society, copyright 2021. (B) The schematic illustration of Cu2+–DNAzyme-powered YES logic gate for detecting the miRNAs input in A549 cells and HeLa cells.59 This figure has been adapted from ref. 59 with permission from American Chemical Society, copyright 2021. (C) A self-driven intracellular sensor consisted of ZIF-8 and DNAzymes for microRNA detection.60 This figure has been adapted from ref. 60 with permission from American Chemical Society, copyright 2022. (D) A photo-caged and cofactor self-supported 3D DNAzyme biosensing platform for the detection of intracellular mRNA.62 This figure has been reproduced from ref. 62 with permission from American Chemical Society, copyright 2021. | |
In addition to microRNA, adenosine triphosphate (ATP), which is the direct source of energy, plays a vital role in multiple physiological activities.63 DNAzyme-based sensors have emerged as a potential tool for detecting ATP in cancer cells due to their differential expression in cancer cells and normal cells. Liu et al. proposed an ATP-sensing platform by combining MnO2 nanoparticles with a DNA amplifier. The platform could provide adequate Mn2+ cofactors degraded by GSH for precise biosensing of ATP in living cells.64 This novel Mn2+-dependent DNAzyme biosensor could detect low-abundance of endogenous ATP in cancer cells. Dai et al. also reported an ATP/miRNA sensing dual-locked nanosensor using a honeycomb MnO2 nanosponge (hMNS) to image ATP in cancer cells.65 The GSH-degradable MnO2 could supply rich Mn2+ for accurate DNAzyme cleavage (Fig. 4A). Similarly, Liu et al. reported a cascade catalytic amplification strategy using MnO2 nanosponge and DNAzyme for fluorescence detection of ATP.66 Stimulated by overexpressed GSH in cancer cells, the nanoprobe released the cofactor Mn2+, which activated substrate-specific cleavage and generated an optical signal (Fig. 4B). This nanoprobe exhibited satisfactory biosafety and could detect ATP specifically within cancer cells. The above works provided some reliable methods for ATP imaging in cancer cells and opened up a new approach for high-precision disease diagnosis.
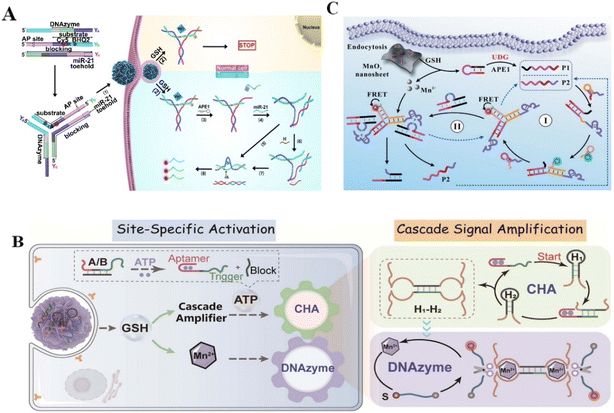 |
| Fig. 4 RNA-cleaving DNAzyme-based biosensors for the detection of other biomarkers.65 (A) This figure has been adapted from ref. 65 with permission from American Chemical Society, copyright 2022. (B) The detection of ATP.66 This figure has been reproduced from ref. 66 with permission from American Chemical Society, copyright 2021. (C) The detection of UDG.25 This figure has been reproduced from ref. 25 with permission from American Chemical Society, Copyright 2021. | |
Furthermore, the detection of protein biomarkers plays a critical role in early diagnosis.67 Nuclear Factor Kappa-B (NF-κB) is a vital mediator of inflammatory pathways and can act as a biomarker for inflammation related disease diagnosis.21,68 However, the low expression of NF-κB in several cell lines limits its recognition. Huang et al. designed a novel biodegradable DNAzyme nanoprobe with cofactor self-supporting properties for detecting the protein in HeLa cells. The Zn2+-dependent DNAzyme nanoprobe showed high-sensitivity in the determination of NF-κB with a low detection limit,69 providing a promising method for efficient imaging of low-abundance biomolecules in living cells. α-Fetoprotein (AFP), a major serum protein produced by the adult hepatic cell, is of great significance for clinical tumor diagnosis.22 Xiang et al. reported a fluorescent probe to this cancer biomarkers.70 As a result, the metal ion-dependent DNAzymes had homogeneous and sensitive detection ability with a limit of 1.8 pM. Moreover, aberrant uracil-DNA glycosylases (UDG) might cause many diseases such as cancers, thus UDG can act as an available biomarker for clinical disease diagnosis. Accurately detecting UDG in living cells is vital for clarifying DNA repair pathways and discovering anti-cancer drugs.71 Zhang et al. constructed an entropy-driven dumbbell-shaped DNAzyme assembly circuit for imaging UDG in living cells and distinguishing cancerous cells from normal cells.72 Targeting UDG to excise damaged uracil released the trigger to form two catalytically active DNAzyme units. The Mg2+–DNAzyme units enhanced the fluorescence signal for detecting intracellular UDG activity, providing a simple and reliable method for biological research and clinical diagnosis. Liu et al. also constructed a DNAzyme nanosystem for imaging intracellular UDG by combining DNAzyme with MnO2 nanosheets.25 In this strategy, intracellular GSH triggered the release of Mn2+ from MnO2 nanosheets, acting as a cofactor to promote catalytic cleavage of substrates (Fig. 4C). The nanosystem realized UDG detection in living cells with enhanced sensitivity (LOD 0.23 mU mL−1). Although DNAzyme-based biosensing is in the primary stage of experimental research, all of the above studies revealed the excellent efficiency of RNA-cleaving DNAzymes in enhanced biosensing and provided a valuable strategy for cellular-level clinical diagnosis and prognosis.
2.3
In vivo biosensing
Although there have been many successful examples of using mRNA-cleaving DNAzyme for sensing diverse targets under different conditions, the applications of these DNAzyme-based probes were restricted by the inefficient in vivo accumulations or insufficient stability of the probes. Fortunately, the recently developed nanoprobes have great potential in solving these obstacles due to their self-protection effect and targeting ability.
Acute heavy metal ion poisoning or long-term ingestion would cause serious pathological changes, thus understanding the spatial-temporal distribution and the role of metal ions in biological systems is particularly important.73 Lu et al. reported a Zn2+-specific near-infrared (NIR) activatable DNAzyme nanoprobe which allowed real-time metal ion tracking of early zebrafish embryos74 (Fig. 5A). As shown in (Fig. 5B), the fluorescence signal inside the zebrafish embryo was significantly enhanced after NIR irradiation, while the same non-cleavable structure showed no fluorescence enhancement. Furthermore, Li et al. proposed an enzyme-activatable DNAzyme sensor for in vivo differentiating metal ion signaling36 (Fig. 5C). Compared to the conventional DNAzyme sensors, the blocking sequence contained a basic site that could be cut by cancer-specific enzymes, thus selectively restoring the inhibited ability of metal ion sensing in cancer cells. Lu et al. reported a Zn2+-selective DNAzyme probe with a protector strand to block the formation of the catalytic domain, the Zn2+-specific fluorescent imaging has been verified by the new DNAzyme based probe in mice (Fig. 5D).75
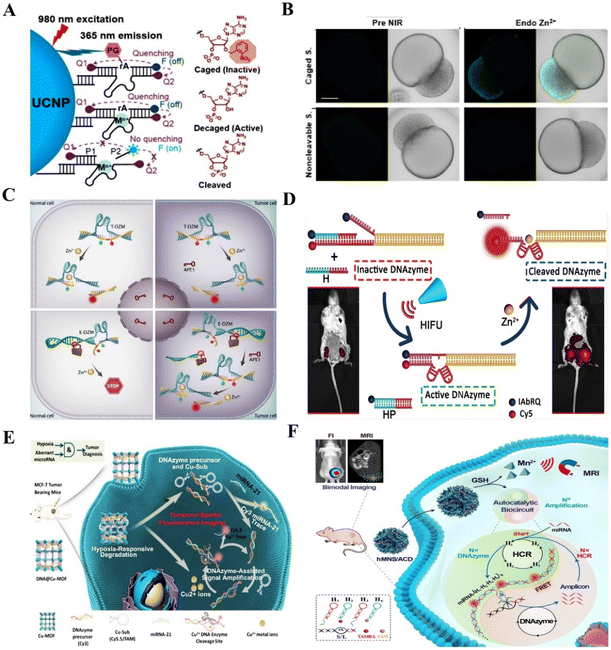 |
| Fig. 5 RNA-cleaving DNAzyme-based biosensors for in vivo sensing. (A) Zn2+-specific NIR activatable DNAzyme nanoprobe for real-time metal ion tracking of early zebrafish embryos.74 This figure has been reproduced from ref. 74 with permission from American Chemical Society, copyright 2018. (B) The fluorescence signal inside the zebrafish embryo.74 This figure has been reproduced from ref. 74 with permission from American Chemical Society, copyright 2018. (C) Enzyme-activatable DNAzyme sensor for in vivo detection of the metal ion.36 This figure has been reproduced from ref. 36 with permission from Wiley-VCH GmbH, Copyright 2022. (D) Noninvasive and spatiotemporal control of DNAzyme-based imaging of metal ions in vivo.75 This figure has been reproduced from ref. 75 with permission from American Chemical Society, Copyright 2022. (E) The multifunctional nanosystem contains a copper metal–organic framework (Cu-MOF) and DNAzymes for detecting miRNA in vivo.30 This figure has been adapted from ref. 30 with permission from Elsevier B.V. All rights reserved, copyright 2021. (F) An autocatalytic DNAzymes biosensor for microRNA imaging in vivo.81 This figure has been reproduced from ref. 81 with permission from Wiley-VCH Verlag GmbH & Co. KGaA, Weinheim, Copyright 2020. | |
MiRNAs have been implicated in the progression of many diseases and have emerged as biomarkers for diagnosis and therapy.76 The aberrant expression of miRNA in combination with tumor microenvironment (TME) characteristics has been extensively investigated for precise tumor imaging.77,78 Although several assays have been applied to zebrafish embryos, the fluorescent signals were dependent on the transparency of early fish larvae, their application was limited to other non-transparent animal models, where the sensitivity and detection limits were disappointing.79 To overcome this barrier, Zhang et al. created a multifunctional nanosystem containing Cu-MOF and DNAzymes for in vivo miRNA detection30 (Fig. 5E). Under hypoxic conditions, the DNA@Cu-MOF nanosystem released Cu2+, DNAzyme precursors, and substrate strands, with a fluorescent signal recovery for miRNA detection. Similarly, Yang et al. developed nanodevices for intracellular and in vivo miRNA detection and manual control of drug release based on upconversion nanoparticles (UCNPs).80 The nanodevices allowed amplified signal for miRNA-21 detection with a lower detection limit of 1.81 × 10−11 M. Furthermore, Wang et al. constructed an autocatalytic DNAzyme amplification bio-scaffold for in vivo microRNA imaging.81 This versatile scaffold not only transported DNA probes but also provided DNAzymes with abundant cofactors (Fig. 5F).
3. RNA-cleaving DNAzymes for gene therapy
Gene therapy is a technique that alters the host's gene to treat diseases. Gene therapy works through several mechanisms, one of which is to inactivate disease-causing genes. RNA-cleaving DNAzyme is a gene therapy tool that offers a “knockdown” approach to produce gene inactivation, which is a powerful tool for performing sequence-specific RNA cutting. In biological systems, gene inactivation by DNAzymes can be carried out at the mRNA level, and then result in the expression inhibition of an unwanted protein. Following the first study of DNAzyme-based targeting inhibition of the Bcr–Abl oncogene in acute lymphoblastic leukemia, DNAzyme has been successfully applied to gene therapy in many clinical diseases related to gene mutation, especially in cancer, cardiovascular disease, and viral infection (Table 2).
