DOI:
10.1039/D2NR07264E
(Paper)
Nanoscale, 2023,
15, 4361-4366
Defect-assisted dynamic multicolor modulation in KLu3F10:Tb crystals for anti-counterfeiting†
Received
28th December 2022
, Accepted 26th January 2023
First published on 27th January 2023
Abstract
Excitation-dependent dynamic multicolor luminescent materials show potential for promising applications in the field of anti-counterfeiting. However, for most ultraviolet (UV)-excited lanthanide-doped materials, more than two types of activators are incorporated to realize multicolors. In this study, for the first time, KLu3F10:Tb crystals were used to realize excitation-dependent multicolor emissions. The morphology was modified by tuning the surface-coated citric acid (CA) content. During hydrothermal reactions, fluorine vacancy defects are formed on the crystal surface, and carboxyl groups (–COOH) are coated on the crystal surface to maintain the charge balance. Under 254 nm UV excitation, typical Tb3+ green emissions were observed, while a strong broadband emission peaking at 442 nm appeared in addition to these Tb3+ emissions under 365 nm excitation. The energy transfer (ET) process between the defect state and Tb3+ is clarified. This work may promote the development of single-type activator-doped multicolor luminescent materials for high-level anti-counterfeiting.
1. Introduction
Lanthanide-doped multicolor luminescent materials show potential for promising applications in anti-counterfeiting, temperature sensing, information encryption, flexible display, bio-detection and bio-imaging.1–6 Several nanoparticles with designed core/shell structure have been used to realize excitation-dependent multicolor upconversion emissions.7–11 For example, the emission color of NaYF4:Yb/Ho/Ce@NaYF4:Yb@NaNdF4:Yb nanoparticles was changed from green to red upon switching the excitation wavelength from 808 nm to 980 nm;12 the observed emission color of NaGdF4:Yb/Tm@NaGdF4@NaYbF4:Nd@Na(Yb,Gd)F4:Ho@NaGdF4 nanoparticles was changed from green to blue when the excitation wavelength was tuned from 808 nm to 976 nm.13 To avoid the use of expensive and dangerous lasers, several UV-excited downshifting materials were employed to control the emission colors. For example, the emission color of Ce/Tb co-doped La9.33(SiO4)6O2 changed from green to blue when the excitation wavelength changed from 229 nm to 315 nm;14 and the output color of Ca2Ba3(PO4)3Cl:Ce/Tb exhibited green light under 229 nm excitation, but it was changed to cyan under 315 nm excitation.15 In these systems, the green light originated from the characteristic transitions of Tb3+ activators, while the blue light originated from the characteristic emission of Ce3+ activators. It is important to develop new UV-excited multicolor materials for advanced applications.
Similar to the lanthanide activators, defects formed in the crystal structure can act as emitters to generate luminescence as well.16–21 The defect luminescence has been reported in pure KRE3F10 (RE = Sm–Lu, Y) nanocrystals. For example, for pure KY3F10 nanocrystals prepared by a CA-assisted hydrothermal method, the strong broadband defect emission centered at 360 nm was recorded under 300 nm UV excitation.22 Under excitation at 395 nm, KEu3F10 nanocrystals exhibited a broadband emission centered at 460 nm.23 However, the origin of defects and the corresponding mechanisms remain unclear. Moreover, inspired by the defect-related photoluminescence process, it is possible to realize dynamic multicolor emissions by incorporating activators into KRE3F10 systems.
In this study, excitation-dependent multicolors are realized in KLu3F10:Tb crystals. Under 254 nm UV excitation, green emissions corresponding to the typical Tb3+:5D4 → 7Fj (j = 6, 5, 4, and 3) transitions were observed. When the excitation wavelength was changed to 365 nm, a strong broadband emission peaking at 442 nm appeared in addition to the Tb3+ emissions. During crystal growth, fluorine vacancies are formed on the crystal surface, which then leads to broad defect luminescence. We further verified that this kind of multicolor product can be used for anti-counterfeiting.
