Ligand functionalization on Zr-MOFs enables efficient visible-light-driven H2O2 evolution in pure water†
Received
27th January 2023
, Accepted 15th February 2023
First published on 15th February 2023
Abstract
Different ligand functionalized UiO-66 (UiO-66-X, X = OH, (OH)2 and NH2) were prepared and then modified by ZnIn2S4 lamellas to form ZnIn2S4/UiO-66-X heterostructures for visible-light-driven H2O2 evolution in pure water. The H2O2 yields using ZnIn2S4/UiO-66-NH2, ZnIn2S4/UiO-66-(OH)2 and ZnIn2S4/UiO-66-OH are 799, 733 and 165 μmol L−1, respectively, which are 9.5, 8.7 and 2.0 times that of ZnIn2S4/UiO-66. The high performance of ZnIn2S4/UiO-66-NH2 and ZnIn2S4/UiO-66-(OH)2 is ascribed to the benign visible-light response and Z-scheme heterostructures, and the H2O2 evolution abides by indirect O2 reduction with ˙O2− as an intermediate species. Additionally, H2O2 yields using ZnIn2S4/UiO-66-NH2 on tap water and Xuanwu Lake (Nanjing, China) water can be comparable to that on the above-deionized water. This study sheds light on the great promise of functionalized MOFs and their applications on green (photo)catalytic energy conversion.
1. Introduction
As an eco-friendly and versatile oxidant, hydrogen peroxide (H2O2) is of great significance in various areas, such as disinfection, pulp bleaching, organic synthesis and effluent treatment.1,2 Additionally, on account of its high energy capacity, facile storage and transportation, H2O2 has been deemed as a promising and ideal liquid fuel.3 Therefore, the market demand for H2O2 all over the world is pretty huge and will continuously increase. Currently, the main strategy to produce H2O2 in industry is the anthraquinone method, which undergoes consecutive hydrogenation and oxidation reactions, inducing large amounts of toxic byproduct generation and high energy input.4 Within this context, photocatalytic evolution of H2O2 from O2 reduction has drawn plenty of interest for the last few years.5,6 In this respect, much effort on traditional semiconductors like CdS,7 g-C3N4,8 Bi2MoO6 (ref. 9) and BiVO4 (ref. 3) as photocatalysts was devoted to H2O2 generation and indeed, their corresponding performances were admirable. Nonetheless, most of these reactions were performed in the presence of sacrificial agents (alcohols) and pure O2, which makes the consequent separation of H2O2 difficult and requires extra energy input, consequently going against green-chemistry principles. Additionally, these photocatalysts normally have a low O2 capture/adsorption capacity due to their limited surface areas.
Thanks to an inherent large surface area, high porosity, tailorable structure and semiconducting behavior,10–12 metal–organic frameworks (MOFs) are vastly acclaimed in gas-associated photocatalysis, like photocatalytic CO2 reduction13 and N2 fixation.14 Whereas, MOFs utilized in H2O2 generation from O2 photocatalytic reduction received only sporadic attention.15 Yamashita and co-workers constructed hydrophobic MIL-125-NH2via ligand16 or metal cluster17 alkylation and achieved H2O2 production in a benzyl alcohol/water two-phase system, and later perylenetetracarboxylic diimide was grafted on MIL-125-NH2 for H2O2 production in water.5 Additionally, MOF/semiconductor hybrids like MIL-125-NH2(TiO2)/Ti3C2,18 ZIF-8/g-C3N4 (ref. 19) and MIL-125-NH2@ZnS (ref. 20) were also fabricated for H2O2 photocatalytic evolution.
Ligand decoration on MOFs, especially MOFs with terephthalic acid as ligands, could facilely and effectively regulate their physicochemical properties, conferring charming versatility.10 The common route for ligand decoration is a substitution by functional groups, such as electron-donating amino and hydroxyl groups. The reasons are as follows: (1) uncoordinated hydroxyl and amino groups could serve as additional active sites to participate in the adsorption and activation of reactants,21,22 and may also induce interactions with guest molecules when using MOFs as hosts to prepare adsorbents or catalysts;23 (2) amino or hydroxyl substituents could serve as auxochromic and bathochromic groups in aromatic rings, rendering a redshift of light absorption;24,25 (3) amino and hydroxyl groups are hydrophilic, which could promote the reaction in water. Given such a circumstance, it is believed that amino or hydroxyl-functionalized MOFs could possess great potential for photocatalytic H2O2 generation under visible light.
Considering the unprecedented thermal, chemical and mechanical stability, UiO-66, a zirconium-based MOF (each Zr6O4(OH)4 cluster coordinates with 12 organic ligands),26 was selected as the matrix. A series of UiO-66-X (X = OH, (OH)2 and NH2) was synthesized through a facile solvothermal method and then decorated by ZnIn2S4 layers for visible-light-driven H2O2 evolution in pure water and ambient air. ZnIn2S4 is a typical layered semiconductor with favorable chemical and photostability, enviable visible-light absorption and delicate band configuration with a strong reduction ability that can reduce O2 into H2O2.27,28 Thereby, ZnIn2S4 was adopted to sensitize UiO-66-X and constructed heterostructures to optimize the photocatalytic performances. On account of the favorable visible-light capture and unique Z-scheme heterostructures, ZnIn2S4 modified UiO-66-NH2 and UiO-66-(OH)2 performed remarkable activities for photocatalytic H2O2 evolution. This study would motivate the development of MOF functionalization in green photo- or electrocatalysis.