Table 2 RNA-cleaving DNAzyme-based gene therapy
Disease |
Target |
Outcome |
Ref. |
Cancer |
C-jun |
Block MRP1 transcription, reverse MDR |
95
|
Block angiogenesis, enhance cell-mediate immunity |
123
|
Downregulate MMP-2 and MMP-9, inhibit cell proliferation, migration, and invasion |
147
|
P-gp |
Reverse MDR |
92
|
MDR1 |
Reverse MDR |
93
|
HSP70 |
Overcome thermoresistance |
101 and 102 |
Survivin |
Promote apoptosis, overcome therapeutic resistance |
103, 109 and 110 |
EGR-1 |
Growth inhibition, enhance PDT therapy |
16 and 111 |
Prevent tumor angiogenesis, promote apoptosis |
136
|
ATG5 |
Enhance autophagy suppression |
113
|
PD-L1 |
Promote T cell activation, reinforce tumor immune microenvironment |
119 and 120 |
β-Catenin |
Improve DCs infiltration and maturation, T cell activation, enhance adaptive immune response |
123
|
VEGFR2 |
Inhibit angiogenesis |
135
|
IGF-II |
Inhibit angiogenesis |
137
|
Twist |
Suppresses EMT, inhibit tumor metastasis |
27
|
Aurora kinase A |
Suppresses EMT, inhibit tumor metastasis |
143
|
MMP-9 |
Prevent ECM degradation |
149
|
Cardiovascular diseases |
EGR-1 |
Suppresses VSMC proliferation, inhibit neointima formation |
155, 156, 158–160 and 170 |
Inhibit inflammation, restore cardiac function |
169 and 171 |
C-jun |
Inhibit VSMC proliferation, inhibit intimal hyperplasia |
157 and 161 |
PAI-1 |
Promote neovascularization, reduce fibrin deposition, inhibited VSMC proliferation and migration, prevent neointima formation |
166–168
|
Viral infection |
HIV-1 integrase |
Inhibit viral replication |
173
|
HIV-1 CCR5 |
Decrease fusion and internalization |
177
|
HIV-1 CXCR-4 |
Decrease fusion and internalization |
178
|
HCV NS5B |
Inhibit HCV RNA replication and translation |
193
|
HCV NS3 |
Inhibit the virus replication |
194
|
HCV IRES |
Inhibit HCV RNA replication and translation |
192, 193 and 195 |
EBV LMP1 |
Inhibit cell proliferation, promote apoptosis |
183 and 184 |
Promote apoptosis, enhance radiosensitivity |
185 and 187–191 |
Induces DNA damage, causes cell cycle arrest |
186
|
Inhibit tumor angiogenesis |
182
|
3.1 DNAzyme-mediated gene therapy in cancer
The progression of cancers involves multiple steps and multiple gene mutations, which induce abnormal signaling pathways.82 In this process, some signaling pathways that determine cell growth, division, and proliferation are improperly activated, while some apoptosis related signaling pathways are blocked, ultimately leading to the occurrence of cancers.83 Although a series of gene therapy strategies targeting these abnormal genes have acquired significant breakthrough in cancer treatment, metastasis, immunosuppression, and treatment resistance is still the main restriction to cure cancers. Given the significant advantages of DNAzymes, the gene intervention strategy using RNA-cleaving DNAzymes is expected to be a promising alternative for solving the above problems in cancer gene therapy.
3.1.1 Reverse treatment resistance.
Treatment resistance, defined by increased tolerance of cancer cells against chemotherapy, radiation therapy, and thermal therapy, has been the main predictor of cancer mortality.84 A variety of reasons contribute to treatment resistance, including multi-drug resistance, apoptosis suppression, specific genetic or epigenetic alterations, enhancing DNA repair in cancer cells, and so on.85–87 Therefore, exploiting the RNA-cleaving DNAzymes to target the treatment resistance-related genes may be a promising therapeutic strategy to enhance the efficacy of cancer therapy. As a kind of innate or acquired resistance, chemoresistance is the main cause of the clinical failure of chemotherapy.88 One of the chemoresistance mechanisms is the overexpression of multidrug resistance (MDR) genes.89 Among their expressed productions, a large superfamily of transporters named ATP-binding cassette (ABC) transporters, such as breast cancer resistance protein (BCRP), P-glycoprotein (P-gp), and multidrug-resistance protein (MRP), can pump out chemotherapy drugs, and reduce the accumulation of drugs in cells.90,91 Therefore, designing MDR-associated ABC transporters targeting DNAzyme is a reliable treatment strategy for reversing MDR. In this aspect, our laboratory constructed a P-gp targeting DNAzyme nanoflowers by the RCA strategy to reverse the MDR of breast cancer. The nanoflowers have better biodegradability than the traditional nucleic acid nanocarriers. The constructed nanoflowers improved the sensitivity of doxorubicin-resistant breast cancer cells (MCF-7/MDR) towards Dox and reversed the P-gp-mediated MDR by silencing the P-gp gene92 (Fig. 6A). Zokaei et al. used chitosan/cyclodextrin/tripolyphosphate nanocarriers to deliver mRNA-cleaving DNAzyme targeting multi-drug resistance gene-1 (MDR1) into MCF-7/MDR cells. The results showed that the nanoplatform could downregulate MDR1 mRNA and sensitize MCF-7/MDR cells to Dox.93 In addition to directly targeting MDR-associated ABC transporters, MDR can also be reversed indirectly by interfering with the expression of other regulators, such as the c-jun protein. Several studies have shown that c-jun, as an important transcription factor, upregulates the expression of ABC transporters such as ABCB1, ABCG2, and ABCC1, leading to increased drug resistance to chemotherapy in tumor cells.94 Sun et al. constructed functionalized mesoporous silica nanoparticles (MSNs) to co-deliver Dox and c-jun targeting DNAzymes for overcoming prostate cancer drug resistance and metastasis. The results showed that the co-delivery system effectively increased intracellular Dox concentration in Dox-resistant PC-3 cells, due to RNA-cleaving DNAzyme-mediated downregulation of c-jun expression and subsequent inhibition of MRP1 expression.95 In a word, although MDR is a thorny problem in cancer chemotherapy, RNA-cleaving DNAzymes show a good synergistic effect by the silencing of drug-resistant genes, showing great application potential in reversing the MDR.
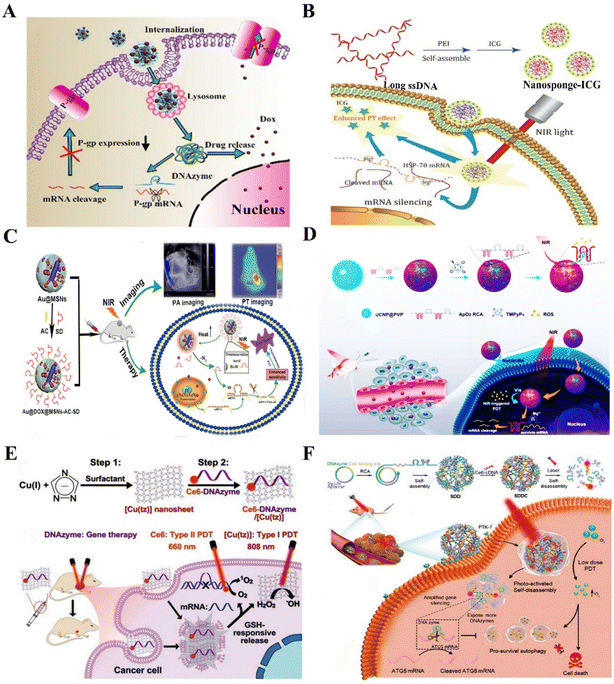 |
| Fig. 6 The DNAzyme-based nanotherapeutic platform for reversing treatment resistance. (A) The DNAzymes NFs targeting P-gp for reversing tumor MDR.92 This figure has been reproduced from ref. 92 with permission from Elsevier, copyright 2021. (B) The nanosponges targeting HSP70 for overcoming thermoresistance.101 This figure has been reproduced from ref. 101 with permission from the author(s), copyright 2020. (C) The nanoplatform targeting survivin for enhanced PTT therapy103 This figure has been reproduced from ref. 103 with permission from the Royal Society of Chemistry, copyright 2018. (D) The DNAzyme-assisted upconversion nanoplatform for enhanced PDT.109 This figure has been reproduced from ref. 109 with permission from American Chemical Society, copyright 2020. (E) The multifunctional nanoplatform for reversing treatment resistance via DNAzyme-mediated EGR-1 gene silencing.112 This figure has been reproduced from ref. 112 with permission from Wiley-VCH GmbH, copyright 2021. (F) Multifunctional DNA nanoflower for enhanced PDT by DNAzyme-mediated ATG5 gene silencing.113 This figure has been reproduced from ref. 113 with permission from Wiley-VCH GmbH, copyright 2021. | |
Thermoresistance is an inescapable problem during the hyperthermia-based treatments of cancer, principally involving photothermal therapy (PTT) and magnetic hyperthermia.96 Although they are promising anticancer strategies to ablate tumors, the accompanied thermoresistance greatly restricts their clinical application.97 Several studies proposed that heat shock proteins (HSPs), especially HSP70 and HSP90, are the key defense molecules of tumor thermoresistance and endow cancer cells with high resistance to heat.98–100 Based on this, our laboratory constructed an HSP70 targeting DNAzyme-based nanoplatform by assembling polyetherimide (PEI), indocyanine green (ICG), and multivalent DNAzymes for reversing thermoresistance in PTT. The nanoplatform presented great gene silencing ability and excellent performance in photothermal conversion, which would sensitize MCF-7 cells to hyperthermia by downregulating the overexpression of HSP70
101 (Fig. 6B). In another work, Xi et al. synthesized a MnO2-coated polydopamine (PDA@MnO2) nanoplatform to deliver HSP70 targeting DNAzymes for enhancing PPT efficiency.102 These works provide promising gene therapy platforms for enhanced PTT via silencing HSP genes. In addition, apoptosis suppressor genes are involved in the occurrence, development, prognosis, and treatment resistance of cancers. Targeting apoptosis suppressor genes will be an effective way to reverse thermoresistance. For example, our laboratory synthesized a NIR-light activated nanoplatform using MSN-coated gold nanorods for delivering survivin targeting DNAzymes. The nanoplatform sensitized triple-negative breast cancer cells to heat by silencing the survivin gene103 (Fig. 6C).