2. Experimental
2.1. Synthesis
2.1.1. Materials.
All raw chemicals including Lu(NO3)3 (99.9%), Tb(NO3)3 (99.9%), NH4F, KOH, and CA were of analytical grade and used directly as received without further refinement. All of the above-mentioned materials were bought from Sigma Aldrich Company. Ethanol was supplied by Sinopharm Chemical Reagent Company.
2.1.2. Synthesis of KLu3F10:15Tb.
A facile hydrothermal method was utilized to synthesize KLu3F10:15Tb. First, 0.85 mmol Lu(NO3)3 and 0.15 mmol Tb(NO3)3 were dissolved in 5 mL deionized water and 15 mL ethanol under vigorous stirring for 15 min. Second, 10 mL deionized water containing 5 mmol KOH, 3.5 mmol NH4F and 1 mmol CA was added to this mixture and vigorously stirred for another 30 min. Finally, the resulting mixture was transferred to a hydrothermal reactor and held at 200 °C for 12 h. The products were washed several times with distilled water and ethanol and dried at 60 °C for 3 h. The other samples studied in this work were synthesized by a similar method.
2.2. Sample characterizations
X-ray diffraction (XRD) patterns of the samples were acquired using a powder diffractometer (Bruker D8 Advance) with Cu-Kα (λ = 1.5405 A) radiation. XRD patterns were recorded in the 2(θ) range of 10° to 80° at a scan rate of 0.02°. The morphology and size of the products were observed using a scanning electron microscope (SEM, FEI Tecnai G2 F20) equipped with an energy-dispersive X-ray spectrometer (EDX, Aztec X-Max 80T). Luminescence spectra and decay curves of the samples were recorded using a fluorescence spectrometer-Fluorolog (Jobin Yvon FL3-211).
3. Results and discussion
As shown in Fig. 1a, the XRD patterns of the KLu3F10 and KLu3F10:15Tb products are well indexed to the standard data of pure cubic phase KYb3F10 (JCPDS no. 27-0462). The XRD peaks shift toward a low angle after doping Tb3+ ions, which is owing to the larger ionic radius of Tb3+ (r = 0.858 nm) than that of Lu3+ (r = 0.848 nm).24 These results also indicate that Tb3+ was successfully incorporated into the host without the emergence of extra impurities.25 Furthermore, since the particle size of KLu3F10 (∼1 μm) is smaller than that of KLu3F10:15Tb (∼4 μm), the XRD diffraction peaks become narrower after the incorporation of Tb3+.26 The products prepared with different CA contents and reaction temperatures were also pure phases (Fig. S1 and S2†). The morphology of the KLu3F10:15Tb product was tuned by adjusting the CA content and reaction temperature. As shown in Fig. 1b, the average particle size of the product without the addition of CA surfactants was about 100 nm. As the CA content increased from 0.3 mmol to 1 mmol, the average particle size increased from ∼300 nm to ∼4 μm, and the shape changed from irregular to spherical (Fig. 1c–e). When the reaction temperature was decreased from 200 to 170 °C, the average particle size reduced from ∼4 to ∼1 μm (Fig. 1f). The energy-dispersive X-ray spectrum suggested the existence of K, Lu, F and Tb elements in the final product (Fig. S3†), and the EDX mapping results further revealed the uniform distribution of these elements in a single particle (Fig. 1g).27
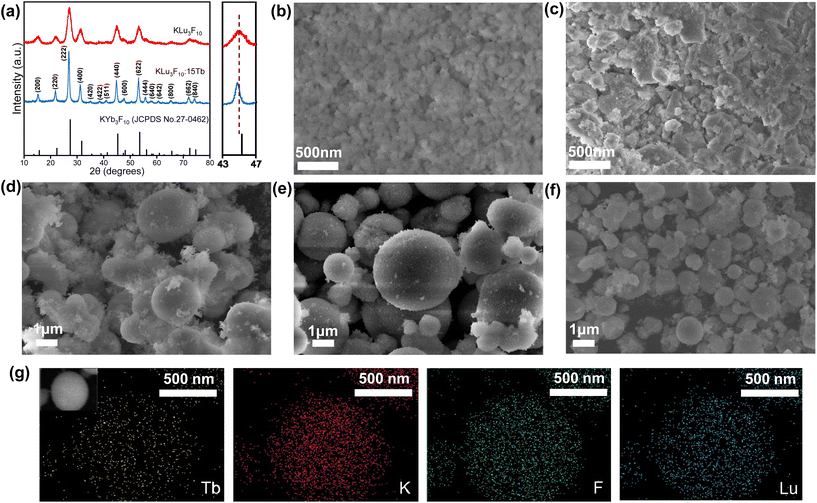 |