2. Experimental section
2.1 Materials
Zirconium chloride (ZrCl4, 98%), 2-hydroxyterephthalic acid (≥98%) and 2,5-dihydroxyterephthalic acid (≥98%) were bought from Aladdin Industrial Company. N,N-Dimethylformamide (DMF), acetic acid, terephthalic acid (≥99%), ethanol, zinc acetate dihydrate (≥99%, Zn(CH3COO)2·2H2O) and thioacetamide (TAA, ≥99%) were obtained from Sinopharm Chemical Reagent. 2-Aminoterephthalic acid (99%) was purchased from Sigma-Aldrich. Indium chloride tetrahydrate (InCl3·4H2O, ≥99%) was bought from Shanghai Macklin.
2.2 Preparation of functionalized Zr-MOFs
UiO-66-OH, UiO-66-(OH)2 and UiO-66-NH2 were prepared based on our previous report.26 Typically for UiO-66-NH2, 190.3 mg of ZrCl4 (0.8 mmol), 147 mg of 2-aminoterephthalic acid (0.8 mmol) and 9.6 g of acetic acid (160 mmol) were added into 81.7 mL of DMF. After ultrasound treatment for 20 min and stirring for 2 h, the solution was transferred into a 150-mL autoclave and kept at 120 °C for 24 h. The products were collected by centrifugation, rinsed with DMF and methanol and finally dried at 80 °C overnight. UiO-66-OH, UiO-66-(OH)2 and UiO-66 were prepared under the same conditions except that 2-aminoterephthalic acid was replaced by equimolar 2-hydroxyterephthalic acid, 2,5-dihydroxyterephthalic acid and terephthalic acid, respectively.
2.3 Zr-MOFs modified by ZnIn2S4 lamellas
In a typical synthesis, 169.3 mg of functionalized Zr-MOFs were dispersed in 15 mL of ethanol with ultrasound for 0.5 h. Meanwhile, 87.8 mg of Zn(Ac)2·2H2O, 234.6 mg of InCl3·4H2O and 120.2 mg of TAA were dissolved into 15 mL of deionized water with stirring for 0.5 h. Subsequently, the above aqueous solution was dropped slowly into the ethanol suspension. After stirring for 2 h, the suspension was transferred into a 50 mL autoclave and kept at 180 °C for 24 h. The products were gathered by centrifugation, rinsed with water and ethanol and finally dried at 80 °C overnight. The obtained ZnIn2S4/Zr-MOFs samples (50 wt% of ZnIn2S4/Zr-MOFs) were named Z-UOH, Z-U(OH)2 and Z-UN, respectively. As comparisons, ZnIn2S4/UiO-66 (Z-U) and bare ZnIn2S4 nanosheets were also prepared under the same conditions with UiO-66 and without any Zr-MOFs, respectively.
2.4 Characterizations
X-ray diffraction (XRD) patterns were obtained on a Rigaku Ultima IV instrument. N2 adsorption–desorption isotherms were measured using a Micromeritics TriStar II equipment, and corresponding specific surface areas were determined by the Brunauer–Emmett–Teller (BET) method. Scanning electron microscopy (SEM) and energy dispersive X-ray spectrometry (EDS) mapping were carried out with a Hitachi Regulus 8100 instrument. Transmission electron microscopy (TEM) was performed using JEOL JEM-1400 and JEM-2100 instruments. UV-vis diffuse reflectance spectra (DRS) were recorded on a Shimadzu UV-2600 spectrophotometer. Fourier transform infrared spectroscopy (FTIR) was conducted on a Thermo Electron Nicolet-360 instrument. Water contact angles were measured using a contact meter (KRUSS CAT, Germany). X-ray photoelectron spectroscopy (XPS) was executed on an AXIS UltraDLD instrument. Photocurrent, electrochemical impedance spectroscopy (EIS) and Mott–Schottky plots were tested using a CHI-760E electrochemical workstation. Electron paramagnetic resonance (EPR) was performed using a Bruker EMXPLUS spectrometer.
2.5 Photocatalytic synthesis of H2O2
In a typical reaction setup, 20 mg of photocatalyst powder was dispersed in 40 mL of deionized water with stirring for 30 min in the dark. The photocatalytic reaction was initiated by irradiation of a 300 W xenon lamp (400–780 nm, 350 mW cm−2) in ambient air. At designated time points, a certain suspension was taken and filtrated. H2O2 concentration was determined using the UV-2600 spectrophotometer at 350 nm according to iodometry (details in ESI†). The apparent quantum yield (AQY) at different irradiation wavelengths was determined using:
Meanwhile, the solar-to-H2O2 (STH) conversion efficiency was determined using:
where Δ
G(H
2O
2) is the free energy for H
2O
2 formation (117 kJ mol
−1), I is the light intensity (100 mW cm
−2) of simulated solar light,
V is the volume of suspension,
A is the irradiation area, and
t is the reaction time.