As one emerging tumor treatment strategy, photodynamic therapy (PDT) has been applied in clinical practice. By employing a photosensitizer (PS) and a light source, reactive oxygen species (ROS) are generated to kill the cancer cells. During this process, cancer cells developed various resistance mechanisms to protect themselves from being damaged by ROS, known as PDT resistance.104–106 Abnormal expression of stress-response-related genes, alteration of apoptosis pathways and autophagy, and increased repair of DNA contribute to PDT resistance.107 For example, survivin, an inhibitor of apoptosis (IAP) family protein, is involved in PDT resistance and acts as a potential target for improving PDT.108 Inspired by this point, our laboratory constructed an upconversion PDT nanoplatform by adsorbing a long single-stranded DNA (ssDNA) on the upconversion nanoparticles for precise PDT, which could be triggered by NIR light to generate ROS for enhancing apoptosis of cancer cells. The ssDNA with multiple aptamer AS1411 and survivin targeting DNAzymes endowed the upconversion nanoplatform with high recognition, drug loading, and gene silencing capacity109 (Fig. 6D). Yi et al. developed an enzyme-activatable nanodevice by adsorbing a DNA duplex probe onto MnO2 nanosheets, which endowed the nanodevice with telomerase-triggered Ce6 signal “turn-on” and survivin mRNA silencing ability for enhanced PDT.110 Early-response genes upregulated after PDT-mediated oxidative stress encoded many transcription factors such as EGR-1. A GSH-responsive MnO2 nanoplatform was constructed to deliver TB and EGR-1 targeting DNAzymes for enhanced PDT. The result showed that the nanoplatforms could effectively inhibit the growth of tumors in vivo and in vitro by the combination of PDT and gene therapy.111 To improve the efficacy and safety of gene silencing, Liu et al. synthesized ultrathin 2D nanosheets of Cu(I) 1,2,4-triazolate coordination polymers (CP) to deliver EGR-1 targeting DNAzymes. The 2D CP nanosheets not only have the ability of GSH-responsive DNAzyme release to ensure the DNAzymes with high gene silencing efficiency and stability but also have the performance of photosensitizers. The results showed that 2D CP nanosheets significantly inhibited tumor growth by up to 88.0% in the MCF-7 tumor-bearing mice, indicating the nanoplatforms with the predominant anticancer effect by combining of DNAzyme-based gene therapy and PDT112 (Fig. 6E). In addition, the stimulation of autophagy in apoptosis-competent cells increases cellular resistance to PDT. In light of this problem, Shi et al. synthesized a photoactivated DNA nanoflower (DNF), which consisted of a tumor-targeting Sgc8c aptamer and autophagy-related-genes targeting DNAzymes, and was modified with PS (Ce6). The results confirmed that DNF improved the therapeutic efficiency of low-dose PDT by inhibiting the expression of autophagy gene ATG5 mRNA113 (Fig. 6F). In view of the abovementioned DNAzyme assisted gene silencing strategy, DNAzyme-based nanoplatforms have represented a promising breakthrough in overcoming PDT resistance.
3.1.2 Overcoming the immunosuppressive effect.
Immunotherapy has become the fourth major cancer treatment method after surgery, radiotherapy, and chemotherapy. In the past decade, although significant breakthroughs have been made in immunotherapy, including CAR-T, immune checkpoint inhibitors, vaccines, oncolytic viruses, etc., immunosuppression is a big challenge during cancer immunotherapy.114,115 Programmed cell death protein 1 (PD-1) and its ligand PD-L1 are important immunoregulatory signaling molecules. The PD-1/PD-L1 signaling pathway can affect the activation of T lymphocytes, and play a vital role in the formation of the local tumor immunosuppressive microenvironment. Studies have shown that the blocking PD-1/PD-L1 signaling pathway could overcome the suppression of immune cells and reactivate cell-mediated immune response.116–118 The latest research of RNA-cleaving DNAzymes aiming at targeting PD-1/PD-L1 has yielded some promising outcomes. Interestingly, to improve the poor immune response in the tumor, Liu et al. developed Mn2+/Fe3+ hybrid metal–phenolic networks (MPNs) loaded with anti-PD-L1 DNAzymes for amplified tumor ferroptosis-immunotherapy. In this study, the nanosystem demonstrated excellent synergistic effects including ferroptosis, PTT, immunogenic cell death (ICD), and Mn2+-activated gene silencing of DNAzymes. The results showed that the nanoplatform achieved a higher level of mature DC infiltration, enhanced intratumoral recruitment of both CD4+ T cells and CD8+ T cells, and increased cytokine excretion (IFN-γ, TNF-α, and IL-6), resulting in strong antitumor immunity119 (Fig. 7A). Guo et al. designed a hybrid nanocarrier with a PLGA core and a MOF shell via Fe3+/Mn2+–tannic acid coordination to deliver DPCPX (an adenosine A1 receptor inhibitor) and anti-PD-L1 DNAzyme for reversing the negative effect of adenosine A1 receptor-targeted immunometabolism modulation. The nanosystem showed an obvious ability to downregulate PD-L1 mRNA for promoting CD8+ T lymphocyte infiltration and activation.120 Furthermore, An et al. designed an immunotherapeutic platform composed of a calcium core, aptamer–DNAzyme conjugate (cAD), and a hydrophilic shell (DSPE-PEG2000) for boosting tumor immunotherapy. This nanoplatform has multifunctional characteristics of Ca2+ interference-mediated TME reprogramming and Ca2+-activated PD-L1 silencing. The result showed that the nanoplatform not only prevented primary tumor development and lung metastasis but also offered a long-lasting immunological memory to avoid tumor recurrence121 (Fig. 7B). Du et al. used hollow-MnO2 nanoparticles to co-deliver glycyrrhizic acid and Ce6-labeled-DNAzyme. The nanosystem specifically silenced the PD-L1 gene, which could facilitate T cell activation, simultaneously resulting in immunogenic cell death122 (Fig. 7C). As the most effective antigen-presenting cells (APCs), DCs are closely related to T cell activation and immunotherapy. To improve tumor antigen presentation and DC infiltration, Zhu et al. fabricated a nanovaccine composed of a polyserotonin core and a MOF shell via TA/Mn2+ coordination for β-catenin targeting DNAzyme delivery. DNAzyme-mediated β-catenin mRNA silencing improved DC infiltration and maturation, resulting in better T cell activation and enhancement of adaptive immune response123 (Fig. 7D). In another study, Cai et al. demonstrated that Dz13 (a DNAzyme-targeting c-jun mRNA) could inhibit squamous cell carcinoma growth involving acquired cell-mediated immunity via an increased percentage of CD8+ and CD4+ cells in tumors.124 A subsequent study by Cai, also demonstrated that Dz13 could prevent tumor recurrence through adaptive immunity.125 All these studies highlighted the benefits of combining mRNA-cleaving DNAzymes via multifunctional nanomedicine to enhance cancer immunotherapy.
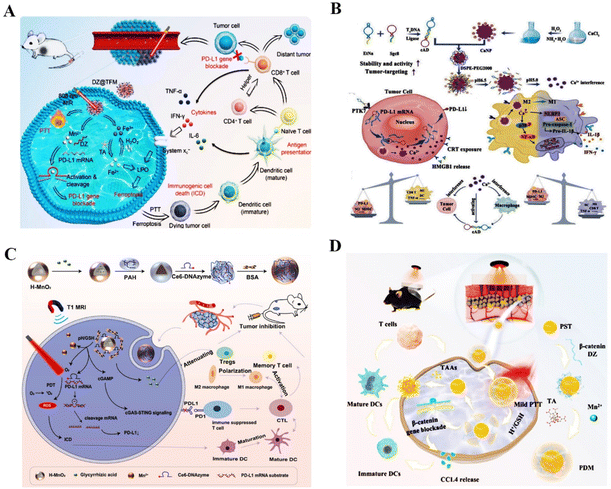 |
| Fig. 7 DNAzyme-based nanotherapeutic platform in tumor immunotherapy therapy. (A) DZ@TFM for PTT-enhanced cyclically amplified tumor ferroptosis-immunotherapy.117 This figure has been adapted from ref. 117 with permission from Advanced Healthcare Materials, Copyright 2021. (B) A bioactive nanoplatform for boosting tumor immunotherapy.71 This figure has been adapted from ref. 71 with permission from Advanced Functional Materials, copyright 2022. (C) The nanosystem for synergistic antitumor therapy of PDT and immunotherapy.119 This figure has been adapted from ref. 119 with permission from Small, copyright 2022. (D) β-Catenin targeting DNAzymes promotes CCL4 excretion and DCs infiltration.120 This figure has been reproduced from ref. 120 with permission from Research, copyright 2022. | |
3.1.3 Inhibition of cancer metastasis.
Metastasis, the most dangerous stage of tumor progression, is the main cause of death.126 Although significant advances have been made in the past decades to reveal the underlying mechanisms and therapeutic targets of cancer metastasis, the inhibition of cancer metastasis has not yet achieved the desired outcome in the clinic. Previous research has demonstrated that angiogenesis, epithelial–mesenchymal transformation (EMT), overexpression of metastasis or invasion-related genes, and destruction of the extracellular matrix (ECM) are implicated in the process of metastasis, and they are considered to be promising targets for gene silencing.127–129
Firstly, angiogenesis is one of the essential processes in cancer metastasis. The development of new blood vessels will provide the tumor with enough nutrition and oxygen to support its growth and spread.130 The essential transcription factors that control tumor angiogenesis and promote metastasis of cancer cells in this process include VEGFR,131 c-jun,132 EGR-1,133 and IGF-II.134 For example, Liu et al. synthesized self-assembled Cu–DNAzyme nanohybrids to co-deliver VEGFR2 targeting DNAzymes and Cu2+ into cancer cells for chemodynamic therapy (CDT) and gene therapy-based combined therapy. This nanoplatform has ultra-high DNAzyme loading and gene silencing capacity, resulting in effective inhibition of the VEGFR2 expression for amplified anti-tumor effects135 (Fig. 8A). In another study, Peng et al. proposed the MnO2–PEI–DNAzyme (MnPD) nanoplatform for self-sufficient anti-angiogenesis and pro-apoptosis combined gene therapy. The dual DNAzyme enabled the downregulation of EGR-1 and survivin mRNAs, effectively inhibiting angiogenesis and promoting cell apoptosis136 (Fig. 8B). Cai et al. demonstrated that the c-jun-targeting DNAzymes could reduce intratumoral vascularity in human primary and metastatic melanoma and prevent tumor recurrence and metastasis through adaptive immunity125 (Fig. 8C). Furthermore, insulin-like growth factor II (IGF-II), a mitogenic peptide, directly promoted angiogenesis in cancer. IGF-II gene targeting DNAzymes could inhibit the invasion, movement, and migration of liver cancer cells.137 All of the above reports demonstrated that angiogenesis-related gene targeting DNAzyme is an alternative tool to inhibit cancer metastasis.
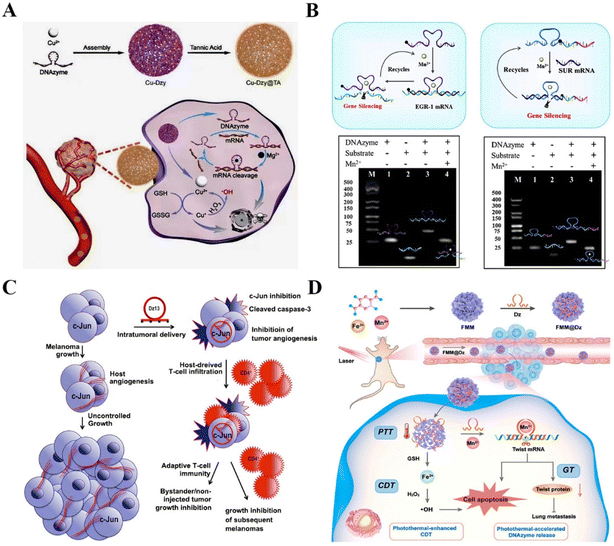 |
| Fig. 8 DNAzyme-based therapeutics for inhibiting cancer metastasis. (A) DNAzyme-based nanoplatform targeting VEGFR2 for enhanced tumor treatment.135 This figure has been reproduced from ref. 135 with permission from Wiley-VCH GmbH, copyright 2021. (B) A versatile nanocomposite with dual gene silencing properties for efficient gene therapy.136 This figure has been reproduced from ref. 136 with permission from Elsevier B.V. All rights reserved, copyright 2022. (C) The targeting c-jun DNAzyme for reducing intratumoral vascularity.137 This figure has been reproduced from ref. 137 with permission from Macmillan Publishers Limited, part of Springer Nature, copyright 2018. (D) The DNAzyme-assisted Fe/Mn-based MOF for improved CDT via DNAzyme-mediated c-jun gene silencing.6 This figure has been reproduced from ref. 6 with permission from American Chemical Society, copyright 2022. | |
EMT is a crucial step in the process of tumor metastasis, which endows cancer cells with metastatic properties by promoting mobility, invasion, and resistance to apoptosis.138 EMT is mediated by some transcription factors, such as Twist,139 Slug,140 and ZEB.141 Among these factors, Twist is verified to be crucial in more invasive human tumors.142 Zhao et al. modified the Fe/Mn-based metal–organic framework (FMM) with DNAzymes to realize improved Twist gene silencing for inhibiting tumor growth and metastasis. The FMM nanoplates not only promoted the efficient delivery and controlled release of DNAzymes but also provided the essential cofactors for DNAzymes to improve catalytic efficiency. The results showed that the nanoplatform is a promising combined gene/chemodynamic therapy system for metastatic cancer therapy6 (Fig. 8D). Another example is Aurora-A kinase, which mediates MAPK-induced metastases via the activation of EMT. Xing et al. constructed the N-acetyl-L-leucine-polyethylenimine to deliver Aurora-A kinase targeting DNAzymes into PC-3 cells. The nanocomplex markedly inhibited the proliferation and migration of human prostate cancer.143
Furthermore, the ECM, one of the major components of tumors, plays multiple key roles in tumor progression, such as mechanical support and regulation of the tumor microenvironment.144 The interaction between cancer cells and the tumor microenvironment (TME) often leads to abnormal ECM, resulting in abnormal mechanical mechanotransduction and further tumor deterioration, even metastasis.145 Several reports suggested that matrix metalloproteinases (MMPs) are involved in the physiological and pathological degradation of the ECM, which is a remarkable feature of metastasis.146,147 Therefore, ECM normalization can serve as a powerful adjuvant for defeating cancer metastasis. Nowadays, DNAzyme-based nanoplatforms targeting ECMs have become a promising strategy to achieve precise, efficient, and safe strategies for inhibiting cancer metastasis and improving poor patient outcomes. Yang et al. developed a tumor-targeting PAMAM derivative to deliver DNAzymes Dz13 for inhibiting cell proliferation, migration, and invasion through the silencing of the c-jun mRNA and subsequently reduced the expression of MMP-2 and MMP-9.148 A similar study reported by Hallett et al. indicated that targeting and silencing of the MMP-9 gene by anti-MMP-9 DNAzyme could inhibit breast cancer cell invasion and metastasis.149 Taken together, these findings demonstrated the remarkable efficiency of DNAzymes in controlling key pathways associated with cancer metastasis and poor patient outcome.
