DOI:
10.1039/D3BM00881A
(Paper)
Biomater. Sci., 2023,
11, 5872-5892
Engineering a platelet-rich plasma-based multifunctional injectable hydrogel with photothermal, antibacterial, and antioxidant properties for skin regeneration†
Received
21st May 2023
, Accepted 5th July 2023
First published on 11th July 2023
Abstract
Wound healing remains a significant challenge worldwide, necessitating the development of new wound dressings to aid in the healing process. This study presents a novel photothermally active hydrogel that contains platelet-rich plasma (PRP) for infected wound healing. The hydrogel was formed in a one pot synthesis approach by mixing alginate (Alg), gelatin (GT), polydopamine (PDA), and PRP, followed by the addition of CaCl2 as a cross-linker to prepare a multifunctional hydrogel (AGC–PRP–PDA). The hydrogel exhibited improved strength and good swelling properties. PDA nanoparticles (NPs) within the hydrogel endowed them with high photothermal properties and excellent antibacterial and antioxidant activities. Moreover, the hydrogels sustained the release of growth factors due to their ability to protect PRP. The hydrogels also exhibited good hemocompatibility and cytocompatibility, as well as high hemostatic properties. In animal experiments, the injectable hydrogels effectively filled irregular wounds and promoted infected wound healing by accelerating re-epithelialization, facilitating collagen deposition, and enhancing angiogenesis. The study also indicated that near-infrared light improved the healing process. Overall, these hydrogels with antibacterial, antioxidant, and hemostatic properties, as well as sustained growth factor release, show significant potential for skin regeneration in full-thickness, bacteria-infected wounds.
1. Introduction
Wound healing is a significant global challenge, and the development of suitable wound dressings that promote all stages of the healing process, including hemostasis, inflammation, proliferation, and remodeling, is urgently needed.1–4 Hydrogels are a promising type of wound dressing material for skin repair because they can absorb exudate and create a moist environment for wound regeneration.5,6 Injectable hydrogels, in particular, can fill any irregular wound shape, providing a physical barrier that shields the wound area from bacterial infection quickly.7–9 Numerous natural polymer-based hydrogels, such as chitosan, hyaluronic acid, and alginate, have been used as wound dressings to promote skin regeneration.10,11 Alginate, a natural polysaccharide, can form biocompatible hydrogels through ionic cross-linking with multivalent cations (e.g., Ca2+, Sr2+, and Ba2+).12,13 Gelatin, a commonly used natural macromolecule in biological sciences, is known for its excellent non-immunogenicity, biocompatibility, and biodegradability. Gelatin-based hydrogels have been extensively employed in designing and constructing injectable hydrogel dressings due to their similarity to the extracellular matrix (ECM), attractive fluid absorption properties, and promotion of cell adhesion and proliferation.14 Recently, metal cross-linked hydrogels using Ca2+,15 Fe3+,16 Cu2+,17 Zn2+,18 and Ag+
19 have garnered increasing attention in the design and fabrication of multifunctional hydrogels. The metal ions not only acted as cross-linkers to produce hydrogels with high mechanical performances, but also the cations endowed the hydrogels with high healing properties. Bacterial infection can impede the healing process of an infected wound, resulting in serious tissue damage and even life-threatening complications. Photothermal therapy (PTT) is a promising strategy that combines near-infrared light (NIR) (700–1100 nm) and light-absorbing materials to kill bacteria by inducing local hyperthermia during the PTT process.20 PDA NPs have emerged as an effective photothermal agent in PTT of infected wounds due to their facile synthesis, long-term safety, high biodegradability, superior biocompatibility, and excellent photostability.21,22 The unique properties of PDA endow NIR-responsive hydrogels with high cell/tissue adhesive, intrinsic antioxidant, and antibacterial properties.23,24 Hemostasis, inflammation, proliferation, and dermal remodeling are four consecutive and overlapping steps involved in the wound healing process.8 Additionally, many growth factors, chemokines, and cytokines play an essential role in this complex process.25 Recent studies have shown that platelet-rich plasma (PRP), containing a high concentration of platelet-oriented plasma, has the potential to improve wound healing. PRP contains numerous growth factors, including vascular endothelial growth factor (VEGF), platelet-derived growth factor, and transforming growth factor-β (TGF-β), which significantly contribute to regenerative medicine and wound repair.26,27 These factors interact with the local environment to accelerate cell proliferation and differentiation, thus promoting angiogenesis and re-epithelialization through extracellular matrix synthesis and mesenchymal cell recruitment.28 However, the application of PRP in wound healing is limited due to its short half-life, burst release, and high cost of synthesis.29,30 Therefore, addressing how to reduce burst release, retain activity, and design a cost-effective method is crucial when using PRP-based tissue engineering. In this study, an injectable, photothermally PRP-loaded hydrogel is developed for infected dermal wound healing. The hydrogels were constructed based on alginate and gelatin cross-linked by Ca2+ ions (abbreviated as AGC) and PDA as a photothermal agent. After blood collection and centrifugation at different rates to fractionate the blood, the PRP fraction was isolated as the yellowish layer of plasma (Fig. 1). To prepare the AGC–PRP–PDA hydrogel, alginate, gelatin, PDA NPs, and PRP were mixed to produce a homogeneous polymeric solution. Subsequently, a mixture of thromboplastin (TPL) and CaCl2 was added to the previous mixture to form the multifunctional hydrogel (Fig. 1). CaCl2 not only acts as a cross-linker to induce gelation, but also activates PRP from a dynamic liquid to a gel, containing a large number of growth factors. We also used TPL in the fabrication of the hydrogels, resulting in a more homogeneous mixture. TPL can accelerate blood coagulation through the conversion of prothrombin to thrombin.31,32 In addition, we also prepared a platelet poor plasma (PPP)-containing hydrogel (AGC–PPP–PDA) to compare its therapeutic effect with AGC–PRP–PDA.
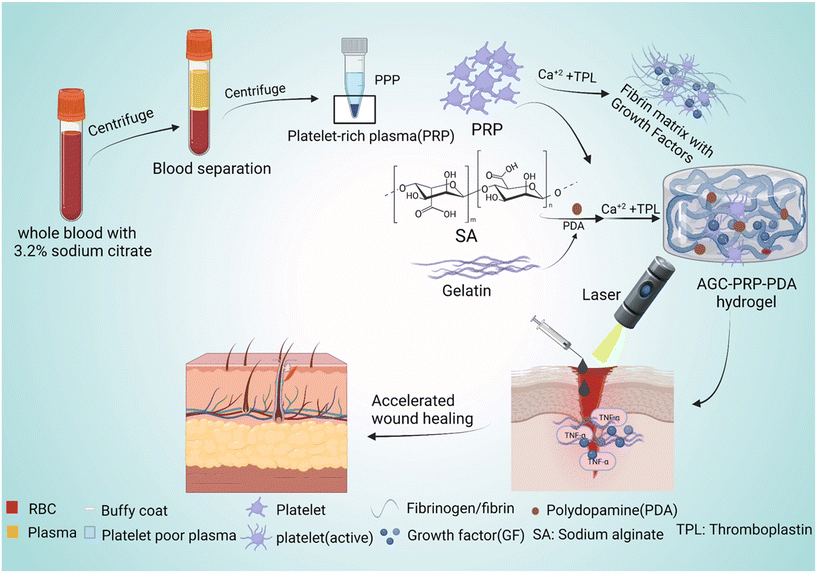 |
| Fig. 1 Schematic representation of the preparation of PRP and PPP as well as the synthetic route of the AGC–PRP–PDA hydrogel with multiple functionalities to promote wound regeneration. | |
2. Materials and methods
2.1 Materials
Gelatin (15% (w/v), Fig. S4B,† type B, from bovine skin), sodium alginate (2.5% (w/v), Fig. S4C†), dopamine hydrochloride, Dulbecco's Modified Eagle's Medium (DMEM), fetal bovine serum (FBS), penicillin/streptomycin and 3-[4,5-dimethylthiazole-2-yl]-2,5-diphenyltetrazolium bromide (MTT) were purchased from Sigma-Aldrich. NIH3T3 cells were obtained from the Iranian Biological Resource Center (ATCC, CRL-1658). Calcium chloride (Merck, Germany) and the other reagents were analytically pure and used as received.
2.2 Preparation of PRP and PPP
PRP and PPP were obtained from Sprague-Dawley rat's blood in consensus with the guidelines of Zanjan University of Medical Sciences, Zanjan, Iran (ethical code: IR.ZUMS.REC.1400.071). PRP and PPP were collected from the rat's peripheral blood using the double-spin method.33 Briefly, the rat's peripheral blood was collected in tubes containing an anticoagulant. Then, the two consecutive stages of centrifugation were carried out following particular parameters (first, 1600 rpm for 12 min and second, 2500 rpm for 5 min at room temperature) to optimize the production of PRP and PPP. Finally, 2/3 of supernatant was collected as PPP, and the remaining plasma and platelets were mixed and considered as PRP.
2.3 Preparation of PRP and AGC hydrogels
PRP gel was prepared by the activation of PRP by CaCl2 (100 μL, 2% w/v) and TPL (100 μL, 22.08 mg mL−1). To fabricate the AGC hydrogel, 200 μL of sodium alginate (2.5% w/v) and 100 μL of gelatin (15% w/v) were firstly mixed to make a homogeneous mixture. Then, 50 μL of Ca2+ solution (2% w/v) was added into the previous mixture to obtain the AGC hydrogel.