3. Results and discussion
3.1 Photocatalytic performance on H2O2 evolution
The prepared ZnIn2S4/UiO-66-X photocatalysts were evaluated to catalyze H2O2 generation under visible light in pure water and ambient air. UiO-66-NH2 and ZnIn2S4 have minimal H2O2 evolution after 2 h illumination (9 μmol L−1 for UiO-66-NH2 and 23 μmol L−1 for ZnIn2S4, Fig. 1a), presumably due to the limited active sites and sluggish charge separation. After ZnIn2S4in situ growth on UiO-66-NH2, H2O2 evolution experienced a pronounced improvement for all ZnIn2S4/UiO-66-NH2 hybrids without exception. Thereinto, the ZnIn2S4/UiO-66-NH2 hybrid with 50 wt% of UiO-66-NH2 performed the highest H2O2 evolution. Thus, this mass ratio was selected to prepare other ZnIn2S4/UiO-66-X photocatalysts, and their corresponding activities for H2O2 generation were displayed in Fig. 1b. The performance of Z-U is inferior. Whereas, the H2O2 yields catalyzed by Z-UN, Z-U(OH)2 and Z-UOH are 799, 733 and 165 μmol L−1, which are 9.5, 8.7 and 2.0-fold enhancements compared with that of Z-U, respectively, affirming that the functionalization of UiO-66 by amino or hydroxyl groups could switch on the efficient photocatalytic generation of H2O2. Generally, the addition of sacrificial agents like alcohols and extra O2 input are two prerequisites for efficient and continuous H2O2 generation by photocatalysis, yet is going against the green-chemistry principles. Comparatively, the H2O2 yield using Z-UN in pure water and ambient air can be even preferable to those of most MOF-based photocatalysts reacting with sacrificial agents and extra O2 input (Table S1†), and Z-UN possesses favorable cycling performance (Fig. S1†). To study the effect of H2O2 decomposition, decomposition experiments with an initial H2O2 concentration of 1000 μmol L−1 were conducted over ZnIn2S4 and Z-UN (Fig. S2†). The H2O2 decomposition rate is greater than the generation rate after 0.5 h illumination over ZnIn2S4. While for Z-UN, the generation rate is greater than the decomposition rate until 1.5 h illumination and close to the decomposition rate after 1.5 h illumination, demonstrating that the combination with MOFs promotes the H2O2 formation apparently.
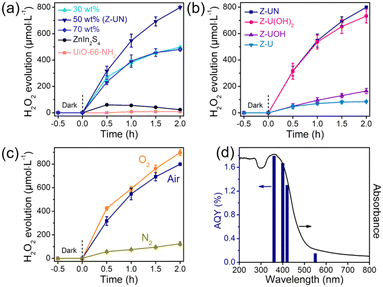 |
| Fig. 1 Photocatalytic H2O2 evolution over ZnIn2S4, UiO-66-NH2 and ZnIn2S4/UiO-66-NH2 hybrids with 30, 50, 70 wt% of UiO-66-NH2 (a), Z-U, Z-UOH, Z-U(OH)2 and Z-UN (b), photocatalytic H2O2 evolution over Z-UN in O2, air and N2 (c), AQYs of photocatalytic H2O2 evolution using Z-UN at different wavelengths and UV-vis DRS of Z-UN (d). Reaction conditions: 20 mg photocatalysts, 40 mL deionized water, 400–780 nm illumination for (a)–(c), ambient air for (a), (b) and (d), ambient temperature. | |
For comparison, 10 vol% ethanol was utilized to replace deionized water as a solution for photocatalytic H2O2 evolution (Fig. S3†), and the yield was boosted to 918 μmol L−1 using Z-UN on account of the introduction of sacrificial agents. Of note, the H2O2 yields using tap water and Xuanwu Lake (Nanjing, China) water can be comparable to that of using deionized water (Fig. S3†), which further improves the economy and sustainability. Meanwhile, reactions in O2 and N2 were also conducted using Z-UN (Fig. 1c). By comparison, O2 promoted the H2O2 generation while N2 dramatically inhibited it, shedding light on that H2O2 photocatalytic generation stems mainly from O2 reduction. H2O2 evolution in air is close to that in O2, implying that enough O2 in air was adsorbed and attached on Z-UN. The AQYs of photocatalytic H2O2 evolution using Z-UN at wavelengths of 360, 400, 420 and 550 nm in pure water and ambient air are 1.79, 1.67, 1.3 and 0.15, respectively, which is in accord with the UV-vis DRS trend of Z-UN (Fig. 1d), indicating good light utilization. Additionally, H2O2 generation can still reach 717 μmol L−1 under simulated solar light (light filter: AM1.5, light intensity: 100 mW cm−1, Fig. S4†), and the corresponding STH is calculated as 0.024%.