3.2 DNAzyme-mediated gene therapy in cardiovascular diseases
Gene therapy has shown great promise in treating inherited and acquired cardiovascular diseases in the past decade. Current studies have found that cardiovascular disease involved complex pathological mechanisms, including intimal hyperplasia,150 impaired fibrinolysis,151 and inflammation.152 Thus, multiple genes can be used as potential targets for gene therapy of cardiovascular diseases. At present, DNAzyme-mediated gene silencing has brought about significant changes in the treatment of cardiovascular diseases.153,154
Restenosis induced by intimal hyperplasia seriously restricts the therapeutic effect of cardiovascular diseases. It is a restorative response to local vascular injury and remodeling mediated by multiple cytokines.155,156 The proliferation and migration of vascular smooth muscle cells (VSMCs) play a master regulatory role in the pathological mechanism of vascular intimal hyperplasia.157 Research has shown that early growth response-1 (Egr-1) was rapidly upregulated in VSMCs and endothelial cells after pathologic stimulation including ischemia and ischemia-reperfusion injury, resulting in VSMC proliferation and intimal thickening.158 ED5, a 10–23 DNAzyme that specifically cleaved Egr-1 mRNA, was the first to prove the potential therapeutic efficacy of DNAzyme in vivo.158 Similarly, ED5 also inhibited Egr-1 expression and neointima formation following permanent ligation of rat common carotid arteries.156 Another research on ED5 confirmed that it was able to inhibit VSMC proliferation by reducing Egr-1 and proliferating cell nuclear antigen (PCNA) protein expression, indicating that ED5 was an efficient gene therapy approach to prevent restenosis following percutaneous coronary intervention (PCI).155 Furthermore, Lowe et al. first used Egr-1 cleaving DNAzyme to inhibit in-stent restenosis in pig coronary arteries, demonstrating that DNAzyme could specifically inhibit EGR-1 protein expression, and block VSMC proliferation and regrowth after injury.159 In addition, EGR-1 cleaving DNAzymes could also prevent stenosis and occlusion of autogenous vein graft in vivo by inhibiting VSMC proliferation and intimal hyperplasia.160 In addition to EGR-1, c-jun is associated with VSMC proliferation and intimal hyperplasia.161 Khachigian and his colleagues successfully used a DNAzyme (Dz13) to inhibit vein graft stenosis after autogenous end-side transplantation and coronary artery bypass grafting.157,162 All these findings demonstrate that catalytic DNAzymes targeting vital regulatory genes have therapeutic prospects in the clinical management of restenosis.
Furthermore, impaired fibrinolysis is a critical cause of atherothrombotic cardiovascular disease. Plasma plasminogen activator inhibitor (PAI)-1, a major inhibitor of the fibrinolytic system, increases in many clinical pathological states that are associated with a high risk of cardiovascular diseases, such as atherosclerosis, coronary heart disease, angina, and myocardial infarction. Elevated levels of PAI-1 could cause excessive blocking of tissue-plasminogen activator, resulting in decreased clot breakdown and eventually a detrimental blood clot.163–165 Witkowski et al. designed a PAI-1 DNAzyme to treat the lumen of the artery after balloon injury. The PAI-1 DNAzyme not only reduced fibrin deposition both in the arterial lumen and arterial media but also inhibited VSMC proliferation and migration to inhibit the formation of neointimal tissue, successfully preventing post-angioplasty neointima formation.166 Elevated levels of PAI-1 are also associated with the inhibition of tissue microvasculature growth via reduced plasmin-dependent matrix degradation, direct pro-apoptotic effects on pre-formed endothelium, and migration of endothelial progenitors.167 In this case, several researchers have confirmed that reducing cardiac PAI-1 activity by PAI-1 targeting DNAzymes may enhance myocardial neovascularization and improve functional recovery.168
Additionally, several studies have provided powerful evidence that inflammation represents a high-risk factor for the development and progression of cardiovascular diseases. In terms of the molecular mechanism of cardiovascular risk and inflammation, emerging research has demonstrated the role of EGR-1 as an important link between these pathological conditions. Some research groups exploited whether the inhibition of Egr-1 in intracoronary could reduce infarct size after myocardial ischemia-reperfusion by regulating its downstream effector molecules. Bhindi et al. delivered human EGR-1 targeting DNAzymes into intracoronary region in the ischemia-reperfusion (IR) model of pig. They found that DNAzymes reduced the infarct size and improved cardiac functional recovery at the initiation of ischemia-reperfusion (IR) by modulating its downstream effector molecules, such as ICAM-1, TNF-α, PAI-1, and TF.169 Similarly, Bhindi et al. also found that DNAzymes could reduce myocardial infarct size following IR in rats.170 In their further research, Bhindi and his colleague demonstrated that EGR-1 targeting DNAzymes had a protective effect against myocardial IR injury through IL-6-dependent JAK–STAT signaling.171 All these results clearly showed the potential of DNAzyme in attenuating the inflammation of damaged tissue to promote cardiac function.
3.3 DNAzyme-mediated gene therapy in viral infection
Viruses are important pathogens that threaten human health, which are essentially composed of nucleic acid (DNA or RNA) and a protein coat. Nucleic acids are the critical components that constitute the virus genome and provide the genetic information for virus proliferation, heredity, and mutation. Gene-targeting strategies are being developed to silence the key virus genes, where DNAzyme can act as viral mRNA cleavage tools to cause the downregulation of viral proteins. Thus, DNAzymes have been validated in a variety of viral diseases, such as human immunodeficiency virus (HIV) infection, Epstein-Barr virus (EBV), hepatitis B virus (HBV), and hepatitis C virus (HCV).
3.3.1 HIV infection.
HIV is classified into the retrovirus family, and antiretroviral drugs are currently employed as the main medicines for the treatment of HIV infection.172 Although antiretroviral therapy can inhibit the replication of the virus for a period of time, HIV infection cannot be completely eliminated. DNAzyme-based gene therapy can destroy specific targets of viruses to inhibit the invasion of viruses and interfere with viral nucleic acid replication, gene transcription, and translational and post-translational modification.12 For example, HIV integrase is an enzyme responsible for integrating the proviral genome into the human genome and is a prerequisite for HIV replication. Inhibition of the expression of HIV-1 integrase by 10–23 DNAzyme could inhibit viral replication.173 Additionally, HIV-1 requires CCR-5 and CXCR-4 chemokine receptors for fusion and internalization into host cells.174–176 Banerjea et al. used DNAzymes to silence host cell receptor CCR-5 and CXCR-4 mRNA, resulting in decreased fusion of cell membranes.177,178 The result demonstrated that these DNAzymes had the function of interfering with the expression of the chemokine receptors and decreasing viral internalization.
3.3.2 EBV infection.
EBV, a double-stranded DNA herpesvirus, is usually carried lifelong as an asymptomatic infection. EBV has been thought to be associated with the development of several malignancies, such as Burkitt's lymphoma, Hodgkin's disease, and undifferentiated nasopharyngeal carcinoma.179,180 Latent membrane protein 1 (LMP1), a key oncoprotein of EBV, is closely associated with various changes in EBV-associated cancers, including nasopharyngeal carcinoma (NPC). This protein might be responsible for apoptosis inhibition, cell proliferation, immortalization and transformation promotion, and metastasis of cancer cells.181,182 Therefore, suppressing the expression of LMP1 by RNA-cleaving DNAzyme might provide a novel strategy for combating EBV-related diseases. Promising evidence has verified that EBV-encoded LMP1 targeting DNAzyme could inhibit LMP1 protein expression, reduce cell proliferation, promote cancer cell apoptosis, and enhance the radiosensitivity of nasopharyngeal carcinoma by regulating multiple signaling pathways, including NF-κB and JNKs/c-jun signaling pathways.183–190 More importantly, Cao et al., for the first time, carried out the preclinical study of DNAzyme (DZ1) on NPC patients.191 The data confirmed that DNAzyme treatment was safe and well-tolerated to serve as an adjuvant for radiotherapy of NPC.
3.3.3 HCV infection.
Hepatitis C virus (HCV) is a positive-stranded RNA virus with an approximately 9.5 kb linear genome whose replication was mediated by viral RNA polymerase.192 The coding region of HCV consisted of four structural proteins (core protein C, envelope proteins E1 and E2, and P7) and six non-structural proteins (NS2, NS3, S4A, NS4B, NS5A, and NS5B).193 An internal ribosome entry site (IRES) within the mRNA 5′-noncoding region mediates the initiation and controls the translation process of the HCV genome. Moreover, the HCV NS3 protein with serine protease and helicase activity is responsible for the post-translational processing of viral proteins. Therefore, IRES and HCV NS3 are currently the main targets of antiviral gene therapy. Ryoo et al. presented an iron oxide nanoparticle-assisted functional DNAzyme system for the treatment of HCV by silencing the HCV NS3 gene.194 The iron oxide-based DNAzyme delivery system can be tracked through non-invasive magnetic resonance imaging. To overcome the drawback of poor stability of DNAzyme, 2′-C-methyl and 2′-O-methyl-modified 10–23 DNAzymes were designed for targeting the 5′-IRES region of HCV.195 Moreover, DNAzymes can also inhibit viral RNA translation and replication by cleaving other related target RNAs of HCV.192,193
In addition, DNAzyme-mediated gene therapy has also been used for other types of viral infections, including respiratory syncytial virus (RSV),196,197 influenza A virus,198,199 and HBV,200,201 indicating that DNAzyme-based gene therapy is an effective approach for treating a variety of viral infections.