2.4 Preparation of AGC–PRP–PDA and AGC–PPP–PDA hydrogels
For fabrication of the AGC–PRP–PDA hydrogel, 400 μL of sodium alginate solution (2.5% w/v), 200 μL of gelatin solution (15% w/v), 200 μL of PRP solution, and 10 μL of PDA NPs (5 mg mL−1) were firstly mixed to make a homogeneous solution. Then, a pre-prepared mixture of 200 μL of Ca2+ solution (2% w/v) and 100 μL of TPL (22.08 mg mL−1) was added to the above mixture to form the AGC–PRP–PDA hydrogel. The AGC–PPP–PDA hydrogel was prepared using the same procedure with the difference that PPP was used instead of PRP.
2.5 Synthesis of PDA NPs
PDA NPs with an average diameter of 170–180 nm were synthesized according to a previous report.34 Briefly, ammonia aqueous solution (1 mL, 28–30%) was added into a mixture of ethanol (20 mL) and deionized water (45 mL) under mild stirring at 30 °C for 30 min. Then, dopamine hydrochloride (0.25 g), dissolved in deionized water (5 mL), was added dropwise into the previous solution. The color of the resulting solution turned pale yellow and gradually changed to dark brown. After 24 h, the final product was obtained by centrifugation and was washed with deionized water three times and then dispersed in deionized water.
2.6 Characterization of the PDA NPs and the hydrogels
The microstructure and morphology of the hydrogels were determined by scanning electron microscopy (SEM) (Quanta 250 FEG, USA).
The formation of the hydrogels was confirmed by Fourier transform infrared spectroscopy (FTIR) (Bruker, Tensor 27, Germany). The UV-vis-NIR analysis of PDA NPs and the AGC–PRP–PDA hydrogel was performed using a UV-vis spectrophotometer (Bruker IFS 66v/s). The zeta potential of PDA NPs was measured by dynamic light scattering (DLS) (Malvern, UK).
2.7 Swelling and in vitro degradation of the AGC–PRP–PDA hydrogel
A weighted cylindrical freeze-dried hydrogel (S0) was incubated in PBS solution (pH ∼ 7.4) at room temperature. At predetermined intervals, the hydrogel was carefully taken out, and the residual water on the surface was removed using filter paper and weighed (S1). The swelling percentage was measured using the following formula: | Swelling percentage = S1/S0 × 100, | (1) |
where S0 represents the initial weight of the hydrogel at time 0 h and S1 is the weight of the hydrogel at different times.35
An in vitro gel degradation assay was carried out based on a previous report.35,36 Briefly, the cylindrical freeze-dried hydrogels were weighed (W0) and incubated in PBS solution (pH ∼ 7.4). At predetermined intervals, the residual hydrogels were carefully taken out from the solution, and freeze-dried and weighed (Wt). The percentage of the remaining weight was measured using the following equation:
| Weight remaining (%) = Wt/W0 × 100, | (2) |
where
W0 and
Wt are the weights of the AGC–PRP–PDA hydrogel before and after degradation, respectively.
2.8 Molecular docking
The structure of the alginate fragment was constructed using the block co-polymer builder of Materials Studio software. The model consisted of 2 and 3 consecutive units of α-L-guluronic acid (G) and β-D-mannuronic acid (M), respectively (GGMMM). This alginate sequence contains all three types of G–G, M–M and G–M bonds. For the gelatin material, with the help of an in-house script, a portion of the collagen type I alpha 1 sequence (UniProt ID: Q6LAN8) was carefully chosen in such a way that it represents the common sequence motifs and amino acid composition of collagen proteins as closely as possible. The initial 3D structure of the collagen fragment with the sequence of Gly–Pro–Ala–Gly–Arg–Pro–Gly–Glu–Val was predicted using the SWISS Model server. The final structure of the peptide was built by manual conversion of the structure of the second proline (position 6) to the 4-hydroxy proline. The structures of both ligands (alginate and collagen) were then geometry optimized using Avogadro software. The molecular structures of the growth factors VEGF-A (PDB ID: 5T89) and TGF-β (PDB ID: 1KLC) were obtained from the Protein Data Bank (PDB). Docking input files were prepared using AutoDock tools (ADT) version 1.5.6. The sizes of the grid boxes were adjusted to include the entire structure of the target molecules. The docking simulations were performed using AutoDock version 4.2.6. The Lamarckian genetic algorithm with default search parameters, with the exception of a larger number of runs (n = 500), was used to predict possible hydrogel–growth factor interaction sites and binding energies. Visualization and binding site analysis of the top-ranked poses were performed using PyMol software.
2.9 Rheological measurements of the hydrogels
The viscoelastic properties of the hydrogels were determined by a frequency sweep test (MCR300, Anton Paar, USA), at the frequency of 0.01–100 Hz to obtain the storage modulus (G′) and loss modulus (G′′). The viscosity of AGC, AGC–PPP–PDA, and AGC–PRP–PDA hydrogels against the shear rate was determined with parallel plate geometry (75 mm diameter) at room temperature using a rheometer (R/S Brookfield, Canada) to confirm shear thinning properties.
2.10 Photothermal evaluation of the hydrogel
The photothermal performance of the AGC–PRP–PDA hydrogel and PDA NPs with different concentrations was investigated using an 808 nm NIR laser at power densities of 0.5, 1, and 1.5 W cm−2 for 10 min. The temperature was recorded using an infrared thermal camera (TiS55, Fluke, USA). The photothermal stability of the AGC–PRP–PDA hydrogel was measured by five cycles of laser on–off. To this end, 1 mL of the hydrogel was irradiated under 808 nm (1 W cm−2) for 10 min (laser on for 10 min per cycle). The temperature was recorded every minute using the infrared thermal camera. The photothermal conversion efficiency (η) of the AGC–PRP–PDA hydrogel was calculated using the following equation:7,37,38 | 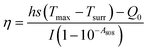 | (3) |
Hence, first, the time constant of heat transfer (τs) was calculated by linear time data versus −ln(θ) from the cooling phase, as follows: |  | (4) |
The θ value was measured using the equation:
|  | (5) |
where
T refers to the temperature in each time point.
Then, (hs) was measured using the following equation:
|  | (6) |
After that, deionized water (solvent) (1 mL) was irradiated using an 808 nm laser for 10 min and the temperature was recorded in the cooling phase and all the above-mentioned processes were repeated for the solvent. Q0 is the heat associated with the light absorbance of the solvent. It was calculated using the following equation:
| Q0 = hs(Tmax, water − Tsurr) | (7) |
Tmax, water −
Tsurr was measured between the maximum temperature of deionized water and the surrounding temperature. Finally, the
η value was obtained, where
h is the heat transfer coefficient,
s is the surface area of the container,
Q0 is the heat associated with the light absorbance of the solvent,
mi is the weight of the dried hydrogel, and
ci is the heat capacity of the solvent, which is 4.2 J g
−1 K
−1.
A808 is the absorbance of PDA NPs at the wavelength of 808 nm.
2.11
In vitro antibacterial activity of the hydrogels
Staphylococcus aureus (S. aureus, ATCC25923) and Escherichia coli (E. coli, ATCC25922) were used to evaluate the antibacterial activity of the hydrogels. Bacteria were cultured in a Brain Heart Infusion (BHI) broth/agar (Merck, Germany) aerobically at 37 °C for 18–24 h. The antibacterial effect of the hydrogels was evaluated by the spread plate method for colony counting of E. coli and S. aureus. A volume of the as-prepared bacterial suspension (20 μL, 1.5 × 108 CFU mL−1) was added into 1 mL of all the hydrogel groups and normal saline as the control group. The NIR-treated groups were exposed to 808 nm light for 10 min (1 W cm−2). After incubation for 2 h, the bacterial solution was further re-suspended and diluted 30 times. Then, 100 μL of the diluted bacterial solution was spread on nutrient agar (Merck, Germany) and incubated for 18–24 h. Bacterial colonies were counted in each sample and the bacterial survival ratio was calculated as follows:38,39 | Bacterial survival ratio% = (N in the sample/N in the control) × 100, | (8) |
where N is the colony number on a plate.
2.12
In vitro antibacterial activity of PDA NPs
The spread plate method was carried out to investigate the antibacterial effect of PDA NPs. 20 μL of the as-prepared E. coli or S. aureus suspension (1.5 × 108 CFU mL−1) was added into 1 mL of LB broth containing 5 mg mL−1 PDA NPs. 1 mL of LB broth containing the same amount of the bacterial suspension was considered as the control group. The NIR-treated groups were exposed to 808 nm laser irradiation for 10 min (1 W cm−2). After incubation for 2 h, the bacterial solution was diluted using normal saline, and subsequently, 100 μL of the diluted solution was spread on the agar plate and incubated at 37 °C for 18–24 h. Finally, bacterial colonies were counted in each plate. At least two samples on each group were tested and the bacterial survival ratio% was expressed as: | Bacterial survival ratio% = (N in the sample/N in the control) × 100, | (9) |
where N is the colony number on a plate.40,41
2.13 Antioxidant capacity of the hydrogels
The efficiency of the free radical scavenging performance of the AGC, AGC–PPP–PDA, and AGC–PRP–PDA hydrogels was studied using 1,1-diphenyl-2-picryl-hydrazyl (DPPH) as an indicator, which is commonly used to assess the reactive nitrogen species (RNS) scavenging capacity of the materials. First, 500 μM DPPH and the desired amount of the AGC–PRP–PDA hydrogel (1, 2, 3, and 4 mg) were dispersed in 3 mL of ethanol. Then, the mixture was stirred in a dark place at room temperature for half an hour. Finally, the absorbance change of DPPH was measured using a UV-vis spectrophotometer. DPPH scavenging activity was studied using the following equation:42 | DPPH scavenging (%) = (Ab − As)/Ab × 100, | (10) |
where Ab is the absorption of the blank (DPPH + ethanol) and As is the absorption of the sample (DPPH + sample + ethanol). A H2O2 scavenging assay was performed using a previously reported study.43,44 6 mg mL−1 and 10 mg mL−1 AGC–PRP–PDA gels were blended with 0.8 mL of freshly prepared 40 mM H2O2 solution. Similar concentrations of ascorbic acid were used as a positive control. The reaction blends were incubated at room temperature for 30 min, and the absorbance of the samples was measured at 230 nm using a UV-visible spectrophotometer.