3.2 Structure characterizations of the prepared ZnIn2S4/UiO-66-X
The preparation processes of the ZnIn2S4/UiO-66-X hybrids were displayed in Fig. 2a, and the only difference in these processes is the organic ligands of UiO-66-X. The XRD pattern of ZnIn2S4 has four characteristic peaks at 21.7, 27.5, 30.3 and 47.1°, ascribing to the (006), (102), (104) and (110) crystal planes of hexagonal ZnIn2S4 (Fig. 2b).29 All functionalized UiO-66 has similar characteristic peaks compared with those of UiO-66, indicating that they are topologically equivalent to the face-centered cubic lattice of the UiO-66 structure and this result is in line with the previous reports.30,31 Evidently, the peaks attributed to ZnIn2S4 and UiO-66-X appeared on each pattern of the ZnIn2S4/UiO-66-X composites, demonstrating their successful integrations (Fig. 2c and S5†). The weak XRD peaks of UiO-66-(OH)2 are triggered by its low crystallinity. In addition, the good stability of Z-UN before and after photocatalytic H2O2 evolution was also verified by XRD (Fig. S6†). The functional groups were identified by FTIR, and the vibration bands between UiO-66-X and the corresponding ZnIn2S4/UiO-66-X are similar (Fig. S7, S8† and 2d). All hybrids show the common bands at 1579, 1506 and 665 cm−1, which are initiated by the vibrations of carboxylate groups from the terephthalic ligand, C
C bonds from the benzene ring and Zr–O bonds from Zr6 clusters, respectively.24 Two bands at 1621 and 1259 cm−1 in the spectrum of Z-UN separately correspond to the N–H bending vibration and C–N stretching vibration, demonstrating the presence of amino groups.32 In the case of Z-U(OH)2 and Z-UOH, the ν(O–H) bands and C–O stretching of hydroxyl on the benzene rings could be found at 1633 and 1238 cm−1.33 The XRD and FTIR results collectively validate the successful preparation of functionalized UiO-66 decorated by ZnIn2S4. It is known that the hydroxyl and amino functionalization would enhance the hydrophilicity. To determine this, water contact angles of Z-U, Z-UOH, Z-U(OH)2 and Z-UN were measured (Fig. S9†). All samples performed good hydrophilicity because of plenty of hydroxyl groups in the metal cluster (Zr6O4(OH)4) of Zr-MOFs. Even so, the hydroxyl and amino functionalization could still improve the hydrophilicity to a certain degree, especially for Z-U(OH)2. The reinforced hydrophilicity is conducive to the H2O2 generation in water.
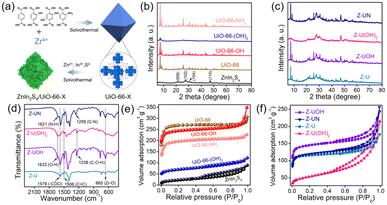 |
| Fig. 2 Preparation diagram of ZnIn2S4/UiO-66-X hybrids (a), XRD patterns (b and c) and N2 adsorption–desorption isotherms (e and f) of UiO-66-NH2, UiO-66-(OH)2, UiO-66-OH, UiO-66, ZnIn2S4, Z-UN, Z-U(OH)2, Z-UOH and Z-U, FTIR spectra of Z-UN, Z-U(OH)2, Z-UOH and Z-U (d). | |
MOFs are known for their large surface area and high porosity,34 which are advantageous for mass transfer, O2 adsorption and attachment. The BET surface areas of UiO-66, UiO-66-OH, UiO-66-NH2 and UiO-66-(OH)2 were determined as 928, 745, 587 and 221 m2 g−1, respectively (Fig. 2e). In contrast with UiO-66-X, ZnIn2S4 possesses a small surface area of 81 m2 g−1. When ZnIn2S4 integrates with UiO-66-X, the surface areas could achieve noticeable magnifications to 352, 448, 361 and 178 m2 g−1 for Z-U, Z-UOH, Z-UN and Z-U(OH)2, respectively (Fig. 2f).
The effect of UiO-66 functionalization on the microstructure was observed by SEM. First of all, ZnIn2S4 is a distinct layer structure while it has a serious aggregation (Fig. 3a).35 Under the same synthetic conditions except for the organic ligand categories, UiO-66-NH2 (Fig. 3b), UiO-66-OH (Fig. 3c) and UiO-66 (Fig. 3d) present regular and uniform octahedrons, while UiO-66-(OH)2 has a tiny nanoparticle morphology caused by the low crystallinity (Fig. 3e).24 In addition, the particle sizes follow a sequence of UiO-66-(OH)2 (∼40 nm) < UiO-66-NH2 (∼450 nm) < UiO-66-OH (∼650 nm) < UiO-66 (∼950 nm), which probably induced by the steric-hindrance effect of functional groups during the crystal nucleus growth. As to Z-UN (Fig. 3f), Z-UOH (Fig. 3g) and Z-U (Fig. 3h), the corresponding UiO-66-NH2, UiO-66-OH and UiO-66 octahedrons were evenly wrapped by ZnIn2S4 nanosheets. Conversely, for Z-U(OH)2, UiO-66-(OH)2 nanoparticles were loaded on ZnIn2S4 layers (Fig. 3i). Besides, the growth of ZnIn2S4 nanosheets on UiO-66-NH2 could be tuned (Fig. S10†) and the morphology of Z-UN relatively remained unchanged after the photocatalytic H2O2 generation (Fig. S11†).