| Fig. 1 (a) XRD patterns of the KLu3F10 and KLu3F10:15Tb products. SEM images of the KLu3F10:15Tb products prepared without CA (b), and with 0.3 mmol CA (c), 0.6 mmol CA (d) and 1 mmol CA (e) addition. SEM image (f) and EDX mapping results (g) of the KLu3F10:15Tb products prepared at 170 °C. | |
As shown in Fig. 2a, with monitoring the 545 nm emission of KLu3F10:Tb, a broad photoluminescence excitation (PLE) spectrum corresponding to the defect transition28 as well as few sharp peaks corresponding to the Tb3+:4f–4f transitions were observed. With monitoring the 442 nm emission, only a broad PLE spectrum in the range of 270–400 nm was recorded. Under 254 nm UV excitation, photoluminescence (PL) peaks at 487 nm, 545 nm, 585 nm and 622 nm were assigned to the Tb3+:5D4 → 7Fj (j = 6, 5, 4, and 3) transitions.29 When the excitation wavelength was changed to 365 nm, the intensity of the four characteristic emissions was significantly enhanced. Moreover, a strong broadband emission peaking at 442 nm was observed (Fig. 2b). By fitting the decay curve with a tri-exponential function,30–32 the lifetime of 442 nm was calculated to be 7.93 ns, while that of Tb3+:5D4 → 7F5 (545 nm) was calculated to be 6.06 ms (Fig. 2c and d). The nanosecond lifetime is consistent with the electron's transition in the defect state.33,34 To clarify the origin of this broad emission, pure KLu3F10 crystals were prepared and studied. Under 365 nm excitation, the broad PL emission at 442 nm was also recorded (Fig. 2e). Furthermore, this PL intensity was greatly decreased after annealing at high temperatures. In particular, after annealing at 500 °C, the broad PL fully disappeared (Fig. 2f). These results indicated that the broad PL originated from the defects formed on the surface of the crystals.35,36
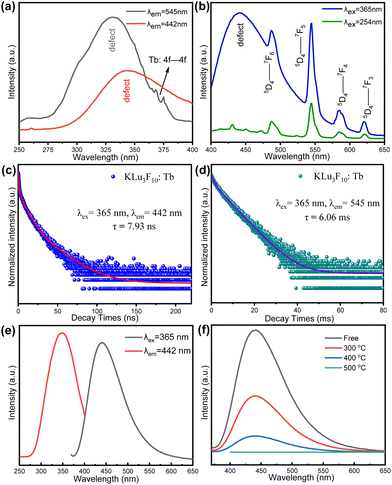 |
| Fig. 2 (a) PLE and (b) PL spectra of KLu3F10:Tb. Decay curves of the 442 nm (c) and 545 nm (d) emissions. (e) PLE and PL spectra of pure KLu3F10. (f) PL spectra of KLu3F10 annealed at different temperatures (free; 300 °C, 3 h; 400 °C, 3 h; 500 °C, 3 h). | |
To further clarify the mechanism for the above-mentioned defect luminescence, the optical properties of KLu3F10:Tb crystals prepared with different CA contents and at different reaction temperatures and times were studied. As shown in Fig. 3a, when the CA content was less than 0.3 mmol, only typical Tb3+ emissions were observed. With increase in CA content to 0.6 mmol, both emissions from Tb3+ and defects were observed. This result suggests that the defect is highly related to the surface CA content. As shown in Fig. 3b–d, with the decrease in the reaction temperature and increase in reaction time, the defect PL intensity was enhanced. Based on these results, a possible mechanism for the formation of defects was schematically illustrated in Fig. 3e. During the crystal growth, fluorine vacancies are formed on the crystal surface. Negatively charged –COOH is coated on the surface to maintain the charge balance. The fluorine vacancies play a role as defect levels to capture electrons, which then leads to defect luminescence. With the decrease in the reaction temperature or increase in the reaction time and CA, the amount of fluorine vacancy and surface coated –COOH increases. As a result, the defect luminescence enhances as well.