2.14 Hemolysis assay
An in vitro hemolysis test was performed using an erythrocyte suspension according to previously reported protocols.45 Whole anticoagulant blood was centrifuged with PBS (pH 7.4) at 3000 rpm for 6 min to obtain fresh red blood cells (RBCs). The purified RBCs were washed five times and diluted to a concentration of 5% (v/v). Afterward, 500 μL of PBS solution (pH 7.4) containing different amounts of hydrogels were mixed with RBC suspensions (100 μL) to obtain the mixtures with different concentrations (0.25, 0.5, and 1 mg mL−1). PBS solution and deionized water were used as the negative and positive control groups, respectively. After incubation at 37 °C for 2, 4, 8, and 24 h, all mixtures were centrifuged at 3000 rpm for 6 min. The supernatant (150 μL) was transferred to a 96-well plate, and the absorbance at 540 nm was measured using a microplate reader. The hemolysis ratio was measured using the following equation: | Hemolysis ratio (%) = [(As − An)/(Ap − An)] × 100, | (11) |
or | Non-hemolysis ratio (%) = 1 − [(As − An)/(Ap − An)] × 100, | (12) |
where As, An, and Ap are the absorbance of a hydrogel, PBS, and deionized water, respectively.
2.15
In vitro cytotoxicity of the hydrogels
The cytotoxicity of the hydrogels was determined by an MTT assay. In order to perform the cell viability assay, mouse embryo fibroblast cells (NIH3T3) were cultured in DMEM with 10% fetal bovine serum and 1% penicillin–streptomycin solution in a CO2 incubator at 37 °C. NIH3T3 cells were seeded at a density of 2 × 106 cells per mL in 96 well plates. After 24 h, the culture medium was replaced by 200 μL of aqueous suspensions of AGC, AGC–PPP–PDA, and AGC–PRP–PDA (1 mg mL−1, 500 μg mL−1, and 250 μg mL−1). After incubation for 48 and 72 h, the medium was replaced with 180 μL of fresh medium and 20 μL of MTT (5 mg in PBS (1 mL)), followed by incubation for another 4 h to allow formation of the formazan dye. The medium was then replaced by 200 μL of dimethylsulfoxide per well to dissolve the purple formazan, and the absorbance was measured using a microplate reader at 570 nm. The cell viability ratio was calculated using the following formula: | Cell viability = As/Ac × 100, | (13) |
where As and Ac represent the absorbance of the sample and the control group, respectively.
2.16
In vitro blood clotting index
50 mg of freeze-dried AGC, AGC–PPP–PDA, and AGC–PRP–PDA hydrogels and gauze were placed into pre-heated falcon tubes at 37 °C. Sodium citrate whole blood (100 μL) was added on to the hydrogel surface, followed by addition of 12.5 μL of 0.2 M CaCl2 solution to start coagulation. The tubes were incubated in 37 °C. After 60, 120, and 240 s, 10 mL of deionized water was added to each tube. Subsequently, the samples were centrifuged at 1000 rpm for 5 min. Finally, the absorbance of the resulting hemoglobin solution was measured at 540 nm. The blood clotting index (BCI) was calculated using the following equation: | BCI (%) = As/Ar × 100, | (14) |
where As and Ar are the absorption of the sample or gauze and deionized water as references, respectively.
2.17
In vivo hemostatic performance
A mouse-tail amputation model was employed to evaluate the hemostatic ability of the hydrogels. All animal studies were approved by the animal research Zanjan University of Medical Sciences’ Animal Ethics Committee (IR.ZUMS.REC.1400.071). The rats (Sprague-Dawley rats, male, 200–250 g) were anesthetized using ketamine/xylazine (6
:
4, v/v). Then, the middle of the tail was cut with surgical bistoury. After cutting, the tail of the mouse was placed in air for 15 s to ensure normal blood loss. After that, the tail was covered with a pre-weighted freeze-dried hydrogel powder under slight pressure. The data of hemostatic time and blood loss were measured as the hemostatic performance. The group without the treatment was used as the control.46
2.18 Growth factor release study
To evaluate the release profile of VEGF and TGF-β of the hydrogels, 400 μL of the PRP gel (containing 200 μL of PRP) and ∼1100 μL of the AGC–PRP–PDA hydrogel (containing 200 μL of PRP) were placed in a 24 well plate (n = 3) with 1.5 mL of PBS (pH 7.4) as the release medium. The well plate was incubated at 37 °C (1 rpm). 150 μL of PBS was collected at a predetermined time interval, while 150 μL of fresh PBS was replaced. The collected PBS was stored at −20 °C for further tests. Finally, the concentrations of VEGF and TGF-β were measured using an ELISA kit following the manufacturer's protocol.47–49
2.19
In vitro wound healing (scratch assay)
The in vitro wound healing potential of the hydrogels was assessed using NIH3T3 fibroblast cells. To this end, NIH3T3 cells were seeded at a density of 5 × 105 cells per mL on 24 well plates to obtain a monolayer of the cells. The cells were starved using DMEM medium and 0.1% FBS with 5% penicillin/streptomycin for 24 h at 37 °C, preventing the cells from proliferating to ensure that wound closure results solely from cell migration.50 Next, an artificial wound was generated to create a scratch with a p100 pipette tip and then the debris was removed using a culture medium. 500 μL of the suspension of the AGC–PPP–PDA and AGC–PRP–PDA hydrogels (250 μg mL−1) dispersed in the fresh culture medium DMEM supplemented with 10% FBS were added into each well plate. The group without any treatment was considered as the control group. The cell migration of each group at 0 h, 10 h, and 24 h was re-photographed using a phase contrast microscope. The quantitative analysis was performed using the following formula:51 | Relative scratch area (%) = An/A0 × 100, | (15) |
where A0 represents the initial area at 0 h and An is the area at different times.
2.20
In vivo wound healing study
A full-thickness defect model was used to assess the healing efficiency of the hydrogels.52,53 In brief, all rats were randomly divided into 9 groups: control, Alfa (available in the market), AGC, AGC–PPP, AGC–PRP, AGC–PPP–PDA (−NIR), AGC–PRP–PDA (−NIR), AGC–PPP–PDA (+NIR), and AGC–PRP–PDA (+NIR). The rats (male, 180 g) were anesthetized using ketamine/xylazine (6
:
4, v/v), and then 10 mm diameter full-thickness skin round wounds were created after shaving on the dorsal area of the rats. Then, the wounds were inoculated with 20 μL of an S. aureus suspension (∼108 CFU mL−1). The rats were subjected to the following different treatments. In the NIR groups, the wounds were irradiated with a NIR laser (808 nm, 1 W cm−2) after adding the hydrogels for 5 min and maintained at 42–45 °C. The regeneration process of wounds was evaluated by wound area monitoring. To monitor, the wounds were photographed on days 0, 5, 10 and 20. The wound dressings were replaced on day 2 and 5. The wound area was analyzed using ImageJ software. Wound closure performance for any treatment was assessed according to the formula: | Relative wound size (%) = Arean/Area0 × 100%, | (16) |
where Area0 and Arean are the wound areas on day 0, and day 5, 10 or 20, respectively.
2.21 Ethical standards
All animals were housed in an animal room before starting the examination, allowing adaptation to the new environment. Principles of laboratory animal care were followed and the procedure was conducted in accordance with the ethical standards and protocols approved by the committee of animal experimentation guidelines of Zanjan University of Medical Sciences, Zanjan, Iran (ethical code: IR.ZUMS.REC.1400.071).
2.22 Histological analysis
Wound tissue samples were collected on the 5th, 10th, and 20th day for the histopathological study of epidermal regeneration and inflammation in the wound area. The samples were fixed in 10% neutral buffered formalin solution, dehydrated (using ascending alcohols), clarified with xylene, impregnated with melted paraffin, paraffin-embedded, and sectioned to 5 μm thick slices, and finally, stained with hematoxylin and eosin (H&E) and Masson's trichrome. All samples were analyzed using a microscope and a Dino-Lite camera and using Dino-Capture software (V.2).
2.23
In vivo toxicity
The rats (male, 250 g) were randomly divided into 3 groups (n = 2): AGC, AGC–PPP–PDA, and AGC–PRP–PDA groups. The rats were anesthetized using ketamine/xylazine (6
:
4, v/v). 200 μL of sterilized AGC, AGC–PPP–PDA, and AGC–PRP–PDA hydrogels were injected subcutaneously under the rat's dorsal skin. After 7 and 21 days, the rats were sacrificed and the tissues were collected and embedded in 4% formaldehyde. The samples were monitored by H&E staining.