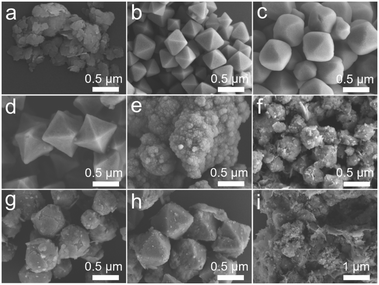 |
| Fig. 3 SEM images of ZnIn2S4 (a) UiO-66-NH2 (b), UiO-66-OH (c), UiO-66 (d), UiO-66-(OH)2 (e), Z-UN (f), Z-UOH (g), Z-U (h) and Z-U(OH)2 (i). | |
To further characterize the microstructure of Z-UN, TEM images of UiO-66-NH2, ZnIn2S4 and Z-UN were conducted. The regular octahedron of UiO-66-NH2 (Fig. 4a) and ultra-thin layers of ZnIn2S4 (Fig. 4b) could be indubitably reflected. What's more, their spatial distribution and interface contact in Z-UN can be clearly observed (Fig. 4c and d). The lattice fringe of 0.295 nm corresponding to the ZnIn2S4 (104) crystal plane in high-resolution TEM of Z-UN further validates the outer layer of ZnIn2S4 (Fig. 4d). Likewise, the (110), (104) and (102) crystal planes of ZnIn2S4 could be also indicated by the diffraction fringes of selected area electron diffraction (SAED) patterns (Fig. 4e). EDS mapping images of Z-UN uncover the uniform distribution of In, S, Zn, Zr, C, O and N elements (Fig. 4f–l), and such results indicate the formation of homogeneous interfacial junctions between ZnIn2S4 nanosheets and UiO-66-NH2 octahedrons.
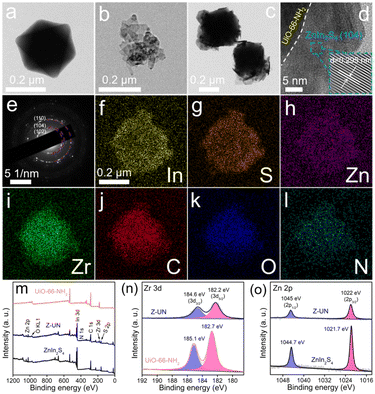 |
| Fig. 4 TEM images of UiO-66-NH2 (a), ZnIn2S4 (b) and Z-UN (c and d), SAED pattern (e) and EDS mapping images (f–l) of Z-UN, XPS spectra of UiO-66-NH2, Z-UN and ZnIn2S4: survey (m), Zr 3d (n) and Zn 2p (o). | |
The elemental composition of In, S, Zn, Zr, C, O and N for Z-UN was further reflected by the XPS survey spectrum (Fig. 4m). The Zr 3d curve of UiO-66-NH2 can be split into two peaks at 182.7 and 185.1 eV (Fig. 4n), which are assigned to Zr 3d5/2 and 3d3/2, respectively. Nevertheless, the two related peaks of Z-UN underwent negative shifts to 182.2 and 184.6 eV, implying a net gain of electrons for Zr4+. In contrast to the peak shifts of Zr4+, peaks of Zn 2p3/2 and 2p1/2 experienced high-frequency shifts from 1021.7 and 1044.7 eV of ZnIn2S4 to 1022 and 1045 eV of Z-UN, respectively (Fig. 4o), suggesting an electron loss of Zn2+. Based on the gleaned above results, it is inferred that the electrons moved from ZnIn2S4 to UiO-66-NH2 with their integration, which demonstrates the successful construction of an internal electric field between ZnIn2S4 and UiO-66-NH2.
3.3 Mechanism discussion
The functionalization of MOFs has a huge effect on their light absorption because the functional groups could serve as auxochromic and bathochromic groups in aromatic rings.24,25 To determine this, UV-vis DRS of UiO-66-X were performed first (Fig. 5a). UiO-66 has the smallest light absorption mainly in 200–320 nm that is aroused by the ligand-to-metal charge transfer, suggesting the bonding between carboxylate oxygen and metal.36 By comparison, the light absorption edges of all functionalized UiO-66 underwent redshifts, especially for UiO-66-NH2 and UiO-66-(OH)2 shifting to the visible-light range, which is initiated by the conjugated π electron transition from the amino or hydroxyl-auxochromic chromophores to the Zr centers.37 Correspondingly, the bandgaps of UiO-66, UiO-66-OH, UiO-66-(OH)2 and UiO-66-NH2 are 3.91, 3.33, 2.79 and 2.93 eV, respectively, according to the curves of (αhv)2versus hv (Fig. 5b). It should be stressed that ZnIn2S4 has an outstanding visible-light absorption with a narrow bandgap of 2.5 eV, and all ZnIn2S4/UiO-66-X heterostructures exhibit striking visible-light absorption with edges about 500 nm similar to that of ZnIn2S4 (Fig. 5c and S12†).