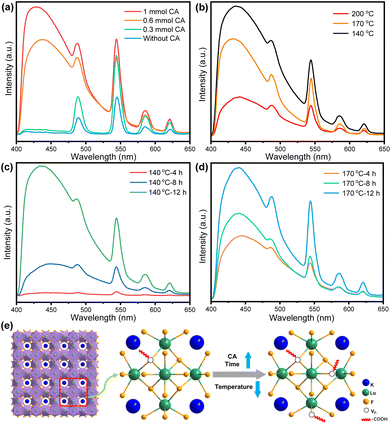 |
| Fig. 3 PL spectra of KLu3F10:15Tb synthesized with different CA contents (a), different reaction temperatures (b), different hydrothermal reaction times at 140 °C (c) and 170 °C (d). (e) Schematic illustration of the proposed defect formation mechanism. | |
To reveal the possible ET process between the defects and Tb3+ activators, the temperature-dependent optical properties of KLu3F10:15Tb were studied. As shown in Fig. 4a and b, with the increase in temperature from 303 to 483 K, the emission intensities of the defects and Tb3+ showed similar decreasing trends. In addition, with the increase in Tb3+ doping concentration, the emission intensity at 545 nm increased gradually, while the defect luminescence at 442 nm decreased gradually (Fig. 4c). The calculated average lifetimes of the defect luminescence decreased from 8.83 ns, 8.3 ns, to 7.89 ns with the increase in Tb3+ concentration from 10, 15 to 20 mmol%, respectively (Fig. 4d–f). These results manifest the existence of ET between the defect states and the Tb3+ excited state. A possible PL mechanism for the excitation-dependent multicolor is shown in Fig. 4g. Under 254 nm UV excitation, the electrons are excited from the ground state (4f8) to the excited state (4f75d), then the 5D3 and 5D4 energy levels are populated via non-radiative relaxation.37 Strong green emissions are produced via5D4–7Fj (j = 3–6) transitions. When the excitation wavelength is changed to 365 nm, the UV input photons are first absorbed by the defects, leading to intense blue emissions. Meanwhile, part of the energy is transferred from the defect state to the Tb3+:5D3 energy level, which leads to green emissions.38
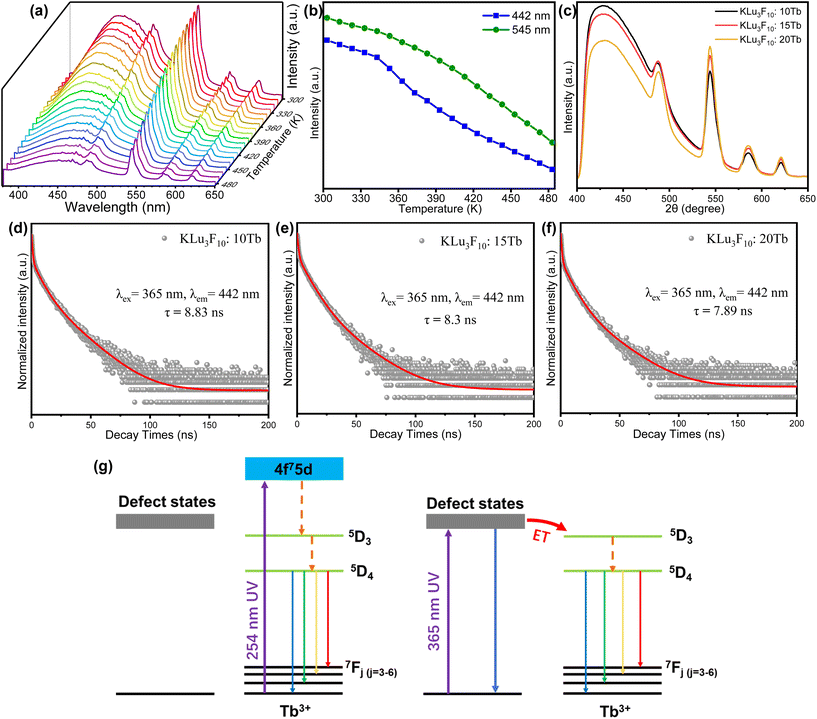 |
| Fig. 4 (a) PL spectra of KLu3F10:15Tb excited at 365 nm under 303–483 K. (b) Variation trend of luminescence intensity at 442 nm and 545 nm under 303–483 K. (c) PL spectra of KLu3F10:xTb (x = 10, 15, and 20). Decay curves of (d) KLu3F10:10Tb, (e) KLu3F10:15Tb and (f) KLu3F10:20Tb at 442 nm. (g) Proposed excitation-dependent PL mechanism. | |