2.24 Statistical analysis
The experimental data were expressed as mean ± standard deviation (SD). Statistical differences were analyzed using a general linear model, repeated measure design and one-way ANOVA, followed by the Bonferroni test for multiple comparisons using SPSS, version 25 (IBM). In all cases, p < 0.05 was considered statistically significant.
3. Results and discussion
3.1 Fabrication and characterization of the hydrogels
The morphology of PDA NPs was identified by SEM (Fig. 2A and B). According to the SEM image, PDA NPs showed a uniform size of 173.9 ± 1.1 nm with a spherical morphology. Zeta potential of the NPs was determined to be −21.3 mV, which was similar to that in previously reported studies (Fig. S2†).54 The cross-linking network AGC–PRP–PDA hydrogel was formed by blending of AGC–PRP–PDA polymeric solution and TBL + CaCl2 aqueous solution as a cross-linker (Fig. 2C). In addition, SEM was applied to observe the morphological features of the lyophilized hydrogels. As shown in Fig. 2D, a 3D structure with open pores was observed in the Alg–Ca hydrogel. The morphology of the AGC hydrogel became denser than Alg–Ca with the introduction of gelatin into the Alg–Ca network. PPP–Ca–TPL and PRP–Ca–TPL hydrogels presented an accumulation of irregular sheet-like fibrin fibers.3,55 In contrast, AGC manifested a porous structure with a wide pore size distribution (Fig. 2D and F). After the introduction of PPP, PRP, and PDA, the porous network structure was retained, and the pore size distribution decreased. Additionally, a wider distribution of pores was observed in AGC–PPP–PDA compared to AGC–PRP–PDA. This can be attributed to the fact that the same volume of PRP and PPP was used in the preparation of the hydrogels. Our measurements showed that the amount of platelets was 3.8 times more in the case of the PRP fraction (Fig. S7†). Therefore, at the same volume, the larger pores were initially filled, thus resulting a noticeable decrease in the pore diameter and pore size distribution in AGC–PRP–PDA than AGC–PPP–PDA (Fig. 2F–H). Energy dispersive spectroscopy (EDS) analysis was employed to analyze the elements of the platelet-containing hydrogels. As shown, the sulfur element recognized in the AGC–PRP–PDA hydrogel, indicating the fibrin networks, could permeate inside the hydrogels, demonstrating successful fabrication of the hydrogels (Fig. 2E).55 Collectively, the three-dimensional and porous structure of the hydrogels can enhance the transmission of oxygen and nutrients, creating a moist environment, and can absorb wound secretions which are beneficial for wound repair.55–57 Furthermore, the successful preparation of AGC, AGC–PRP–PDA, and AGC–PPP–PDA hydrogels was confirmed by FTIR through identifying the surface functional groups of the hydrogels (Fig. 3A). The peak at 530 cm−1 in the AGC, AGC–PPP–PDA, and AGC–PRP–PDA hydrogel spectra is attributed to the metal–oxygen bond, which is considered as the key characteristic peak in the formation of the hydrogels.58 Generally, the spectra of the pure compounds (GT, Alg, and CaCl2) broadened and shifted to higher wavenumbers after the fabrication of AGC, AGC–PPP–PDA, and AGC–PRP–PDA. For example, in the spectrum of calcium chloride hexahydrate, the absorption band at 1630 cm−1 was due to the bending vibration of the H–O–H bond of crystal water.59 In addition, a band at 2900 cm−1 could be related to the C–H stretching vibrations of alginate.60 Such absorption bands broadened and shifted to higher wavelengths in the spectra of AGC–PRP–PDA and AGC–PPP–PDA. These results demonstrated successful fabrication of the hydrogels through attractive interactions between the functional groups of the hydrogels by coordinative and hydrogen bonding interactions.7,17 All of the hydrogels exhibited a high water content, which is essential in the wound repair process (Fig. 3B). The swelling profile of the AGC–PRP–PDA hydrogel was measured by gravimetric analysis (Fig. S3A†). The good swelling properties of AGC–PRP–PDA endow the hydrogels with capabilities to effectively maintain a wet environment during wound healing, absorb the exuded tissue, and control the release of bioactive materials such as growth factors.61,62 An in vitro gel degradation assay was carried out in PBS (pH 7.4). As shown in (Fig. S3B†), degradation of AGC–PRP–PDA increased over time. The UV-vis-NIR absorbance spectra of AGC–PRP–PDA, AGC–PRP–PDA, and PDA showed absorbance in the NIR region (700–900 nm), demonstrating NIR activity of the materials, which is a crucial factor in PTT. As shown, this activity increased with increasing concentration, indicating that the photoactive properties of the materials were concentration-dependent (Fig. 3C and D).
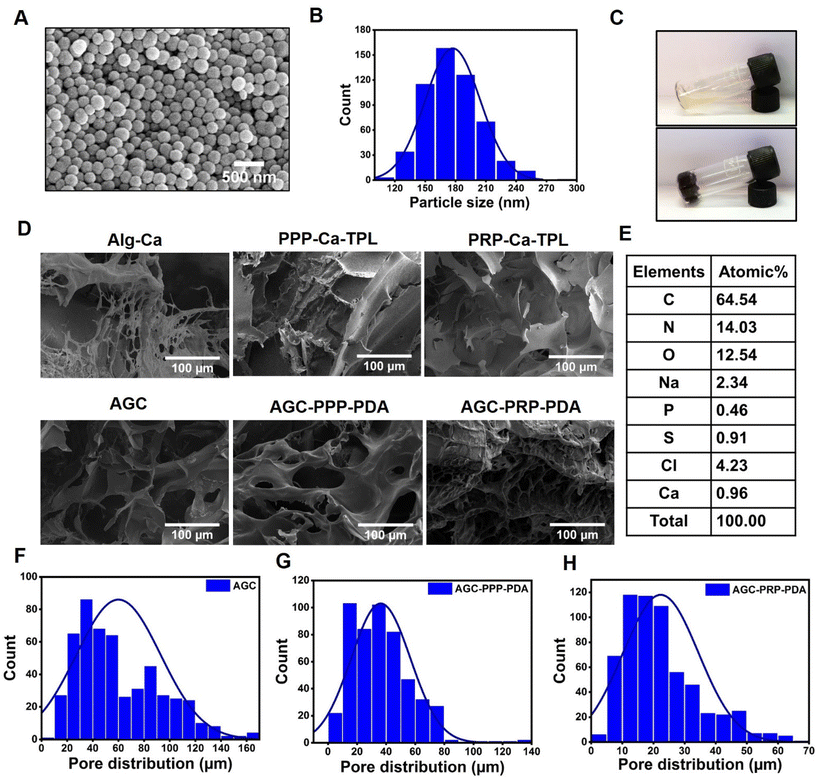 |
| Fig. 2 (A) SEM image of PDA NPs, scale bar: 500 nm. (B) Histogram distribution of PDA NPs. (C) Digital images of AGC (top image) and AGC–PRP–PDA (bottom image) hydrogels. (D) SEM image of the hydrogels. (E) EDS analysis of the AGC–PRP–PDA hydrogel. (F–H) Pore size distribution of the AGC, AGC–PPP–PDA, and AGC–PRP–PDA hydrogels, respectively. | |
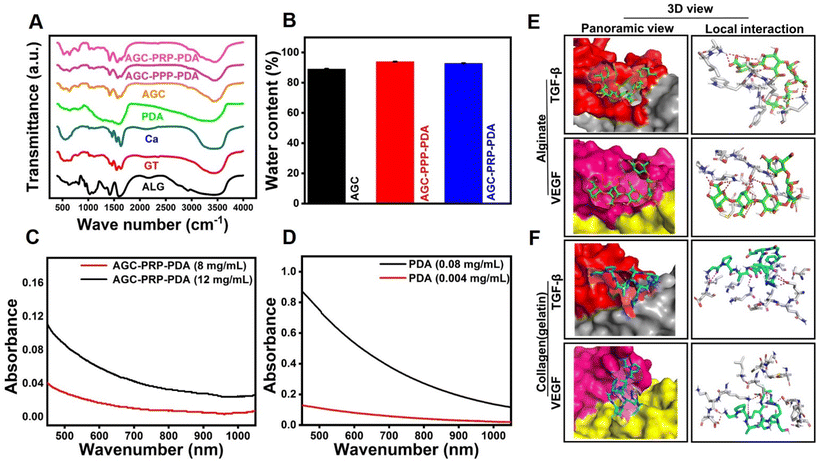 |
| Fig. 3 (A) FTIR spectra of the components of the AGC, AGC–PPP–PDA, and AGC–PRP–PDA hydrogels. (B) Water content of the hydrogels. (C and D) UV-vis-NIR spectra of PDA NPs and AGC–PPP–PDA hydrogels at different concentrations. (E and F) Structures of the complexes of growth factors with hydrogel polymers predicted by docking simulations. The panoramic images show the location of the alginate and collagen (gelatin) fragments on the surface of TGF-β and VEGF. The local interaction images represent the hydrogen bonds between polymers and amino acids surrounding the binding site of the growth factors. | |
3.2 Molecular docking study
The release of the trapped molecules in the hydrogel structure was determined by the various characteristics of the hydrogel at macroscopic and molecular scale levels. The interactions of drugs with polymeric chains of hydrogels such as hydrophobic and electrostatic attractions on an atomic scale play an important role in the sustained release of drugs.63,64 In this study, to investigate the role of the molecular structure of the hydrogels in the slow release of growth factors, i.e., VEGF and TGF-β proteins, a molecular docking study was used to evaluate the interaction of alginate and gelatin as ligands with the proteins (Fig. 3E and F). The value of the binding energy of the best position for each run is presented in Table 1. The more negative binding energy values for all complexes indicate more affinity between the growth factor and alginate/gelatin ligands, resulting from favourable interactions between gelatin and alginate fragments with the neighboring amino acids at the binding site of the proteins. Our computational studies showed that VEGF had much higher affinity toward gelatin than the alginate segment. In contrast, TGF-β exhibited high affinity toward the alginate fragment (Table 1).