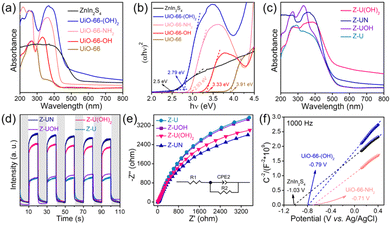 |
| Fig. 5 UV-vis DRS (a) and (αhv)2versus hv curves (b) of ZnIn2S4, UiO-66-(OH)2, UiO-66-NH2, UiO-66-OH and UiO-66, UV-vis DRS (c), photocurrent spectra (d) and EIS Nyquist plots (e) of Z-UN, Z-U(OH)2, Z-UOH and Z-U, Mott–Schottky plots of ZnIn2S4, UiO-66-(OH)2 and UiO-66-NH2 (f). | |
With the aim to validate the charge separation and transfer over ZnIn2S4/UiO-66-X heterostructures, the photocurrent and EIS were tested. At first, in comparison with ZnIn2S4 and UiO-66-NH2, Z-UN performed with a stronger photocurrent signal and smaller arc radius of the EIS Nyquist plot (Fig. S13 and S14†), which signifies the reinforced separation of photo-excited charge carriers and diminished resistance of charge transfer. In the case of ZnIn2S4/UiO-66-X heterostructures, Z-UN and Z-U(OH)2 possess more efficient charge separation and lower resistance of charge transportation than those of Z-UOH and Z-U counterparts (Fig. 5d and e), which accord well with the corresponding results of photocatalytic H2O2 generation. The charge transfer routes meet the thermodynamic requirements, thus the band configurations of ZnIn2S4, UiO-66-(OH)2 and UiO-66-NH2 are indispensable to be uncovered. To this end, Mott–Schottky plots of ZnIn2S4, UiO-66-(OH)2 and UiO-66-NH2 were monitored at 1000 and 2000 Hz (Fig. 5f and S15†). The flat-band potentials are −1.03, −0.79 and −0.71 V vs. Ag/AgCl (−0.833, −0.593, −0.513 V vs. NHE, ENHE = EAg/AgCl + 0.197 V) for ZnIn2S4, UiO-66-(OH)2 and UiO-66-NH2, respectively. It is regarded that the conduction band (CB) or lowest unoccupied molecular orbital (LUMO) potentials for n-type semiconductors are more negative by 0.1 V than their flat-band potentials.38 As such, the CB potential of ZnIn2S4, LUMO potentials of UiO-66-(OH)2 and UiO-66-NH2 are about −0.93, −0.69 and −0.61 eV, corresponding to the VB or highest occupied molecular orbital (HOMO) potentials of 1.57, 2.1 and 2.32 eV, respectively (EVB or HOMO = EBandgap + ECB or LUMO).
Although the related band configurations were known, the routes of photocatalytic H2O2 generation must be confirmed before elucidating the whole mechanism. Generally, it is deemed that the photocatalytic O2 reduction into H2O2 experiences a direct (O2 + 2H+ + 2e− → H2O2) or indirect (O2 + e− → ˙O2−, ˙O2− + e− + 2H+ → H2O2) way.2,20 To unveil this, the generation of ˙O2− under visible-light illumination using Z-UN was first evidenced by EPR (Fig. 6a). Subsequently, p-benzoquinone was employed as a scavenger of ˙O2− in the H2O2 evolution process using Z-UN. As illustrated in Fig. 6b, it is noticeable that the H2O2 yield has a stark decrease in the presence of p-benzoquinone, affirming that ˙O2− serves as a significant intermediate species for H2O2 generation. Likewise, the same trend also happened on Z-U(OH)2 (Fig. S16†). These results collectively suggest that photocatalytic H2O2 generation using Z-UN and Z-U(OH)2 underwent an indirect O2 reduction reaction.
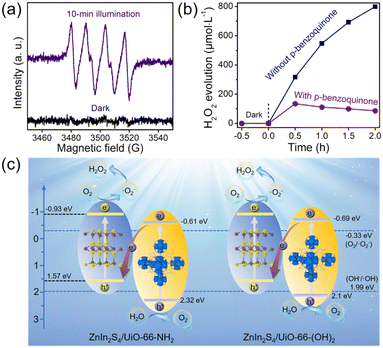 |
| Fig. 6 EPR spectra of DMPO–˙O2− adducts using Z-UN at dark and 10 min illumination (a), photocatalytic H2O2 evolution using Z-UN with and without p-benzoquinone (b), the proposed mechanism for photocatalytic H2O2 evolution using Z-UN and Z-U(OH)2 (c). | |
At last, the proposed mechanism for photocatalytic H2O2 evolution using ZnIn2S4/UiO-66-X was discussed based on the above analyses. By means of the narrow bandgaps, ZnIn2S4 (2.5 eV), UiO-66-NH2 (2.93 eV) and UiO-66-(OH)2 (2.79 eV) are capable to be excited to generate electrons and holes by visible-light illumination (Fig. 6c). At this time, according to the band configuration of each monomer, the transfer routes of charge carriers should be determined using Z-UN and Z-U(OH)2, namely, conforming to type-II or Z-scheme heterostructure. To this end, ˙OH generation was tested since the corresponding potential (E(OH−/˙OH) = 1.99 eV (ref. 39)) is in between the VB potential of ZnIn2S4 (1.57 eV) and HOMO potential of UiO-66-NH2 (2.32 eV) or UiO-66-(OH)2 (2.1 eV). As a consequence, ˙OH generation was demonstrated by EPR spectra using Z-UN (Fig. S17†) and Z-U(OH)2 (Fig. S18†), suggesting that the holes on the HOMO of UiO-66-NH2 and UiO-66-(OH)2 were kept. Thereby, Z-scheme heterostructures were formed in Z-UN and Z-U(OH)2. While for Z-U and Z-UOH, the electrons would transfer from the CB of ZnIn2S4 to LUMO of UiO-66 or UiO-66-OH (Fig. S19†) because of no generation of electrons and holes by UiO-66 and UiO-66-OH. Ultimately, electrons on the CB of ZnIn2S4 in Z-UN and Z-U(OH)2 possess sufficient abilities to reduce O2 into ˙O2− (E(O2/˙O2−) = −0.33 eV) and further evolve into H2O2. Appreciably, the conspicuous visible-light absorption and unique Z-scheme heterostructures decide the superiority of Z-UN and Z-U(OH)2 on photocatalytic H2O2 evolution in comparison with those of Z-U and Z-UOH. That is to say, the functionalization of UiO-66 does enable the efficient photocatalytic generation of H2O2.