4. Conclusions and outlook
Collectively, exciting progress has already been made in the area of RNA-cleaving DNAzymes. This review systematically summarized the applications of RNA-cleaving DNAzymes in recent years, explaining the uniqueness and superiority of RNA cleaving-DNAzymes in biosensing and gene therapy. RNA-cleaving DNAzymes can cleave RNAs in a sequence-specific manner, which is key to accurate biosensing and gene therapy. Due to the excellent advantages such as high stability, high catalytic activity, easy synthesis, easy functionalization, and modification, the RNA-cleaving DNAyzmes can be incorporated into different biosensors to specifically identify metal ions, neutral molecules, bacteria, and other cofactors. Furthermore, DNAzymes have prominent merits, such as no permanent influence on the genome, no immune responses and significant cytotoxicity, and multiple turnover rates as well as suitability for large-scale rapid production. However, the current application of DNAzyme-based biosensing systems focuses on the laboratory environment, and in vivo detection is still limited by many biological factors. To make DNAzymes more applicable in practical detection, it is necessary to improve the anti-interference ability of the detection system and improve stability in vivo, and more in-depth research is urgently required to solve the above problems. In addition, based on the characteristics of cutting specific sites of RNA, RNA-cleaving DNAzymes have been used to destroy pathogenic RNA in cells or viruses with potential therapeutic effects. Although DNAzymes have a similar function to antisense oligonucleotides, siRNA, and other nucleic acid drugs, they have incomparable properties. DNAzymes have higher gene silencing ability compared to antisense oligonucleotides. Moreover, they have higher stability and lower synthesis cost than siRNA and work independently of the RNA-induced silencing complex (RISC). However, inefficient delivery is an essential problem that hinders the widespread use of DNAzyme-based gene therapy. Nowadays with the rapid development of nanotechnology, new exciting studies for self-supported multifunctional nano-delivery systems to promote the delivery efficiency of DNAzymes are underway. Subsequently, the effectiveness of DNAzymes should be taken into consideration in the future, including the binding efficiency with their targets, the cellular uptake efficiency and stability, and the concentration of local divalent cations that may affect the effectiveness of the final treatment of DNAzyme. In addition, more diseases should be taken into account for RNA-cleaving DNAzyme research. In the last five years, although the use of RNA-cleaving DNAzymes in gene therapy has been explored extensively, most of the diseases being studied are cancers. Therefore, the application of DNAzymes should not be limited to cancer treatment, and wider disease research should be carried out. In fact, the gene therapy of DNAzymes in related diseases is still active in the scientific research stage, and there is still a long distance to clinical application. Many scientific problems need to be further explored and solved, including how to transport DNAzymes from the administration site to the blood circulation, how to overcome the physiological barrier in the body to reach the target organ, how to enter the cells, and how to improve the activity of DNAzymes in vivo. Therefore, we firmly believe that with the in-depth study and the development of novel efficient delivery systems, DNAzyme will certainly play a greater role in diagnostics, enzymology, molecular biology, and other research fields as well as gene therapy in diverse diseases.
Conflicts of interest
There are no conflicts to declare.
Acknowledgements
This work was supported by the National Natural Science Foundation of China (31971304 and 31470961), the Natural Science Foundation of Hebei Province (B2021201005 and B2022201029), and the Hebei Youth Top Talent Project, Science Fund for Creative Research Groups of Nature Science Foundation of Hebei Province (B2021201038).
References
- V. S. A. Jayanthi, A. B. Das and U. Saxena, Biosens. Bioelectron., 2017, 91, 15–23 CrossRef CAS PubMed.
- M. Puiu, C. Nativi and C. Bala, TrAC, Trends Anal. Chem., 2023, 160, 116981 CrossRef CAS.
- J. Wang, K. M. Koo, Y. Wang and M. Trau, Adv. Sci., 2019, 6, 1900730 CrossRef CAS.
- C. Horbinski, K. L. Ligon, P. Brastianos, J. T. Huse, M. Venere, S. Chang, J. Buckner, T. Cloughesy, R. B. Jenkins, C. Giannini, L. B. Nabors, P. Y. Wen, K. J. Aldape, R. V. Lukas, E. Galanis, C. G. Eberhart, D. J. Brat and J. N. Sarkaria, Neuro-Oncology, 2019, 21, 1498–1508 CrossRef CAS PubMed.
- L. W. Chen, Y. F. Liu, W. S. Guo and Z. B. Liu, Macromolecules, 2019, 14, 17862–17870 Search PubMed.
- Y. Zhao, R. Li, J. Sun, Z. Zou, F. Wang and X. Liu, ACS Nano, 2022, 16, 5404–5417 CrossRef CAS PubMed.
- R. R. Breaker and G. F. Joyce, Chem. Biol., 1994, 1, 223–229 CrossRef CAS PubMed.
- K. Jiao, Y. Hao, F. Wang, L. Wang, C. Fan and J. Li, Biosci. Rep., 2021, 7, 21–34 CAS.
- H. Liu, X. Yu, Y. Chen, J. Zhang, B. Wu, L. Zheng, P. Haruehanroengra, R. Wang, S. Li, J. Lin, J. Li, J. Sheng, Z. Huang, J. Ma and J. Gan, Nat. Commun., 2017, 8, 2006 CrossRef PubMed.
- K. Rudeejaroonrung, O. Hanpanich, K. Saito, N. Shimada and A. Maruyama, Biomater. Sci., 2020, 8, 3812–3818 RSC.
- Y. Wang, K. Nguyen, R. C. Spitale and J. C. Chaput, Nat. Chem., 2021, 13, 319–326 CrossRef CAS PubMed.
- J. Yan, M. Ran, X. Shen and H. Zhang, Adv. Mater., 2023, e2300374 CrossRef.
- J. Xin, W. Qin, P. Jiezhou, W. Jin, H. Yunxiang, S. Jiaojiao, C. Mei, H. Xianglian, Z. Yaoyao, W. Bo, W. Yajie, G. Guidong and G. Junling, Chin. Chem. Lett., 2023, 108200 Search PubMed.
- E. M. McConnell, I. Cozma, Q. Mou, J. D. Brennan, Y. Lu and Y. Li, Chem. Soc. Rev., 2021, 50, 8954–8994 RSC.
- J. Shang, S. Yu, R. Li, Y. He, Y. Wang and F. Wang, Nano Lett., 2023, 23, 1386–1394 CrossRef CAS PubMed.
- S.-Y. Liu, Y. Xu, H. Yang, L. Liu, M. Zhao, W. Yin, Y.-T. Xu, Y. Huang, C. Tan, Z. Dai, H. Zhang, J.-P. Zhang and X.-M. Chen, Adv. Mater., 2021, 33, 2108114 Search PubMed.
- Z. Cheng, J. Wei, L. Gu, L. Zou, T. Wang, L. Chen, Y. Li, Y. Yang and P. Li, J. Hazard. Mater., 2022, 431, 128606 CrossRef CAS PubMed.
- S. Khan, B. Burciu, C. D. M. Filipe, Y. Li, K. Dellinger and T. F. Didar, ACS Nano, 2021, 15, 13943–13969 CrossRef CAS PubMed.
- W. Ren, P.-J. J. Huang, D. de Rochambeau, W. J. Moon, J. Zhang, M. Lyu, S. Wang, H. Sleiman and J. Liu, Biosens. Bioelectron., 2020, 165, 112285 CrossRef CAS PubMed.
- X. Zhang, X. Huang, Y. Xu, X. Wang, Z. Guo, X. Huang, Z. Li, J. Shi and X. Zou, Biosens. Bioelectron., 2020, 168, 112544 CrossRef CAS.
- D. D. Ilchovska and D. M. Barrow, Autoimmun. Rev., 2021, 20, 102741 CrossRef CAS.
- M. Zhu, E. Chen, S. Yu, C. Xu, Y. Yu, X. Cao, W. Li, P. Zhang, Y. Wang, Y. Cui, Q. Li and T. Liu, J. Clin. Oncol., 2022, 40, e16094 CrossRef.
- L. Tian, J. Zhang, Y. Zhang, O. Oderinde, C. Li, L. Duan, Y. Wang and J. Cui, Biosens. Bioelectron., 2023, 220, 114879 CrossRef CAS.
- Y. Duan, Y. Song, N. Fan, Y. Yao, S. Deng, S. Ding, B. Shen and Q. Yin, Biosens. Bioelectron., 2022, 197, 113784 CrossRef CAS PubMed.
- X. Song, Q. Ding, J. Zhang, R. Sun, L. Yin, W. Wei, Y. Pu and S. Liu, Anal. Chem., 2021, 93, 13687–13693 CrossRef CAS PubMed.
- L. T. Chu, H. K. Kwong, H. Hartanto and T.-H. Chen, Biosens. Bioelectron., 2022, 218, 114753 CrossRef CAS PubMed.
- D. Li, T. Zhao, J. Chen, J. Shi, J. Wang, Y. Yin, S. Chen, S. Xu and X. Luo, Anal. Chem., 2022, 94, 14467–14474 CrossRef CAS PubMed.
- H. Wang, R. Chen, F. Zhang, Z. Yu, Y. Wang, Z. Tang, L. Yang, X. Tang and B. Xiong, Int. J. Mol. Sci., 2022, 23, 7799 CrossRef CAS PubMed.
- S. Zhang, Y. Luan, M. Xiong, J. Zhang, R. Lake and Y. Lu, ACS Appl. Mater. Interfaces, 2021, 13, 9472–9481 CrossRef CAS.
- X. Meng, K. Zhang, F. Yang, W. Dai, H. Lu, H. Dong and X. Zhang, Anal. Chem., 2020, 92, 8333–8339 CrossRef CAS PubMed.
- J. Shikha, D. Neeraj, S. Nitin Kumar, K. Ajeet, K. Ki-Hyun and K. Sandeep, Chem. Eng. J., 2022, 372, 109677 Search PubMed.
- J. Xu, M. Liu, W. Zhao, S. Wang, M. Gui, H. Li and R. Yu, J. Hazard. Mater., 2022, 429, 128347 CrossRef CAS PubMed.
- M. M. Ali, M. Wolfe, K. Tram, J. Gu, C. D. M. Filipe, Y. Li and J. D. Brennan, Angew. Chem., Int. Ed., 2019, 58, 9907–9911 CrossRef CAS PubMed.
- R. Zhao, L. Zhao, H. Feng, X. Chen, H. Zhang, Y. Bai, F. Feng and S. Shuang, Analyst, 2021, 146, 1340–1345 RSC.
- L. Zhu, X. Lv, Z. Li, H. Shi, Y. Zhang, L. Zhang and J. Yu, Biosens. Bioelectron., 2021, 192, 113524 CrossRef CAS PubMed.
- D. Yi, J. Zhao and L. Li, Angew. Chem., Int. Ed., 2021, 60, 6300–6304 CrossRef CAS PubMed.
- Y. Chang, X. Tang, J. Huang, Y. Chai, Y. Zhuo, H. Li and R. Yuan, Anal. Chem., 2021, 93, 12075–12080 CrossRef CAS PubMed.
- Y. Li, Y. Chang, J. Ma, Z. Wu, R. Yuan and Y. Chai, Anal. Chem., 2019, 91, 6127–6133 CrossRef CAS PubMed.
- J. Liu, Q. Hu, L. Qi, J.-M. Lin and L. Yu, J. Hazard. Mater., 2020, 400, 123218 CrossRef PubMed.
- C. Liu, S. Gou, Y. Bi, Q. Gao, J. Sun, S. Hu and W. Guo, Biosens. Bioelectron., 2022, 210, 114290 CrossRef CAS PubMed.
- X. Song, Y. Wang, S. Liu, X. Zhang, J. Wang, H. Wang, F. Zhang, J. Yu and J. Huang, Microchim. Acta, 2019, 186, 559 CrossRef CAS PubMed.