Table 1 Binding energy of the top-ranked docking poses for growth factors complexed with hydrogel polymers
Ligand/target |
Binding energy (kcal mol−1) |
Alginate/VEGF |
−0.2 |
Alginate/TGF-β |
−5.21 |
Gelatin/TGF-β |
−4.01 |
Gelatin/VEGF |
−5.80 |
3.3 Rheological performance
The mechanical properties of the hydrogels were evaluated by a rheology amplitude sweep test (Fig. 4). The results showed that the hydrogels had a gel-like behavior with the storage modulus (G′) being higher than the loss modulus (G′′). The addition of PPP and PRP did not significantly affect the rheological properties of the hydrogels, indicating that they were stable and had good consistency. Moreover, the viscosity of the hydrogels decreased with increasing shear rate, indicating their shear thinning properties, thus making the hydrogels to be injectable (Fig. 4E). The hydrogels also exhibited shear-thinning properties, which allowed them to be easily injected using a syringe (Fig. 4F).65,66 In addition, we evaluated the rheological performance of AGC–PRP with/without PDA using a shear thinning assay of the hydrogels. As shown in Fig. S4A,† PDA did not affect the rheological behaviour of AGC–PRP.
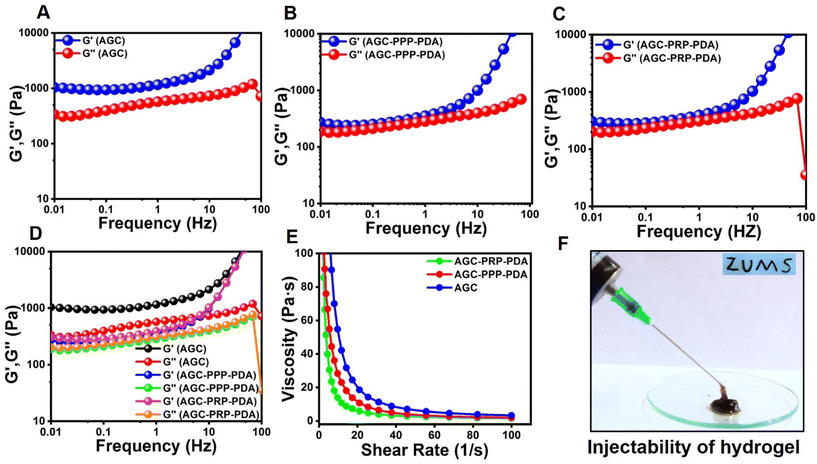 |
| Fig. 4 Rheological performance of the hydrogels. (A) Frequency sweep test of (A) AGC, (B) AGC–PPP–PDA, and (C) AGC–PRP–PDA hydrogels. (D) Rheological performance of the hydrogels determined by employing an oscillation–frequency test. (E) Shear thinning assay of the hydrogels. (F) Injectability test of the AGC–PPP–PDA hydrogel using a 27-G needle. | |
3.4 Photothermal properties of the hydrogels
PTT has been extensively developed as an effective and safe method for bacterial elimination through heat generation from NIR light absorption.34,67 PDA acted as a photothermal agent in the AGC–PRP–PDA hydrogel. As shown in Fig. 5A, the hydrogel without PDA (AGC–PRP) indicated no significant temperature change. The in vitro photothermal effect of the AGC–PRP–PDA hydrogel was examined at various amounts of PDA (5 μL and 10 μL, 5 mg mL−1) and different power densities for 10 min under NIR laser irradiation. As shown in Fig. 5B and C, the temperatures increased with increasing concentration and power densities. Photothermal stability of the hydrogel was investigated by exposing the hydrogels to five heating/cooling cycles (Fig. 5D). The thermal performance of the AGC–PRP–PDA hydrogel showed no difference during the five circles of NIR irradiation and free cooling continuous illumination, indicating good photostability of the hydrogel. Additionally, the photothermal conversion efficiency (η) was measured by obtaining the value of τs (τs = 297.27).68,69 The η value of the AGC–PRP–PDA hydrogel was calculated to be 18.2% (Fig. 5E and F, and eqn (2)). The infrared thermographic image of the AGC–PRP–PDA hydrogel was recorded by NIR laser irradiation (808 nm, 1 W cm−2) (Fig. 5G and H). After 10 min of irradiation, there was no noticeable temperature increase for the hydrogel without PDA just at about 4.5 °C, whereas the temperature of the hydrogel with PDA increased instantaneously by 24 °C. We also observed that the photothermal activity of AGC–PRP–PDA and AGC–PPP–PDA hydrogels was similar (data are not shown).
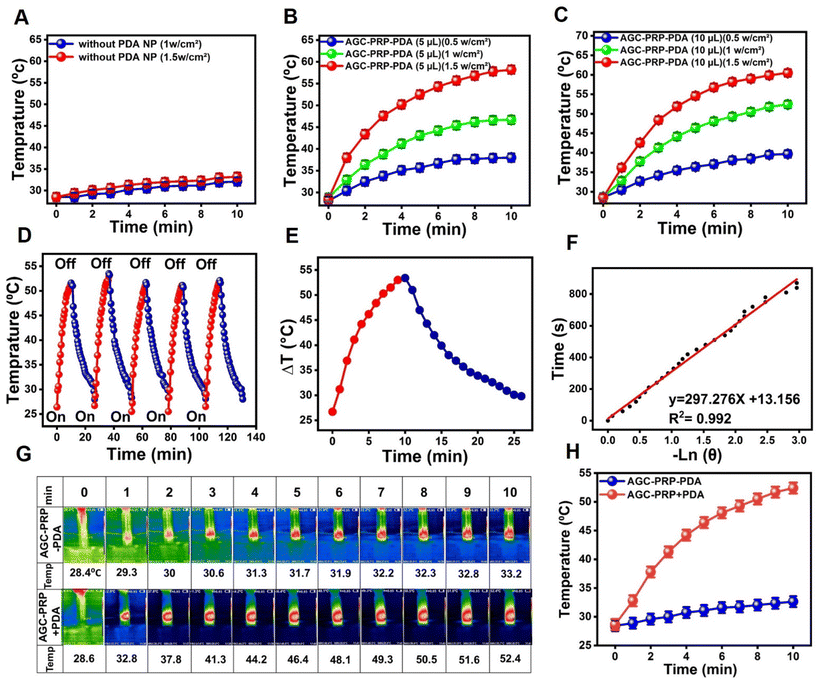 |
| Fig. 5 (A) Temperature elevation of the AGC–PRP hydrogel at different powers of NIR light (808 nm). (B and C) Temperature increase profiles of the AGC–PRP–PDA hydrogel at different concentrations and powers over 10 min. (D) Temperature changes of AGC–PRP–PDA during five on/off cycles of NIR laser illumination at 1.0 W cm−2 for 130 min. (E) Photothermal response of the AGC–PRP–PDA hydrogel for one cycle using a 808 nm laser (1 W cm−2). (F) Linear time data versus −ln θ measured using the cooling period. (G and H) Infrared thermal images and the temperature profile of the AGC–PRP–PDA hydrogel under 808 nm laser irradiation (1 W cm−2) (mean ± SD, n = 3). | |
3.5 NIR-assisted antibacterial properties of the hydrogels
The essential factor in wound repair is the inhibition of bacterial infection.5,39,41 The antibacterial activity of the hydrogels was tested against the Gram-negative and Gram-positive bacterial strains in the presence and absence of 808 nm NIR light. Normal saline and the AGC hydrogel were taken as the control groups (Fig. 6). When introducing PDA as a photothermal agent, the AGC–PPP–PDA and AGC–PRP–PDA hydrogels showed a considerably decreased bacteria survival ratio of 22.88% for E. coli and 37.34% for S. aureus, and 29.16% for E. coli and 18.38% for S. aureus, respectively. In contrast, the bacteria survival ratio of these hydrogels without NIR light for E. coli and S. aureus had not been reduced compared to the control group. There were no obvious change in the colonies in the control group with or without NIR light. According to Fig. 6B–D, no bacterial killing occurred in the AGC hydrogel group both in the presence/absence of NIR irradiation. Furthermore, the antibacterial performance of PDA NPs was tested against E. coli and S. aureus based on the photothermal ability in LB broth medium (Fig. S5†). It has been reported that bacterial strains can be killed during PTT when the temperature is increased to 45 °C.24,67,70 The generated heat could cause destruction of bacteria by damage to the cellular membrane and intracellular proteins.39,71,72