4. Conclusions
To sum up, various ZnIn2S4/UiO-66-X heterostructures were fabricated for photocatalytic H2O2 generation under visible light in pure water. The H2O2 yields using ZnIn2S4/UiO-66-NH2, ZnIn2S4/UiO-66-(OH)2 and ZnIn2S4/UiO-66-OH are 799, 733 and 165 μmol L−1, respectively, while the corresponding yield using ZnIn2S4/UiO-66 is only 84 μmol L−1. The outstanding performances by using ZnIn2S4/UiO-66-NH2 and ZnIn2S4/UiO-66-(OH)2 are attributed to the favorable visible-light absorption and Z-scheme heterostructures. Furthermore, it is concluded that the H2O2 generation using ZnIn2S4/UiO-66-NH2 and ZnIn2S4/UiO-66-(OH)2 followed indirect O2 reduction with ˙O2− as the intermediate species. This study could provide new insight into MOF functionalization and a new doorway to green energy conversion by (photo)catalysis.
Author contributions
JQ: conceptualization, investigation, funding acquisition, and writing – original draft; LZ: data curation, formal analysis, investigation, and writing – original draft; GX, DD, YT: investigation; JY: conceptualization, resources, funding acquisition, and writing – review & editing.
Conflicts of interest
There are no conflicts of interest.
Acknowledgements
The authors are grateful for the financial supports of the Natural Science Foundation of Jiangsu Province Youth Fund (BK20210628) and the Scientific Research Foundation from Nanjing Forestry University. We also thank the Advanced Analysis & Testing Center, Nanjing Forestry University for sample tests. JQ and LZ contributed equally to this work.
References
- Y. Xue, Y. Wang, Z. Pan and K. Sayama, Angew. Chem., Int. Ed., 2021, 60, 10469–10480 CrossRef CAS PubMed.
- A. Gopakumar, P. Ren, J. Chen, B. V. Manzolli Rodrigues, H. Y. Vincent Ching, A. Jaworski, S. V. Doorslaer, A. Rokicińska, P. Kuśtrowski, G. Barcaro, S. Monti, A. Slabon and S. Das, J. Am. Chem. Soc., 2022, 144, 2603–2613 CrossRef CAS PubMed.
- T. Liu, Z. Pan, J. J. M. Vequizo, K. Kato, B. Wu, A. Yamakata, K. Katayama, B. Chen, C. Chu and K. Domen, Nat. Commun., 2022, 13, 1034 CrossRef CAS PubMed.
- J. Hu, T. Yang, J. Chen, X. Yang, J. Qu and Y. Cai, Chem. Eng. J., 2022, 430, 133039 CrossRef CAS.
- X. Chen, Y. Kondo, S. Li, Y. Kuwahara, K. Mori, D. Zhang, C. Louis and H. Yamashita, J. Mater. Chem. A, 2021, 9, 26371–26380 RSC.
- X. Zeng, Y. Liu, X. Hu and X. Zhang, Green Chem., 2021, 23, 1466–1494 RSC.
- H.-I. Kim, O. S. Kwon, S. Kim, W. Choi and J.-H. Kim, Energy Environ. Sci., 2016, 9, 1063–1073 RSC.
- L. Chen, C. Chen, Z. Yang, S. Li, C. Chu and B. Chen, Adv. Funct. Mater., 2021, 31, 2105731 CrossRef CAS.
- C. Chen, G. Qiu, T. Wang, Z. Zheng, M. Huang and B. Li, J. Colloid Interface Sci., 2021, 592, 1–12 CrossRef CAS PubMed.
- J. Qiu, X. Zhang, Y. Feng, X. Zhang, H. Wang and J. Yao, Appl. Catal., B, 2018, 231, 317–342 CrossRef CAS.
- A. S. Belousov and E. V. Suleimanov, Green Chem., 2021, 23, 6172–6204 RSC.
- J. Qiu, X.-F. Zhang, X. Zhang, Y. Feng, Y. Li, L. Yang, H. Lu and J. Yao, J. Hazard. Mater., 2018, 349, 234–241 CrossRef CAS PubMed.