- J. Zhang, T. Lan and Y. Lu, Annu. Rev. Anal. Chem., 2022, 15, 151–171 CrossRef PubMed.
- L. Zhou, Y. Xiong, H. Wang, A. Yin, X. Zhang, H. Li, Q. Fu and P. Huang, Sens. Actuators, B, 2021, 334, 129589 CrossRef CAS.
- Z. Yu, H. Wang, Y. Zhao, F. Zhang, X. Tang and B. Xiong, Biosensors, 2022, 12, 331 CrossRef CAS PubMed.
- J. Wang, Q. Zhang, Z. Liu, C. Yang, Y. Li, X. Ma, T. Lv and C. Sun, J. Agric. Food Chem., 2022, 12220–12231 CrossRef CAS PubMed.
- H. Xia, J. Huang, X. Lu, G. Wang, Z. Zhang, J. Yue, Q. Li, S. Wang, J. Yan, L. Deng and Y. Xiang, Sens. Actuators, B, 2021, 330, 129255 CrossRef CAS.
- H. Yousefi, S. E. Samani, S. Khan, A. Prasad, A. Shakeri, Y. Li, C. D. M. Filipe and T. F. Didar, ACS Nano, 2021, 29–37 Search PubMed.
- Z. Zhou, Y. Zhang, M. Guo, K. Huang and W. Xu, Biosens. Bioelectron., 2020, 167, 112475 CrossRef CAS PubMed.
- Y. Luo, X. Jiang, R. Zhang, C. Shen, M. Li, Z. Zhao, M. Lv, S. Sun, X. Sun and B. Ying, Small, 2023, e2301146 CrossRef PubMed.
- Z. Tianqing, Q. Xinmin, Z. Wenwen and W. Bryan, Chem, 2023, 1505–1517 Search PubMed.
- L. Chen, H. Huang, Z. Wang, K. Deng and H. Huang, Talanta, 2022, 243, 123352 CrossRef CAS.
- L. Zhang, A. Kan, S. Wang, X. Xu, N. Zhang and W. Jiang, Sens. Actuators, B, 2021, 333, 129551 CrossRef CAS.
- X.-H. Zhao, L.-Z. Zhang, S.-Y. Zhao, X.-H. Cui, L. Gong, R. Zhao, B.-F. Yu and J. Xie, Analyst, 2019, 144, 1982–1987 RSC.
- J. Xiong, C. Dong, J. Zhang, X. Fang, J. Ni, H. Gan, J. Li and C. Song, Biosens. Bioelectron., 2022, 213, 114442 CrossRef CAS PubMed.
- Q. Wang, Z. Wang, Y. He, B. Xiong, Y. Li and F. Wang, TrAC, Trends Anal. Chem., 2023, 159, 116910 CrossRef CAS.
- M. Liu, D. Chang and Y. Li, Acc. Chem. Res., 2017, 50, 2273–2283 CrossRef CAS PubMed.
- J. Cacheux, A. Bancaud, T. Leichle and P. Cordelier, Front. Chem., 2019, 7, 815 CrossRef CAS PubMed.
- K. Chen, Q. Huang, T. Fu, G. Ke, Z. Zhao, X. Zhang and W. Tan, Anal. Chem., 2020, 92, 7404–7408 CrossRef CAS.
- J. Zhang, H. Fu and X. Chu, Anal. Chem., 2021, 93, 14675–14684 CrossRef CAS PubMed.
- J. Su, J. Du, R. Ge, C. Sun, Y. Qiao, W. Wei, X. Pang, Y. Zhang, H. Lu and H. Dong, Anal. Chem., 2022, 94, 13108–13116 CrossRef CAS PubMed.
- J.-L. Gao, Y.-H. Liu, B. Zheng, J.-X. Liu, W.-K. Fang, D. Liu, X.-M. Sun, H.-W. Tang and C.-Y. Li, ACS Appl. Mater. Interfaces, 2021, 13, 31485–31494 CrossRef CAS.
- Y.-H. Liu, J.-L. Gao, J.-X. Liu, D. Liu, W.-K. Fang, B. Zheng, H.-W. Tang and C.-Y. Li, Biosens. Bioelectron., 2021, 190, 113445 CrossRef CAS PubMed.
- D. Zhao, D. Chang, Q. Zhang, Y. Chang, B. Liu, C. Sun, Z. Li, C. Dong, M. Liu and Y. Li, J. Am. Chem. Soc., 2021, 143, 15084–15090 CrossRef CAS PubMed.
- Z. Zou, M. Pan, F. Mo, Q. Jiang, A. Feng, Y. Zhou, F. Wang and X. Liu, Chem. Sci., 2022, 13, 12198–12207 RSC.
- X. Huang, Y. Zhang, J. Chen, L. Zhang, Y. Xu, W. Yin, Y. Shi, X. Zou, S.-Y. Liu and Z. Dai, Anal. Chem., 2022, 94, 12221–12230 CrossRef CAS PubMed.
- Y. Zhou, L. Zou, G. Li, T. Shi, S. Yu, F. Wang and X. Liu, Anal. Chem., 2021, 93, 13960–13966 CrossRef CAS.
- Y. Shen, J. Gong, S. Li, C. Liu, L. Zhou, J. Sheng and Q. Xu, Sens. Actuators, B, 2021, 329, 129078 CrossRef CAS.
- A. Stuckert, A. Arabzade, Y. Zhao, H.-C. Chen and S. Mack, Neuro-Oncology, 2021, 23, i13 CrossRef.
- J. Xu, L. Yao, X. Zhong, K. Hu, S. Zhao and Y. Huang, Talanta, 2023, 253, 123983 CrossRef CAS PubMed.
- M. Zou, D. Li, R. Yuan and Y. Xiang, Biosens. Bioelectron., 2017, 92, 624–629 CrossRef CAS PubMed.
- J. H. J. Hoeijmakers, Nature, 2001, 411, 366–374 CrossRef CAS PubMed.
- Q. Zhang, R. Zhao, C.-C. Li, Y. Zhang, C. Tang, X. Luo, F. Ma and C.-Y. Zhang, Anal. Chem., 2022, 94, 13978–13986 CrossRef CAS PubMed.
- D. Yi, H. Zhao, J. Zhao and L. Li, J. Am. Chem. Soc., 2022, 145, 1678–1685 CrossRef PubMed.
- Z. Yang, K. Y. Loh, Y.-T. Chu, R. Feng, N. S. R. Satyavolu, M. Xiong, S. M. N. Huynh, K. Hwang, L. Li, H. Xing, X. Zhang, Y. R. Chelma, M. Gruebele and Y. Lu, J. Am. Chem. Soc., 2018, 140, 17656–17665 CrossRef CAS PubMed.
- X. Wang, G. Kim, J. L. Chu, T. Song, Z. Yang, W. Guo, X. Shao, M. L. Oelze, K. C. Li and Y. Lu, J. Am. Chem. Soc., 2022, 144, 5812–5819 CrossRef CAS PubMed.
- H. Fan, X. Zhang and Y. Lu, Sci. China: Chem., 2017, 60, 591–601 CrossRef CAS.
- H. Ji, Z. Wang, S. Wang, C. Wang, Y. Chu, H. Liu, Y. Zhang and L. Han, Adv. Funct. Mater., 2023, 2213277 CrossRef CAS.
- S. Kameda, H. Ohno and H. Saito, Nucleic Acids Res., 2023, 51, e24 CrossRef PubMed.
- L. Huang, F. Chen, X. Zong, Q. Lu, C. Wu, Z. Ni, M. Liu and Y. Zhang, Talanta, 2021, 227, 122156 CrossRef CAS.
- Y. Zhou, W. Ma, R. Sun, B. Liu, X. Zhang and H. Yang, Biosens. Bioelectron., 2022, 214, 114549 CrossRef CAS PubMed.
- J. Wei, H. Wang, Q. Wu, X. Gong, K. Ma, X. Liu and F. Wang, Angew. Chem., Int. Ed., 2020, 59, 5965–5971 CrossRef CAS.
- W. Huo, X. Li, B. Wang, H. Zhang, J. Zhang, X. Yang and Y. Jin, Biophys. Rep., 2020, 6, 256–265 CrossRef CAS.
- J.-W. Bai, S.-Q. Qiu and G.-J. Zhang, Signal Transduction Targeted Ther., 2023, 8, 89 CrossRef PubMed.
- R. Perona and I. Sanchez-Perez, Br. J. Cancer, 2004, 90, 573–577 CrossRef CAS PubMed.
- B. Kinnel, S. K. Singh, G. Oprea-Ilies and R. Singh, Cancers, 2023, 15, 1320 CrossRef CAS PubMed.
- P. Mondal and S. M. Meeran, Front. Pharmacol., 2023, 14, 1105484 CrossRef CAS PubMed.
- N. Shahar and S. Larisch, Drug Resistance Updates, 2020, 52, 100712 CrossRef PubMed.
- X. Guo, C. Gao, D.-H. Yang and S. Li, Drug Resistance Updates, 2023, 67, 100937 CrossRef CAS PubMed.
- H. Jiang, D. Guo, D. Chen, Y. Wu, X. Jin and X. Zhu, Biomater. Sci., 2019, 7, 3489–3496 RSC.
- E. L. Giddings, D. P. Champagne, M.-H. Wu, J. M. Laffin, T. M. Thornton, F. Valenca-Pereira, R. Culp-Hill, K. A. Fortner, N. Romero, J. East, P. Cao, H. Arias-Pulido, K. S. Sidhu, B. Silverstrim, Y. Kam, S. Kelley, M. Pereira, S. E. Bates, J. Y. Bunn, S. N. Fiering, D. E. Matthews, R. W. Robey, D. Stich, A. D'Alessandro and M. Rincon, Nat. Commun., 2021, 12, 2082 CrossRef PubMed.
- M. Klabusay, V. Novakova, J. Medalova and A. Konieczna, J. Clin. Oncol., 2016, 34, e14112 Search PubMed.
- L. Liang, W. Huo, B. Wang, L. Cao, H. Huo, Y. Liu, Y. Jin and X. Yang, J. Colloid Interface Sci., 2022, 608, 2985–2993 CrossRef CAS PubMed.
- E. Zokaei, A. Badoei-dalfrad, M. Ansari, Z. Karami, T. Eslaminejad and S. N. Nematollahi-Mahani, Appl. Biochem. Biotechnol., 2019, 187, 708–723 CrossRef CAS PubMed.
- K. M. Hatle, W. Neveu, O. Dienz, S. Rymarchyk, R. Barrantes, S. Hale, N. Farley, K. M. Lounsbury, J. P. Bond, D. Taatjes and M. Rincon, Mol. Cell. Biol., 2007, 27, 2952–2966 CrossRef CAS PubMed.
- S.-P. Sun, C.-P. Liu, I. P. Huang, C.-H. Chu, M.-F. Chung, S.-H. Cheng, S.-Y. Lin and L.-W. Lo, Nano Futures, 2017, 1, 035005 CrossRef.
- J. Wu, X. Cai, G. R. Williams, Z. Meng, W. Zou, L. Yao, B. Hu, Y. Chen and Y. Zheng, Bioact. Mater., 2022, 10, 295–305 CrossRef CAS PubMed.
- L. Cai, R. Ni, X. Ma, R. Huang, Z. Tang, J. Xu, Y. Han, Y. Guo and Z. Gu, Nano Res., 2022, 15, 6361–6371 CrossRef CAS.
- G. Gao, Y.-W. Jiang, Y. Guo, H.-R. Jia, X. Cheng, Y. Deng, X.-W. Yu, Y.-X. Zhu, H.-Y. Guo, W. Sun, X. Liu, J. Zhao, S. Yang, Z.-W. Yu, F. M. S. Raya, G. Liang and F.-G. Wu, Adv. Funct. Mater., 2020, 30, 1909391 CrossRef CAS.