As shown in Fig. 5a, the emission color of KLu3F10:15Tb can be changed from green to bright cyan by adjusting the excitation wavelength. The shift in luminescent color can also be observed clearly in the International Commission on Illumination (CIE) chromaticity diagram of KLu3F10:15Tb (yellow arrow in Fig. S4†). We calculated the ratio of the emission intensity at 442 nm and 545 nm for different wavelength excitations (Fig. 5b). The ratio decreases as the excitation wavelength increases from 250 nm to 290 nm, but increases with the further increase in excitation wavelength to 370 nm. The change in the ratio of the two emission peaks leads to a change in the final luminescence color of the product. To demonstrate the effectiveness of KLu3F10:15Tb in practical anti-counterfeiting applications, we used KLu3F10:15Tb to prepare the letter “L”, the number “20” and circular sector patterns by screen printing technology. The color of these patterns changed significantly when the excitation wavelength was changed from 254 nm to 365 nm (Fig. 5c). These results indicate that the present studied KLu3F10:Tb crystals are potential candidates for anti-counterfeiting labels.
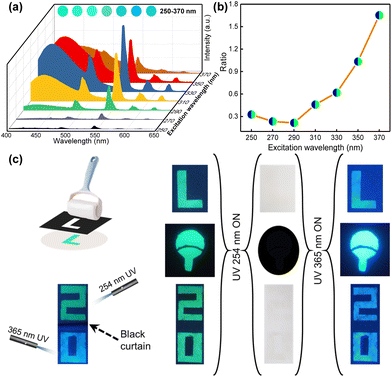 |
| Fig. 5 (a) PL spectra of KLu3F10:15Tb under 250–370 nm excitation. (b) Corresponding luminescence intensity ratio of 442 nm to 545 nm. (c) Screen printing process and luminous patterns irradiated by 254 nm and 365 nm UV lamps (6 W). | |
4. Conclusion
In summary, single Tb3+-doped KLu3F10 crystals prepared by a hydrothermal method exhibited excitation-dependent dynamic multicolor variations. We verified that fluorine vacancies are formed on the crystal surface and –COOH groups are coated on the crystal surface to maintain the charge balance during the hydrothermal reaction. Under 254 nm UV excitation, typical Tb3+ green emissions were observed, while a strong broadband emission peaking at 442 nm appeared in addition to these Tb3+ emissions under 365 nm excitation. The broad band emission originated from the electron transition in the defects. Moreover, the energy transfer from the defect state to Tb3+ is clarified. We further verified that this kind of material can be used for anti-counterfeiting labels. Our results may promote the development of single-type activator-doped multicolor luminescent materials for high-level anti-counterfeiting applications.