- L.-Y. Wu, Y.-F. Mu, X.-X. Guo, W. Zhang, Z.-M. Zhang, M. Zhang and T.-B. Lu, Angew. Chem., Int. Ed., 2019, 58, 9491–9495 CrossRef CAS PubMed.
- H. Huang, X.-S. Wang, D. Philo, F. Ichihara, H. Song, Y. Li, D. Li, T. Qiu, S. Wang and J. Ye, Appl. Catal., B, 2020, 267, 118686 CrossRef CAS.
- Y. Kondo, Y. Kuwahara, K. Mori and H. Yamashita, Chem, 2022, 8, 2924–2938 CAS.
- Y. Isaka, Y. Kawase, Y. Kuwahara, K. Mori and H. Yamashita, Angew. Chem., Int. Ed., 2019, 58, 5402–5406 CrossRef CAS PubMed.
- Y. Kawase, Y. Isaka, Y. Kuwahara, K. Mori and H. Yamashita, Chem. Commun., 2019, 55, 6743–6746 RSC.
- Y. Wu, X. Li, Q. Yang, D. Wang, F. Yao, J. Cao, Z. Chen, X. Huang, Y. Yang and X. Li, Chem. Eng. J., 2020, 390, 124519 CrossRef CAS.
- Y. Zhao, Y. Liu, J. Cao, H. Wang, M. Shao, H. Huang, Y. Liu and Z. Kang, Appl. Catal., B, 2020, 278, 119289 CrossRef CAS.
- C. Liu, T. Bao, L. Yuan, C. Zhang, J. Wang, J. Wan and C. Yu, Adv. Funct. Mater., 2022, 32, 2111404 CrossRef CAS.
- H. Jasuja, G. W. Peterson, J. B. Decoste, M. A. Browe and K. S. Walton, Chem. Eng. Sci., 2015, 124, 118–124 CrossRef CAS.
- A. Jrad, M. Hmadeh, G. Awada, R. Chakleh and M. Ahmad, Chem. Eng. J., 2021, 410, 128237 CrossRef CAS.
- D. Chen, W. Yang, L. Jiao, L. Li, S.-H. Yu and H.-L. Jiang, Adv. Mater., 2020, 32, 2000041 CrossRef CAS PubMed.
- Y. L. Wang, S. Zhang, Y. F. Zhao, J. Bedia, J. J. Rodriguez and C. Belver, J. Environ. Eng., 2021, 9, 106087 CAS.
- H. Liu, M. Cheng, Y. Liu, G. Zhang, L. Li, L. Du, B. Li, S. Xiao, G. Wang and X. Yang, Coord. Chem. Rev., 2022, 458, 214428 CrossRef CAS.
- J. Qiu, Y. Feng, X. Zhang, M. Jia and J. Yao, J. Colloid Interface Sci., 2017, 499, 151–158 CrossRef CAS PubMed.
- J. Qiu, L. Zhang, D. Dai, G. Xia and J. Yao, ChemSusChem, 2022, 15, e202200399 CAS.
- H. Su, C. Rao, L. Zhou, Y. Pang, H. Lou, D. Yang and X. Qiu, Green Chem., 2022, 24, 2027–2035 RSC.
- Y. Qin, H. Li, J. Lu, Y. Feng, F. Meng, C. Ma, Y. Yan and M. Meng, Appl. Catal., B, 2020, 277, 119254 CrossRef CAS.
- S. Li, S. Sun, H. Wu, C. Wei and Y. Hu, Catal. Sci. Technol., 2018, 8, 1696–1703 RSC.
- Y. Sun, M. Chen, H. Liu, Y. Zhu, D. Wang and M. Yan, Appl. Surf. Sci., 2020, 525, 146614 CrossRef CAS.
- G. W. Peterson, J. J. Mahle, J. B. DeCoste, W. O. Gordon and J. A. Rossin, Angew. Chem., 2016, 128, 6343–6346 CrossRef.
- J. Liu, X. Huang, L. Liu, Q. Nie, Z. Tan and H. Yu, J. Environ. Eng., 2022, 10, 108294 CAS.
- Y. Li, F. Ma, L. Zheng, Y. Liu, Z. Wang, P. Wang, Z. Zheng, H. Cheng, Y. Dai and B. Huang, Mater. Horiz., 2021, 8, 2842–2850 RSC.
- C. Du, Q. Zhang, Z. Lin, B. Yan, C. Xia and G. Yang, Appl. Catal., B, 2019, 248, 193–201 CrossRef CAS.
- R. W. Liang, F. F. Jing, L. J. Shen, N. Qin and L. Wu, J. Hazard. Mater., 2015, 287, 364–372 CrossRef CAS PubMed.
- X. Mu, J. Jiang, F. Chao, Y. Lou and J. Chen, Dalton Trans., 2018, 47, 1895–1902 RSC.
- J. Qiu, D. Dai, L. Zhang, G. Xia and J. Yao, Sep. Purif. Technol., 2022, 301, 121990 CrossRef CAS.
- J. J. Shi, S. D. Li, F. M. Wang, Y. M. Li, L. N. Gao, X. R. Zhang and J. Lu, Catal. Sci. Technol., 2018, 8, 6458–6467 RSC.
|
This journal is © The Royal Society of Chemistry 2023 |