- W. Guo, X. Gao, Q. Zhang, B. Hu, J. Sun, X. Zhao, K. Xu and B. Tang, Anal. Chem., 2023, 95, 4682–4691 CrossRef CAS PubMed.
- Z. Wen, F. Liu, G. Liu, Q. Sun, Y. Zhang, M. Muhammad, Y. Xu, H. Li and S. Sun, J. Colloid Interface Sci., 2021, 590, 290–300 CrossRef CAS PubMed.
- Y. Jin, L. Liang, X. Sun, G. Yu, S. Chen, S. Shi, H. Liu, Z. Li, K. Ge, D. Liu, X. Yang and J. Zhang, NPG Asia Mater., 2018, 10, 373–384 CrossRef CAS.
- Y. Xi, X. Xie, Y. Peng, P. Liu, J. Ding and W. Zhou, Nanoscale, 2021, 13, 5125–5135 RSC.
- X. Sun, Y. Jin, H. Wang, N. Feng, Z. Li, D. Liu, K. Ge, H. Liu, J. Zhang and X. Yang, J. Mater. Chem. B, 2018, 6, 6950–6956 RSC.
- W. Li, X. Guo, F. Kong, H. Zhang, L. Luo, Q. Li, C. Zhu, J. Yang, Y. Du and J. You, J. Controlled Release, 2017, 258, 171–181 CrossRef CAS PubMed.
- J. Zhou, S. Geng, W. Ye, Q. Wang, R. Lou, Q. Yin, B. Du and H. Yao, Pharmacol. Res., 2020, 158, 120621 CrossRef.
- H. Wang, Y. Guo, C. Wang, X. Jiang, H. Liu, A. Yuan, J. Yan, Y. Hu and J. Wu, Biomaterials, 2021, 269, 104885 Search PubMed.
- V. Rapozzi, F. D'Este and L. E. Xodo, J. Porphyrins Phthalocyanines, 2019, 23, 410–418 CrossRef CAS.
- A. Ferrario, N. Rucker, S. Wong, M. Ltma and C. J. Gomer, Cancer Res., 2007, 67, 4989–4995 CrossRef CAS PubMed.
- Y. Jin, H. Wang, X. Li, H. Zhu, D. Sun, X. Sun, H. Liu, Z. Zhang, L. Cao, C. Gao, H. Wang, X.-J. Liang, J. Zhang and X. Yang, ACS Appl. Mater. Interfaces, 2020, 12, 26832–26841 CrossRef CAS PubMed.
- J.-T. Yi, Q.-S. Pan, C. Liu, Y.-L. Hu, T.-T. Chen and X. Chu, Nanoscale, 2020, 12, 10380–10389 RSC.
- X. Wang, J. Dai, X. Wang, Q. Hu, K. Huang, Z. Zhao, X. Lou and F. Xia, Talanta, 2019, 202, 591–599 CrossRef CAS PubMed.
- J. Wang, S. Yu, Q. Wu, X. Gong, S. He, J. Shang, X. Liu and F. Wang, Angew. Chem., Int. Ed., 2021, 60, 10766–10774 CrossRef CAS PubMed.
- J. Shi, D. Wang, Y. Ma, J. Liu, Y. Li, R. Reza, Z. Zhang, J. Liu and K. Zhang, Small, 2021, 17, 2104722 CrossRef CAS.
- E. Alard, A.-B. Butnariu, M. Grillo, C. Kirkham, D. A. Zinovkin, L. Newnham, J. Macciochi and Z. I. Pranjol, Cancers, 2020, 12, 1826 CrossRef CAS PubMed.
- J. C. Del Paggio, Nat. Rev. Clin. Oncol., 2018, 15, 268–270 CrossRef PubMed.
- M. R. Pitter and W. Zou, Cancer Res., 2021, 81, 5141–5143 CrossRef CAS PubMed.
- P. Zatloukalova, M. Pjechova, S. Babcanova, T. R. Hupp and B. Vojtesek, Klin. Onkol., 2016, 29, 72–77 Search PubMed.
- G. Zhulai and E. Oleinik, Scand. J. Immunol., 2022, 95, e13129 CAS.
- P. Liu, X. Shi, Y. Peng, J. Hu, J. Ding and W. Zhou, Adv. Healthcare Mater., 2022, 11, 2102315 CrossRef CAS.
- J. Guo, P. Liu, B. Wei, Y. Peng, J. Ding, H. Zhang, G. Zhang, J. Su, H. Liu, W. Zhou and X. Chen, Nano Today, 2023, 48, 101722 CrossRef CAS.
- J. An, M. Liu, L. Zhao, W. Lu, S. Wu, K. Zhang, J. Liu, Z. Zhang and J. Shi, Adv. Funct. Mater., 2022, 32, 2201275 CrossRef CAS.
- S. Du, C. Chen, S. Qu, H. Song, J. Yang, Y. Li, K. Liu, Q. Lu, W. Luo, R. Wang, X. Guan, Y. Song and X. Han, Small, 2022, 18, e2203942 CrossRef PubMed.
- J. Zhu, R. Chang, B. Wei, Y. Fu, X. Chen, H. Liu and W. Zhou, Research, 2022, 2022, 9816272 CAS.
- H. Cai, F. S. Santiago, L. Prado-Lourenco, B. Wang, M. Patrikakis, M. P. Davenport, G. J. Maghzal, R. Stocker, C. R. Parish, B. H. Chong, G. J. Lieschke, T.-W. Wong, C. N. Chesterman, D. J. Francis, F. J. Moloney, R. S. C. Barnetson, G. M. Halliday and L. M. Khachigian, Sci. Transl. Med., 2012, 4, 139ra82 Search PubMed.
- H. Cai, E.-A. Cho, Y. Li, J. Sockler, C. R. Parish, B. H. Chong, J. Edwards, T. J. Dodds, P. M. Ferguson, J. S. Wilmott, R. A. Scolyer, G. M. Halliday and L. M. Khachigian, Oncogene, 2018, 37, 5115–5126 CrossRef CAS PubMed.
- A. W. Lambert, D. R. Pattabiraman and R. A. Weinberg, Cell, 2017, 168, 670–691 CrossRef CAS PubMed.
- Y. Feng, Z. Liao, H. Zhang, X. Xie, F. You, X. Liao, C. Wu, W. Zhang, H. Yang and Y. Liu, Chem. Eng. J., 2023, 452, 139506 CrossRef CAS.
- I. Pastushenko and C. Blanpain, Trends Cell Biol., 2019, 29, 212–226 CrossRef CAS PubMed.
- Z. Yuan, Y. Li, S. Zhang, X. Wang, H. Dou, X. Yu, Z. Zhang, S. Yang and M. Xiao, Mol. Cancer, 2023, 22, 48 CrossRef CAS PubMed.
- W. C. Cho, G. Jour and P. P. Aung, Semin. Cancer Biol., 2019, 59, 175–186 CrossRef CAS PubMed.
- T. Denize, S. Farah, A. Cimadamore, A. Flaifel, E. Walton, M. A. Sticco-Ivins, C. Labaki, D. A. Braun, M. Sun, E. Wang, W. Xie, T. K. Choueiri and S. Signoretti, Clin. Cancer Res., 2022, 28, 748–755 CrossRef CAS PubMed.
- Z.-y. Lin, G. Chen, Y.-q. Zhang, H.-c. He, Y.-x. Liang, J.-h. Ye, Y.-k. Liang, R.-j. Mo, J.-m. Lu, Y.-j. Zhuo, Y. Zheng, F.-n. Jiang, Z.-d. Han, S.-l. Wu, W.-d. Zhong and C.-l. Wu, Mol. Cancer, 2017, 16, 48 CrossRef PubMed.
- S. Y. Wu, R. Rupaimoole, F. Shen, S. Pradeep, C. V. Pecot, C. Ivan, A. S. Nagaraja, K. M. Gharpure, E. Pham, H. Hatakeyama, M. H. McGuire, M. Haemmerle, V. Vidal-Anaya, C. Olsen, C. Rodriguez-Aguayo, J. Filant, E. A. Ehsanipour, S. M. Herbrich, S. N. Maiti, L. Huang, J. H. Kim, X. Zhang, H.-D. Han, G. N. Armaiz-Pena, E. G. Seviour, S. Tucker, M. Zhang, D. Yang, L. J. N. Cooper, R. Ali-Fehmi, M. Bar-Eli, J.-S. Lee, P. T. Ram, K. A. Baggerly, G. Lopez-Berestein, M.-C. Hung and A. K. Sood, Nat. Commun., 2016, 7, 11169 CrossRef CAS PubMed.
- I. Sandovici, A. Georgopoulou, V. Pérez-García, A. Hufnagel, J. López-Tello, B. Y. H. Lam, S. N. Schiefer, C. Gaudreau, F. Santos, K. Hoelle, G. S. H. Yeo, K. Burling, M. Reiterer, A. L. Fowden, G. J. Burton, C. M. Branco, A. N. Sferruzzi-Perri and M. Constância, Dev. Cell, 2022, 57, 63 CrossRef CAS PubMed.
- C. Liu, Y. Chen, J. Zhao, Y. Wang, Y. Shao, Z. Gu, L. Li and Y. Zhao, Angew. Chem., Int. Ed., 2021, 60, 14324–14328 CrossRef CAS PubMed.
- W. Peng, S. Li, Y. Zhang, Q. Zhang, W. Li, H. Han, D. Zhou, S. Wang, J. Chang and X. Gong, Chem. Eng. J., 2022, 450, 138138 CrossRef CAS.
- Z. Min, H. Zhao, F. Luo, L. Su and W. Shi, Biotechnol. Lett., 2011, 33, 911–917 CrossRef PubMed.
- T. Chen, Y. You, H. Jiang and Z. Z. Wang, J. Cell. Physiol., 2017, 232, 3261–3272 CrossRef CAS.
- J. Meng, S. Chen, J.-X. Han, B. Qian, X.-R. Wang, W.-L. Zhong, Y. Qin, H. Zhang, W.-F. Gao, Y.-Y. Lei, W. Yang, L. Yang, C. Zhang, H.-J. Liu, Y.-R. Liu, H.-G. Zhou, T. Sun and C. Yang, Cancer Res., 2018, 78, 4150–4162 CrossRef CAS PubMed.
- M. V. Recouvreux, M. R. Moldenhauer, K. M. O. Galenkamp, M. Jung, B. James, Y. Zhang, A. Lowy, A. Bagchi and C. Commisso, J. Exp. Med., 2020, 217, e20200388 CrossRef CAS PubMed.
- N. Feldker, F. Ferrazzi, H. Schuhwerk, S. A. Widholz, K. Guenther, I. Frisch, K. Jakob, J. Kleemann, D. Riegel, U. Boenisch, S. Lukassen, R. L. Eccles, C. Schmidl, M. P. Stemmler, T. Brabletz and S. Brabletz, EMBO J., 2020, 39, e103209 CrossRef CAS PubMed.
- Y. Huang, W. Hong and X. Wei, Haematologica, 2022, 15, 129 Search PubMed.
- Z. Xing, S. Gao, Y. Duan, H. Han, L. Li, Y. Yang and Q. Li, Int. J. Nanomed., 2015, 10, 5715–5727 CAS.
- J. F. Hastings, J. N. Skhinas, D. Fey, D. R. Croucher and T. R. Cox, Br. J. Pharmacol., 2019, 176, 82–92 CrossRef CAS PubMed.
- J. Huang, L. Zhang, D. Wan, L. Zhou, S. Zheng, S. Lin and Y. Qiao, Signal Transduction Targeted Ther., 2021, 6, 153 CrossRef PubMed.
- J. Xu, S. Lamouille and R. Derynck, Cell Res., 2009, 19, 156–172 CrossRef CAS PubMed.