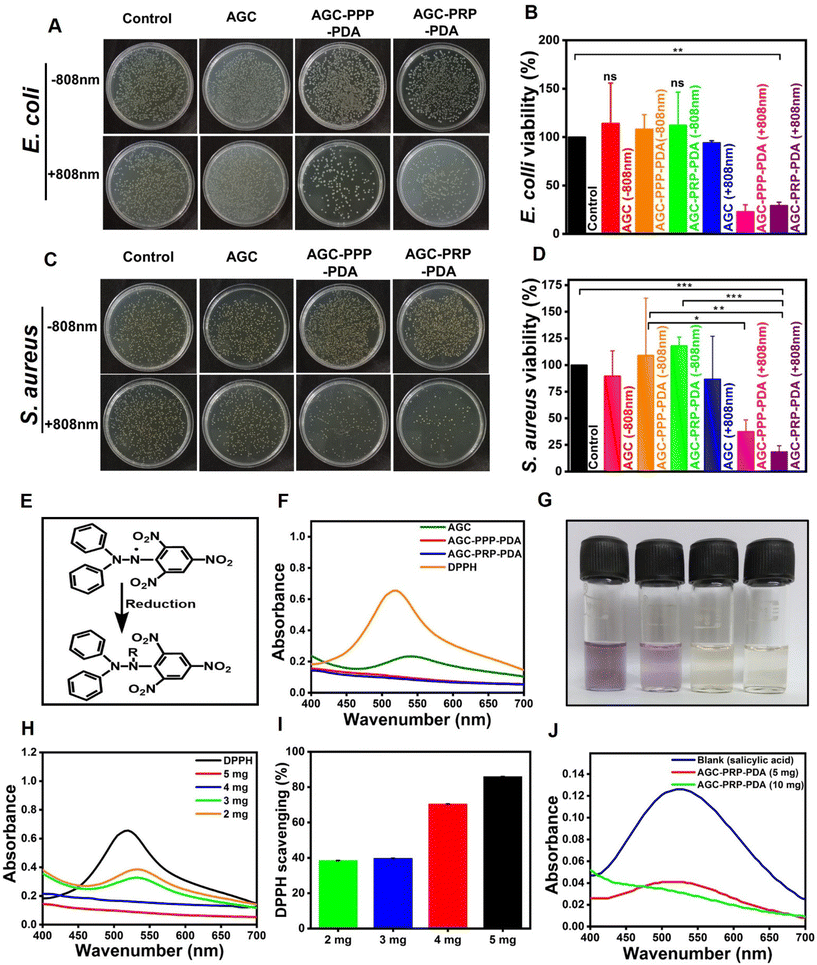 |
| Fig. 6 Illustration of the antibacterial activity of the hydrogels with and without NIR light. (A) Image of the agar plate indicating E. coli growth. (B) Viability ratio of E. coli. (C) Image of the agar plate indicating S. aureus growth. (D) Viability ratio of S. aureus growth of the hydrogels with and without NIR light (mean ± SD, n = 3, *p < 0.05, **p < 0.01). Antioxidant performance of the hydrogels. (E) Reduction mechanism of DPPH. (F) Absorbance curve of different hydrogels and DPPH. (G) Digital images of DPPH and the hydrogel solutions. (H) Absorbance spectra of different amounts of the hydrogels and DPPH. (I) Scavenging rate of different amounts of the hydrogels. (J) Antioxidant activity of the AGC–PRP–PDA hydrogel with salicylic acid. | |
3.6 Antioxidant properties of the hydrogels
There is a high amount of ROS produced through oxidative stress at a wound site, thus resulting oxidation of proteins and lipids, or DNA damage.73 Therefore, design and fabrication of multifunctional wound dressings with antioxidant properties is of particular importance. Free radical scavenging capacity of PDA has been reported related to the catechol-rich surface of PDA and their quinone groups.9,74–76 As shown in Fig. 6F, the visible spectroscopy of the DPPH solution showed an obvious absorption at 517 nm. The indicator was a stable RNS compound and its ethanol solution color was purple.42 The decolorization of DPPH in AGC, AGC–PPP–PDA, and AGC–PRP–PDA solutions is shown in Fig. 6G. When the DPPH solution was exposed with AGC–PPP–PDA and AGC–PRP–PDA dispersions, the color of the solutions was completely faded and there was no absorbance peak at 517 nm, indicating reduction of DPPH by the hydrogels (Fig. 6F and G). Furthermore, the DPPH scavenging performance of the AGC–PRP–PDA hydrogel indicated a concentration-dependent behaviour. The DPPH scavenging ratio increased from 38% to 86% when the amount of the AGC–PRP–PDA hydrogel increased from 2 mg to 5 mg (Fig. 6H and I). In an another attempt, the antioxidant properties of the AGC–PRP–PDA hydrogel were assessed using salicylic acid as an indicator.77 As shown in Fig. 6J, the absorption peak of salicylic acid disappeared upon using the AGC–PRP–PDA hydrogel at 517 nm. On the other hand, this effect was promoted with increasing concentration of the hydrogel. In addition, excellent H2O2 scavenging properties were found upon using AGC–PRP–PDA (Fig. S6†). We used ascorbic acid as a positive antioxidant agent. At a lower concentration (6 mg mL−1), the scavenging percentage was similar to that of ascorbic acid, while at a higher concentration (10 mg mL−1), the H2O2 scavenging activity (%) of AGC–PRP–PDA was significantly higher than ascorbic acid (P < 0.01). The above results demonstrated that the existence of PDA endowed the hydrogel with excellent antioxidant properties, thus, highlighting the potential application of the antioxidant hydrogels for skin wound regeneration.39
3.7
In vitro cytotoxicity and biocompatibility evaluations
A desirable wound dressing should possess hemostatic capacity with good hemocompatibility.35,78 Hemocompatibility of AGC, AGC–PPP–PDA, and AGC–PRP–PDA hydrogels was investigated by a hemolysis test (Fig. 7A–D). As shown, all of the hydrogels showed a negligible hemolysis ratio (<2.5%), exhibiting excellent hemocompatibility in vitro. In the hemolytic test, deionized water (positive control group) showed an apparent red color, while the hydrogel groups showed the same color as PBS (negative control group) (Fig. 7D). Cytocompatibility evaluation is an essential factor for any biomaterial to be used in biomedical applications (Fig. 7E and F).56,79,80 Fibroblast cells plays a main role in the wound healing process and their proliferation and migration accelerate wound closure.68,81 Cytocompatibility of the hydrogels was examined with NIH3T3 fibroblast cells using an MTT assay.
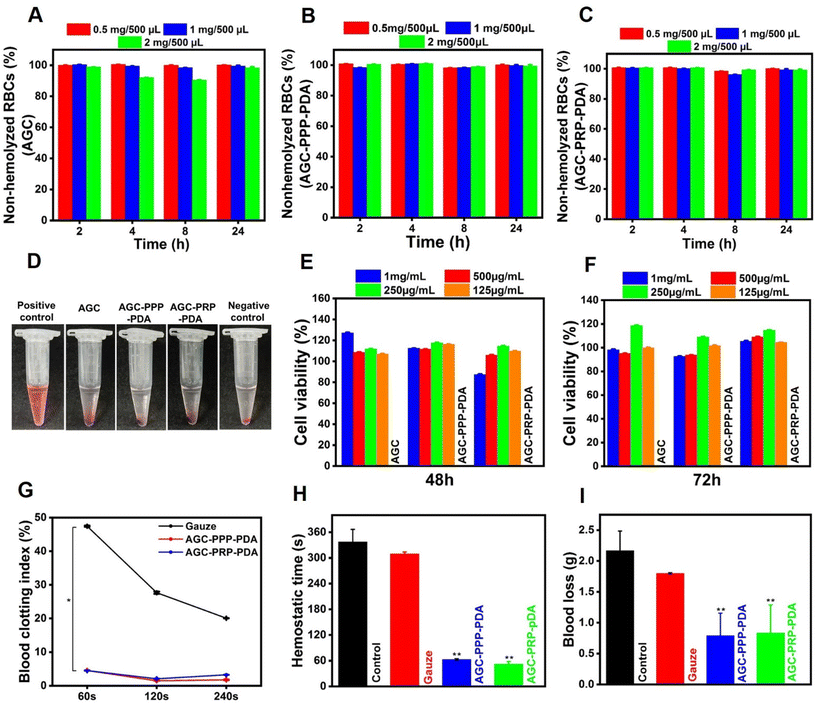 |
| Fig. 7 Biocompatibility evaluation and hemostatic performance of the hydrogels. In vitro cell viability assays of (A) AGC, (B) AGC–PPP–PDA, and (C) AGC–PRP–PDA hydrogels at different concentrations. (D) Images of the supernatants of RBC suspensions treated with deionized water, the hydrogels, and PBS (pH ∼ 7.4). Cell viability evaluation of the hydrogels at (E) 48 h and (F) 72 h (mean ± SD, n = 3, *p < 0.05, **p < 0.01). (G) In vitro BCI index of the hydrogels, gauze, and control groups after 60, 120, and 240 s. (H and I) In vivo hemostatic time blood loss evaluation in a wound tail amputation model for the different groups (mean ± SD, n = 3, *p < 0.05, **p < 0.01). | |
During co-incubation for 48 and 72 h, all groups had higher than ∼90% cell viability even at a concentration of 1 mg mL−1, indicating cytocompatibility of the hydrogels. Such findings demonstrated that all the hydrogels had desirable hemocompatibility and cytocompatibility, making them excellent candidates for subsequent wound regeneration.