Conflicts of interest
There are no conflicts to declare.
Acknowledgements
This work was supported by the Zhejiang Provincial Natural Science Foundation of China (no. LZ20E020001, no. LZ21A040002), the National Natural Science Foundation of China (no. 61875176, 52172164).
References
- J. Xu, B. Zhang, L. Jia, Y. Fan, R. Chen, T. Zhu and B. Liu, ACS Appl. Mater. Interfaces, 2019, 11, 35294–35304 CrossRef CAS.
- Y. Liu, G. Bai, Y. Lyu, Y. Hua, R. Ye, J. Zhang, L. Chen, S. Xu and J. Hao, ACS Nano, 2020, 14, 16003–16012 CrossRef CAS PubMed.
- Y. Zhu, X. Li, Z. Guo, H. Sun, Q. Zhang and X. Hao, J. Am. Ceram. Soc., 2020, 103, 3205–3216 CrossRef CAS.
- M. Yu, Y. Chen, Y. Luo, G. Gong, Y. Zhang, H. Tan, L. Xu and J. Xu, React. Funct. Polym., 2022, 178, 105350 CrossRef CAS.
- S. K. Ray, B. Joshi, S. Ramani, S. Park and J. Hur, J. Alloys Compd., 2022, 892, 162101 CrossRef CAS.
- J. Wang, Y. Zhu, C. A. Grimes and Q. Cai, Nanoscale, 2019, 11, 12497–12501 RSC.
- L. Lei, Y. Wang, W. Xu, R. Ye, Y. Hua, D. Deng, L. Chen, P. N. Prasad and S. Xu, Nat. Commun., 2022, 13, 5739 CrossRef CAS PubMed.
- L. Lei, Y. Wang, A. Kuzmin, Y. Hua, J. Zhao, S. Xu and P. N. Prasad, eLight, 2022, 2, 17 CrossRef.
- H. Dong, L. D. Sun, W. Feng, Y. Gu, F. Li and C. H. Yan, ACS Nano, 2017, 11, 3289–3297 CrossRef CAS.
- P. Hu, S. Zhou, Y. Wang, J. Xu, S. Zhang and J. Fu, Chem. Eng. J., 2022, 431, 133728 CrossRef CAS.
- F. Lin, Z. Sun, M. Jia, A. Zhang, Z. Fu and T. Sheng, J. Lumin., 2020, 218, 116862 CrossRef CAS.
- X. Fu, S. Fu, Q. Lu, J. Zhang, P. Wan, J. Liu, Y. Zhang, C. H. Chen, W. Li, H. Wang and Q. Mei, Nat. Commun., 2022, 13, 4741 CrossRef CAS PubMed.
- H. Wen, H. Zhu, X. Chen, T. F. Hung, B. Wang, G. Zhu, S. F. Yu and F. Wang, Angew. Chem., Int. Ed., 2013, 52, 13419–13423 CrossRef CAS.
- C. Peng, M. Shang, G. Li, Z. Hou, D. Geng and J. Lin, Dalton Trans., 2012, 41, 4780 RSC.
- M. Shang, D. Geng, D. Yang, X. Kang, Y. Zhang and J. Lin, Inorg. Chem., 2013, 52, 3102–3112 CrossRef CAS.
- C. Zhang and J. Lin, Chem. Soc. Rev., 2012, 41, 7938–7961 RSC.
- Y. K. Jung, J. Calbo, J. S. Park, L. D. Whalley, S. Kim and A. Walsh, J. Mater. Chem. A, 2019, 7, 20254–20261 RSC.
- M. A. Reshchikov and H. Morkoç, J. Appl. Phys., 2005, 97, 061301 CrossRef.
- A. Kar, S. Kundu and A. Patra, J. Phys. Chem. C, 2011, 115, 118–124 CrossRef CAS.