- C. B. Brachmann, Y. Zhang, M. Zavodovskaya, J. Hu, J. D. Maltzman, V. Smith, Y. Xiao and S. D. Patterson, J. Clin. Oncol., 2017, 35, 58 Search PubMed.
- J. Yang, J. Zhang, J. Xing, Z. Shi, H. Han and Q. Li, Int. J. Nanomed., 2021, 16, 8117–8119 CrossRef PubMed.
- M. A. Hallett, B. Teng, H. Hasegawa, L. P. Schwab, T. N. Seagroves and T. Pourmotabbed, Breast Cancer Res., 2013, 15, 1–11 CrossRef PubMed.
- A. C. Newby and A. B. Zaltsman, J. Pathol., 2000, 190, 300–309 CrossRef CAS PubMed.
- M. Farag, N. Spinthakis, Y. X. Gue, M. Srinivasan, K. Sullivan, D. Wellsted and D. A. Gorog, Eur. Heart J., 2019, 40, 295–305 CrossRef CAS PubMed.
- P. Libby, Z. Mallat and C. Weyand, Cardiovasc. Res., 2021, 117, 2503–2505 CAS.
- A. A. Fokina, D. A. Stetsenko and J.-C. Francois, Expert Opin. Biol. Ther., 2015, 15, 689–711 CrossRef CAS PubMed.
- W. Zhou, J. Ding and J. Liu, Theranostics, 2017, 7, 1010–1025 CrossRef CAS PubMed.
- J. Zhang, C. Guo, R. Wang, L. Huang, W. Liang, R. Liu and B. Sun, Exp. Ther. Med., 2013, 5, 1371–1374 CrossRef CAS PubMed.
- H. C. Lowe, C. N. Chesterman and L. M. Khachigian, Thromb. Haemostasis, 2002, 87, 134–140 CrossRef CAS.
- Y. Li, R. Bhindi, Z. J. Deng, S. W. Morton, P. T. Hammond and L. M. Khachigian, Int. J. Cardiol., 2013, 168, 3659–3664 CrossRef PubMed.
- F. S. Santiago, H. C. Lowe, M. M. Kavurma, C. N. Chesterman, A. Baker, D. G. Atkins and L. M. Khachigian, Nat. Med., 1999, 5, 1264–1269 CrossRef CAS PubMed.
- H. C. Lowe, R. G. Fahmy, M. M. Kavurma, A. Baker, C. N. Chesterman and L. M. Khachigian, Circ. Res., 2001, 89, 670–677 CrossRef CAS PubMed.
- C. Liu, X. Zhang, S. Wang, M. Cheng, C. Liu, S. Wang, X. Hu and Q. Zhang, BioMed Res. Int., 2013, 2013, 310406 Search PubMed.
- L. M. Khachigian, R. G. Fahmy, G. Zhang, Y. V. Bobryshev and A. Kaniaros, J. Biol. Chem., 2002, 277, 22985–22991 CrossRef CAS PubMed.
- P. C. Saunders, G. Pintucci, C. S. Bizekis, R. Sharony, K. M. Hyman, F. Saponara, F. G. Baumann, E. A. Grossi, S. B. Colvin, P. Mignatti and A. C. Galloway, J. Thorac. Cardiovasc. Surg., 2004, 127, 1276–1284 CrossRef CAS PubMed.
- K. Leander, M. Blomback, H. Wallen and S. He, Thromb. Haemostasis, 2012, 107, 1092–1099 CrossRef CAS PubMed.
- J. A. P. E. Rocha, Fibrinolysis, 1994, 8, 294–303 CrossRef.
- M. H. Prins and J. Hirsh, Am. Heart J., 1991, 122, 545–551 CrossRef CAS PubMed.
- P. Witkowski, T. Seki, G. Xiang, T. Martens, H. Sondermeijer, F. See, G. Bhagat, M. Schuster, M. A. Hardy and S. Itescu, J. Cardiovasc. Pharmacol., 2007, 50, 633–640 CrossRef CAS PubMed.
- G. S. Xiang, M. D. Schuster, T. Seki, A. A. Kocher, S. Eshghi, A. Boyle and S. Itescu, J. Exp. Med., 2004, 200, 1657–1666 CrossRef CAS PubMed.
- G. S. Xiang, M. D. Schuster, T. Seki, P. Witkowski, S. Eshghi and S. Itescu, J. Am. Coll. Cardiol., 2005, 46, 536–541 CrossRef CAS PubMed.
- R. Bhindi, R. G. Fahmy, A. C. McMahon, L. M. Khachigian and H. C. Lowe, J. Pathol., 2012, 227, 157–164 CrossRef CAS PubMed.
- R. Bhindi, L. M. Khachigian and H. C. Lowe, J. Thromb. Haemostasis, 2006, 4, 1479–1483 CrossRef CAS PubMed.
- M. Billah, A. Ridiandries, B. S. Rayner, U. K. Allahwala, A. Dona, L. M. Khachigian and R. Bhindi, Basic Res. Cardiol., 2019, 115, 1–20 Search PubMed.
- H. F. Gunthard, M. S. Saag, C. A. Benson, C. del Rio, J. J. Eron, J. E. Gallant, J. F. Hoy, M. J. Mugavero, P. E. Sax, M. A. Thompson, R. T. Gandhi, R. J. Landovitz, D. M. Smith, D. M. Jacobsen and P. A. Volberding, JAMA, J. Am. Med. Assoc., 2016, 316, 191–210 CrossRef CAS PubMed.
- N. Singh, A. Ranjan, S. Sur, R. Chandra and V. Tandon, J. Biosci., 2012, 37, 493–502 CrossRef CAS PubMed.
- T. Murakami and N. Yamamoto, Tanpakushitsu Kakusan Koso, 1997, 42, 1427–1434 CAS.
- A. S. Fauci, Nature, 1996, 384, 529–534 CrossRef CAS.
- J. S. Cairns and M. P. D'Souza, Nat. Med., 1998, 4, 563–568 CrossRef CAS.
- R. Goila and A. C. Banerjea, FEBS Lett., 1998, 436, 233–238 CrossRef CAS PubMed.
- S. Basu, B. Sriram, R. Goila and A. C. Banerjea, Antiviral Res., 2000, 46, 125–134 CrossRef CAS PubMed.
- G.-L. Bu, C. Xie, Y.-F. Kang, M.-S. Zeng and C. Sun, Viruses, 2022, 14, 2372 CrossRef CAS PubMed.
- K. F. Hui, S. P. T. Yiu, K. P. Tam and A. K. S. Chiang, Front. Oncol., 2019, 9, 81 CrossRef PubMed.
- M. R. Cheerathodi and D. G. Meckes, Jr., Future Virol., 2018, 13, 863–887 CrossRef CAS PubMed.
- W.-H. Liao, L.-F. Yang, X.-Y. Liu, G.-F. Zhou, W.-Z. Jiang, B.-L. Hou, L.-Q. Sun, Y. Cao and X.-Y. Wang, BMC Cancer, 2014, 14, 835 CrossRef PubMed.
- Z. X. Lu, M. Ye, G. R. Yan, Q. Li, M. Tang, L. M. Lee, L. Q. Sun and Y. Cao, Cancer Gene Ther., 2005, 12, 647–654 CrossRef CAS PubMed.
- X. Ke, Y.-c. Yang and S.-l. Hong, Med. Oncol., 2011, 28, S326–S332 CrossRef PubMed.
- L. Yang, Z. Lu, X. Ma, Y. Cao and L.-Q. Sun, Molecules, 2010, 15, 6127–6139 CrossRef CAS PubMed.
- X. Ma, Z. Xu, L. Yang, L. Xiao, M. Tang, J. Lu, S. Xu, Y. Tang, X. Wen, X. Deng, L. Sun and Y. Cao, Int. J. Oncol., 2013, 43, 1541–1548 CrossRef CAS PubMed.
- Z.-X. Lu, X.-Q. Ma, L.-F. Yang, Z.-L. Wang, L. Zeng, Z.-J. Li, X.-N. Li, M. Tang, W. Yi, J.-P. Gong, L.-Q. Sun and Y. Cao, Cancer Lett., 2008, 265, 226–238 CrossRef CAS PubMed.
- X. Ma, L. Yang, L. Xiao, M. Tang, L. Liu, Z. Li, M. Deng, L. Sun and Y. Cao, PLoS One, 2011, 6, 2011 Search PubMed.
- L. Yang, Z. Xu, L. Liu, X. Luo, J. Lu, L. Sun and Y. Cao, Cancer Biol. Ther., 2013, 15, 61–68 CrossRef.
- L. Yang, L. Liu, Z. Xu, W. Liao, D. Feng, X. Dong, S. Xu, L. Xiao, J. Lu, X. Luo, M. Tang, A. M. Bode, Z. Dong, L. Sun and Y. Cao, Oncotarget, 2015, 6, 5804–5817 CrossRef.
- Y. Cao, L. Yang, W. Jiang, X. Wang, W. Liao, G. Tan, Y. Liao, Y. Qu, D. Feng, F. Tang, B. L. Hou, L. Zhang, J. Fu, F. He, X. Liu, W. Jiang, T. Yang and L.-Q. Sun, Mol. Ther., 2014, 22, 371–377 CrossRef CAS.
- S. Roy, N. Gupta, N. Subramanian, T. Mondal, A. C. Banerjea and S. Das, J. Gen. Virol., 2008, 89, 1579–1586 CrossRef CAS.
- D. Kumar, I. Chaudhury, P. Kar and R. H. Das, J. Gastroenterol. Hepatol., 2009, 24, 872–878 CrossRef CAS.
- S.-R. Ryoo, H. Jang, K.-S. Kim, B. Lee, K. B. Kim, Y.-K. Kim, W.-S. Yeo, Y. Lee, D.-E. Kim and D.-H. Min, Biomaterials, 2012, 33, 2754–2761 CrossRef CAS PubMed.
- L. Robaldo, A. Berzal-Herranz, J. M. Montserrat and A. M. Iribarren, ChemMedChem, 2014, 9, 2172–2177 CrossRef CAS PubMed.
- Y.-Y. Xie, X.-D. Zhao, L.-P. Jiang, H.-L. Liu, L.-J. Wang, P. Fang, K.-L. Shen, Z.-D. Xie, Y.-P. Wu and X.-Q. Yang, Antiviral Res., 2006, 71, 31–41 CrossRef CAS PubMed.
- J. Zhou, X.-Q. Yang, Y.-Y. Xie, X.-D. Zhao, L.-P. Jiang, L.-J. Wang and Y.-X. Cui, Virus Res., 2007, 130, 241–248 CrossRef CAS PubMed.
- B. Kumar, M. Khanna, P. Kumar, V. Sood, R. Vyas and A. C. Banerjea, Mol. Biotechnol., 2012, 51, 27–36 CrossRef CAS PubMed.
- Y. Li, L. Wei, A. Cheng, M. Wang, X. Ou, S. Mao, B. Tian, Q. Yang, Y. Wu, S. Zhang, J. Huang, Q. Gao, D. Sun, X. Zhao, R. Jia, M. Liu, D. Zhu, S. Chen, Y. Yu, L. Zhang and L. Pan, Front. Microbiol., 2022, 13, 1064612 CrossRef PubMed.
- W. Hou, Q. Ni, J. Wo, M. Li, K. Liu, L. Chen, Z. Hu, R. Liu and M. Hu, Antiviral Res., 2006, 72, 190–196 CrossRef CAS PubMed.
- J.-E. Wo, X.-L. Wu, L.-F. Zhou, H.-P. Yao, L.-W. Chen and R. H. Dennin, World J. Gastroenterol., 2005, 11, 3504–3507 CrossRef CAS.
Footnote |
† These authors contributed equally to this work. |
|
This journal is © The Royal Society of Chemistry 2023 |