3.8 Hemostatic performance of the hydrogels
During an injury in the skin, bleeding is unavoidable. Therefore, evaluation of hemostasis should be the first stage in the wound repair assessment.82In vitro blood clotting properties of the hydrogels and gauze were measured using the whole blood-clotting index three times (60, 120, and 240 s) at 37 °C.7 Gauze as a traditional hemostatic model was used as the control. Both AGC–PPP–PDA, and AGC–PRP–PDA groups indicated a lower BCI than the control group and no significant difference between the AGC–PPP–PDA and AGC–PRP–PDA groups was observed (Fig. 7G). When the AGC–PRP–PDA or AGC–PPP–PDA hydrogels were placed as powder for wound tail amputation, blood loss was quickly stopped at 52 and 63 s, respectively; the timings were significantly shorter than those of the control group (337 s), confirming a promising performance of the hydrogels as a hemostatic agent (Fig. 7H). In addition, the in vivo hemostatic properties of the hydrogels were further evaluated by the amount of blood loss in the model. Total blood loss in the control group (without treatment) was calculated to be 2.1 g in 337 s. In contrast, the amount of blood loss was ∼1 g in the AGC–PRP–PDA and AGC–PPP–PDA hydrogel-treated groups at 52 and 63 s, respectively (Fig. 7H and I). Hemostatic images under different treatments also clearly showed the anti-bleeding properties of the hydrogels (Fig. S8†). On one hand, the hemostasis capacity of the hydrogels in the tail amputation model might be related to the strong adhesiveness between the amputated tail and the PDA-containing hydrogels acting as a physical barrier for bleeding. PDA has catechol groups, endowing the material with high adhesive properties. Therefore, wound dressings containing PDA possess enhanced adhesiveness to a wounded tissue, thus improving the hemostatic effect of the dressing.39 On the other hand, the hemostatic function of platelets endows AGC–PRP–PDA and AGC–PPP–PDA hydrogels with antibleeding properties to promote hemostasis immediately after the injury.33 Therefore, the abovementioned properties introduced the multifunctional hydrogels as a good candidate in wound closure for their excellent anti-bleeding performance.
3.9
In vitro growth factor release evaluation
VEGF and TGF-β play an important role for promoting wound healing. VEGF is a key dynamic molecule stimulating angiogenesis. In addition, it is involved in the migration process of fibroblast cells to accelerate wound closure.30,83 TGF-β regulates not only angiogenesis, inflammation, and granulation tissue formation, but also re-epithelialization, thus accelerating the healing process.84 The rapid release of growth factors from PRP limits its clinical applications.3 In order to achieve a sustained release of growth factors, loading PRP into a hydrogel is a promising strategy to improve wound healing efficiency.56,57,85 An ELISA assay was used to evaluate the release curves of VEGF and TGF-β (Fig. 8A and B). As shown, the burst release of the PRP gel (without Alg, the gel, and the cross-linker) was realized in the initial hours, while the release behaviours of VEGF and TFG-β were relatively slower, indicating better sustained release properties of the growth factors in the AGC–PRP–PDA hydrogel compared to the PRP gel.
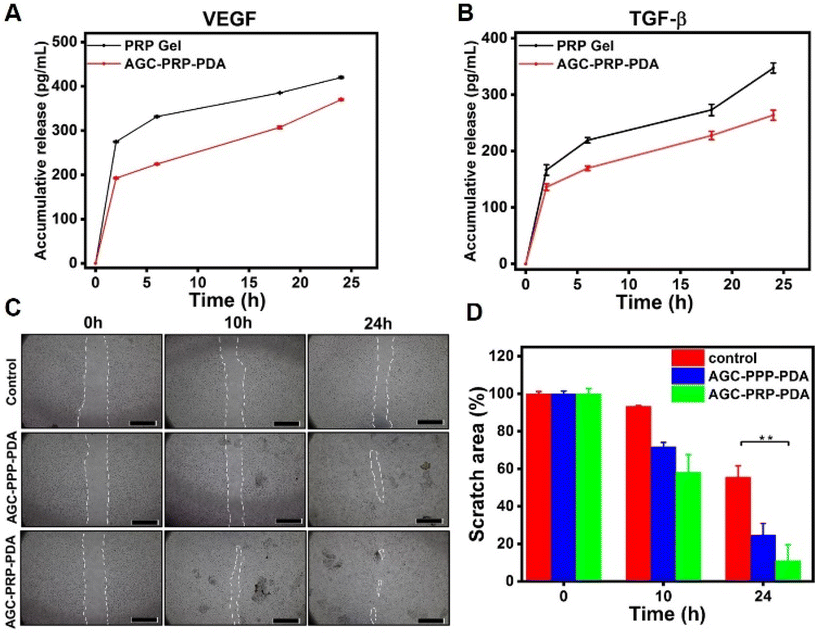 |
| Fig. 8 Release profiles of (A) VEGF and (B) TGF-β from the PRP gel and AGC–PRP–PDA hydrogel (mean ± SD, n = 3). In vitro wound healing (scratch assay) of the AGC–PPP–PDA and AGC–PRP–PDA hydrogels, and (C) the scratch image of NIH3T3 cells. (D) Relative healing area obtained for the hydrogel and control groups (mean ± SD, n = 3, **p < 0.01), scale bar: 1 mm. | |
3.10
In vitro wound healing assay
To evaluate whether the AGC–PRP–PDA hydrogel could promote the migration of repaired cells, a scratch assay was performed using NIH3T3 cells. The in vitro test is a well-known method based on creating a “scratch” on a cell monolayer mimicking to some extent cell–cell and cell–matrix interactions during wound healing in vivo.86 The results showed that the proliferation of NIH3T3 cells was stimulated by AGC–PRP–PDA and AGC–PPP–PDA hydrogels faster than the control (without treatment) group (Fig. 8C). After 24 h, the relative wound areas in the AGC–PRP–PDA, AGC–PPP–PDA hydrogels and control group were determined to be 11%, 24.8%, and 55.5%, respectively (Fig. 8D). As shown, compared with AGC–PRP–PDA, the in vitro wound healing was significantly higher than AGC–PPP–PDA. This can be attributed to the higher expression of growth factors in the case of AGC–PRP–PDA, thus accelerating the repair of the scratch area effectively.26,87 For example, it has been shown that the platelet derived growth factors such as VEGF could regulate cell proliferation and migration.88 Moreover, we measured the amount of platelets at similar volumes of PRP and PPP fractions. The amount was 3.8 times more in the case of the PRP fraction (Fig. S7†). It should be noted that gelatin can promote cell adhesion and proliferation, thus accelerating wound closure.14 These results show that PRP/PPP-loaded hydrogels could stimulate fibroblast growth, suggesting the possibility of prompting the wound healing process in vivo.
3.11
In vivo wound healing study
The PRP-loaded hydrogels presented sustained release of growth factors and exhibited excellent photothermal antibacterial activity as well as improved cell migration behaviors in our in vitro studies. The therapeutic efficiency of the hydrogels was evaluated using an infected wound model. We established a 10 mm diameter full-thickness skin wound on the back of rats, followed by infecting the wound site using S. aureus (∼108 CFU mL−1). Except for the control group (no treatment), wounds were covered by the hydrogels and monitored on days 5, 10, and 20 during the healing process. Alfa ointment (available in the market) was used for the control group (Fig. 9A). Compared with the other groups, the AGC–PRP–PDA (+NIR) treated group showed a faster wound closure rate. Quantitative wound closure results are shown in Fig. 9E. On day 5, the AGC–PRP, AGC–PRP–PDA (±NIR) and AGC–PRP–PDA (±NIR) groups healed significantly faster than the other groups (p < 0.05) and statistical analysis suggested that there was no significant difference among the control, Alfa, and AGC groups. On days 10 and 20, wound closure occurred at both times and there was no significant difference among the groups on each day (Fig. 9C–E). The increased healing in AGC–PRP–PDA (±NIR) than the control group can be attributed to the antioxidant, antibacterial, and healing properties of the Alg–Gel-based hydrogels as well as the maintenance of a wet environment during wound healing by the hydrogel. In vivo antibacterial properties of AGC–PRP–PDA (−NIR) and AGC–PRP–PDA (+NIR) hydrogels were evaluated and the corresponding results are shown in Fig. 9F. The anti-infective effects were assessed by comparing the bacterial counts in the infected wounds. After 24 h of treatment, the control (without any treatment) and the AGC–PRP–PDA (−NIR) groups indicated a large number of surviving bacteria on the plate. In contrast, the AGC–PRP–PDA (+NIR) hydrogel showed a noticeable reduction in the bacteria number, providing a promising goal to use it as an excellent dressing to treat S. aureus-infected wounds (Fig. 9F). This finding highlighted the antibacterial activity of the hydrogel under NIR light irradiation stemming from PDA.39,89Fig. 9G shows the infrared thermal images of the treated groups with AGC–PRP–PDA under 808 nm laser irradiation (1 W cm−2).