- T. P. Mokoena, Z. P. Tshabalala, K. T. Hillie, H. C. Swart and D. E. Motaung, Appl. Surf. Sci., 2020, 525, 146002 CrossRef CAS.
- E. Mitterreiter, B. Schuler, A. Micevic, D. Hernangómez-Pérez, K. Barthelmi, K. A. Cochrane, J. Kiemle, F. Sigger, J. Klein, E. Wong, E. S. Barnard, K. Watanabe, T. Taniguchi, M. Lorke, F. Jahnke, J. J. Finley, A. M. Schwartzberg, D. Y. Qiu, S. Refaely-Abramson, A. W. Holleitner, A. Weber-Bargioni and C. Kastl, Nat. Commun., 2021, 12, 3822 CrossRef CAS PubMed.
- Q. Cui and J. Xu, J. Mater. Sci.: Mater. Electron., 2016, 27, 4372–4377 CrossRef CAS.
- C. Li, Z. Xu, D. Yang, Z. Cheng, Z. Hou, P. a. Ma, H. Lian and J. Lin, CrystEngComm, 2012, 14, 670–678 RSC.
- D. Xu, A. Li, L. Yao, H. Lin, S. Yang and Y. Zhang, Sci. Rep., 2017, 7, 43189 CrossRef CAS.
- G. Amira, B. Chaker and E. Habib, Spectrochim. Acta, Part A, 2017, 177, 164–169 CrossRef CAS PubMed.
- R. Yogamalar, R. Srinivasan, A. Vinu, K. Ariga and A. C. Bose, Solid State Commun., 2009, 149, 1919–1923 CrossRef CAS.
- Y. Cheng, L. Lei, W. Zhu, Y. Wang, H. Guo and S. Xu, Nano Res., 2022 DOI:10.1007/s12274-022-4998-7.
- N. Mendelson, D. Chugh, J. R. Reimers, T. S. Cheng, A. Gottscholl, H. Long, C. J. Mellor, A. Zettl, V. Dyakonov, P. H. Beton, S. V. Novikov, C. Jagadish, H. H. Tan, M. J. Ford, M. Toth, C. Bradac and I. Aharonovich, Nat. Mater., 2020, 20, 321–328 CrossRef PubMed.
- S. Singh and D. Singh, Rare Met., 2020, 40, 3289–3298 CrossRef.
- S. Mukherjee, N. Pathak, D. Das and D. Dutta, RSC Adv., 2021, 11, 5815–5831 RSC.
- Y. Fujimoto, K. Saeki, T. Yanagida, M. Koshimizu and K. Asai, Radiat. Meas., 2017, 106, 151–154 CrossRef CAS.
- K. Jiang, Y. Wang, X. Gao, C. Cai and H. Lin, Angew. Chem., Int. Ed., 2018, 57, 6216–6220 CrossRef CAS PubMed.
- M. Fujimaki, Y. Ohki and H. Nishikawa, J. Appl. Phys., 1997, 81, 1042–1046 CrossRef CAS.
- C. K. Lin, Y. Luo, H. You, Z. Quan, J. Zhang, J. Fang and J. Lin, Chem. Mater., 2005, 18, 458–464 CrossRef.
- Y. Ji, X. Liu, B. Li, J. Zhang and X. Wang, J. Lumin., 2014, 146, 150–156 CrossRef CAS.
- W. Q. Peng, S. C. Qu, G. W. Cong and Z. G. Wang, Mater. Sci. Semicond. Process., 2006, 9, 156–159 CrossRef CAS.
- X. Li, Y. Zhang, D. Geng, J. Lian, G. Zhang, Z. Hou and J. Lin, J. Mater. Chem. C, 2014, 2, 9924–9933 RSC.
- Y. Xie, Z. Ma, L. Liu, Y. Su, H. Zhao, Y. Liu, Z. Zhang, H. Duan, J. Li and E. Xie, Appl. Phys. Lett., 2010, 97, 141916 CrossRef.
|
This journal is © The Royal Society of Chemistry 2023 |