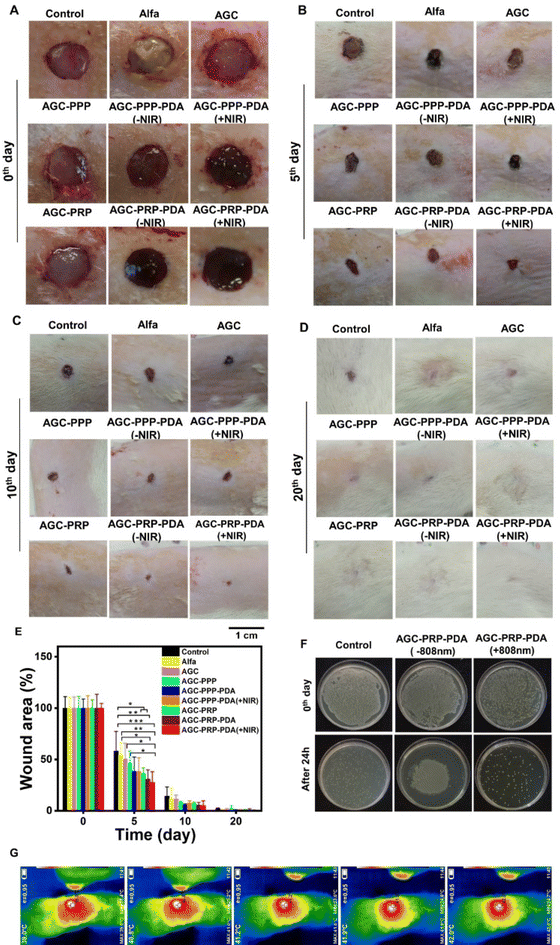 |
| Fig. 9
In vivo skin regeneration and antibacterial assay of the hydrogels. Macroscopic view of the wounds on (A) 0th, (B) 5th, (C) 10th and (D) 20th days. (E) Statistical wound closure ratio of all groups (mean ± SD, n = 5, *p < 0.05, **p < 0.01, ***p < 0.001). (F) In vivo antibacterial properties of AGC–PRP–PDA with and without NIR light irradiation after 24 h of treatment. (G) In vivo infrared thermal images of the wounds covered by the AGC–PRP–PDA hydrogel under 808 nm (1 W cm−2), scale bar: 1 cm. | |
As shown, the temperature at the wound site rapidly increased to 42 °C for 5 min, indicating the excellent photothermal activity of the hydrogel in vivo (Fig. 9G).
3.12 Histological analysis
To further evaluate the quality of the regenerated skin, H&E and Masson's trichrome staining on day 5, day 10, and day 20 were performed (Fig. S10†). As shown in Fig. 10A, after 5 days of the treatment, all groups indicated varying degrees of inflammatory cells, indicating the typical first stage of the wound repair process. New blood vessels are essential for wound healing, to provide nutrients and oxygen to the damaged area.39,90,91 On day 5, the AGC–PPP–PDA (+NIR) and AGC–PRP–PDA (+NIR) groups exhibited more blood vessels than the other groups. On day 10, the AGC–PRP–PDA (+NIR) and AGC–PRP–PDA (−NIR) groups were covered with a thin layer of epithelial tissue. The AGC–PPP–PDA (+NIR) and AGC–PPP–PDA (−NIR) groups exhibited partial re-epithelialization on the edge of the wound. In addition, PRP-loaded hydrogels showed less inflammatory cell infiltration at the wound site than the PPP-loaded ones. However, no obvious epithelialization was observed for the other groups (Fig. 10B). On day 20, the wound in the AGC–PRP–PDA (+NIR) group reached the state of normal skin structure, and many skin accessory structures including mature blood vessels, hair follicles, and dermis thickening were observed in the group than the AGC–PRP–PDA (−NIR) group (Fig. S9†). Furthermore, it was observed that the epithelial thickness of the AGC–PRP–PDA groups at day 20 was reduced compared with the AGC–PPP–PDA groups, indicating a transition from phase three (proliferation) to phase four (remodeling) of a healing process (Fig. 10C).92 Masson's trichrome staining was used to track collagen deposition throughout the wound repair process on day 20 (Fig. 10D). As shown, no obvious difference in collagen deposition was observed among the control, Alfa, AGC, AGC–PPP, and AGC–PRP groups. In addition, number of collagen fibers in the PRP- and PPP-containing groups was much higher when NIR light was used, confirming that collagen deposition was improved by hyperthermia during the PTT. Compared with AGC–PPP–PDA (+NIR), well-arranged collagen deposition with dense fibers was observed in the AGC–PRP–PDA (+NIR) treated group, indicating the impact of the release growth factors in the healing process, which was more in the AGC–PRP–PDA group. These results demonstrated that AGC–PRP–PDA (+NIR) would be a promising hydrogel dressing for cutaneous tissue remodeling and regeneration.
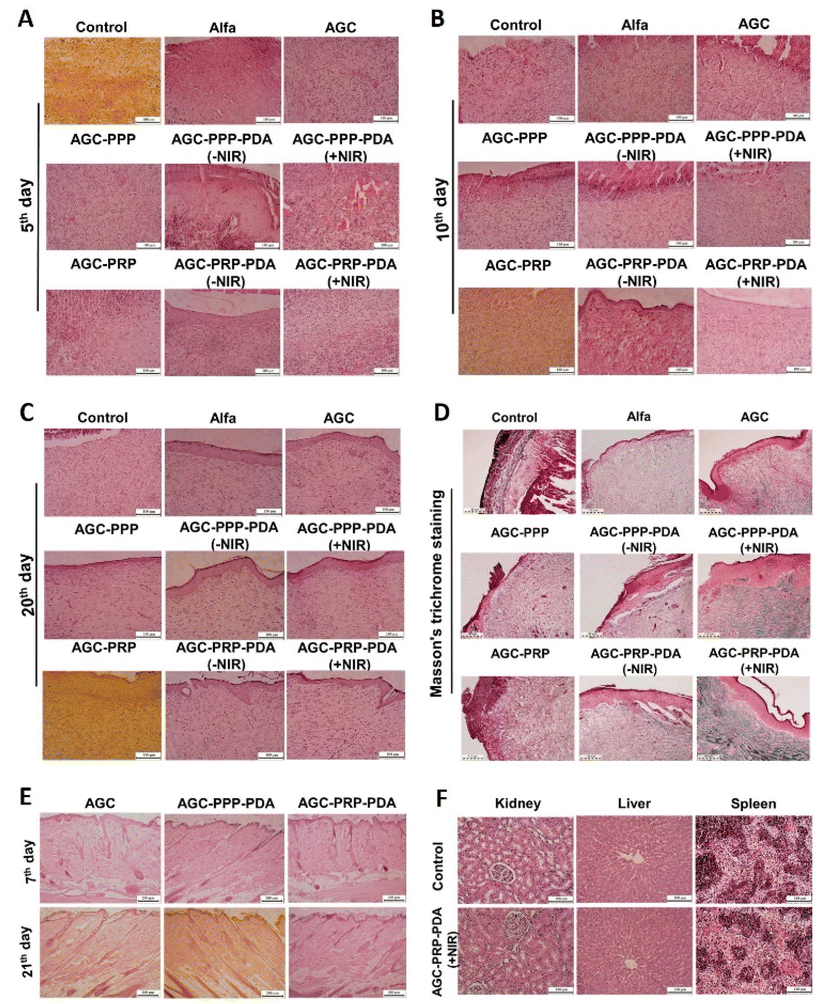 |
| Fig. 10 Histological analysis of the wound area with H&E staining after the (A) 5th, (B) 10th, and (C) 20th day of therapy for all groups, scale bar: 200 μm. (D) Masson's trichrome staining histological images on day 20 for all groups, scale bar: 110 μm. In vivo biocompatibility and biosafety assessment. (E) H&E staining image of the skin tissues after subcutaneous implantation on days 7 and 21. Scale bar: 200 μm. (F) Histological evaluation of the kidneys, livers and spleens of the control and AGC–PRP–PDA groups. Scale bar: 100 μm. | |
3.13
In vivo biosafety evaluation of the hydrogels
In vivo biocompatibility is of vital importance for the hydrogels applied in regenerative medicine.67 Biocompatible properties of AGC, AGC–PPP–PDA, and AGC–PRP–PDA hydrogels were evaluated by subcutaneous injection of the hydrogel into the shaved dorsal side of a male rat (Fig. 10E). The results were assessed by H&E staining on day 7 and day 21. On both days, epidermis, dermis, and hypodermis were similar to the natural skin. As shown in H&E images, inflammation was not observed and hair follicles were visible. In addition, fibroblasts and fibrocytes were the main connective cells. These findings indicated biocompatibility of the hydrogels. Moreover, the histological analysis of the major organs (kidneys, livers, and spleens) in the AGC–PRP–PDA (+NIR)-treated group was used to further evaluate the biocompatibility of the hydrogel. As shown in Fig. 10F, no obvious pathological abnormalities or inflammation was observed in the treatment group.
Hematology and blood biochemical analysis were used to further evaluate the biosafety of the treatment groups (control, Alfa, AGC–PRP, and AGC–PRP–PDA) on day 20. As shown in Fig. 11A, the white blood cell (WBC) level in the control group was much higher than those of the Alfa, AGC, and AGC–PRP–PDA groups. This is because WBCs are involved in inflammatory responses and their amount are usually used as an index in the evaluation of inflammatory activities.24 In addition, there were no significant differences in red blood cells (RBCs), total protein (TP), hemoglobin (HGB), hematocrit test (HCT), and renal function parameters [blood urea nitrogen (BUN) and creatinine (CRE)], as well as liver function parameters [albumin (Alb) and alkaline phosphatase (Alp)] between the control group and the Alfa, AGC, and AGC–PRP–PDA groups. These findings confirmed good in vivo biocompatibility of the hydrogels.
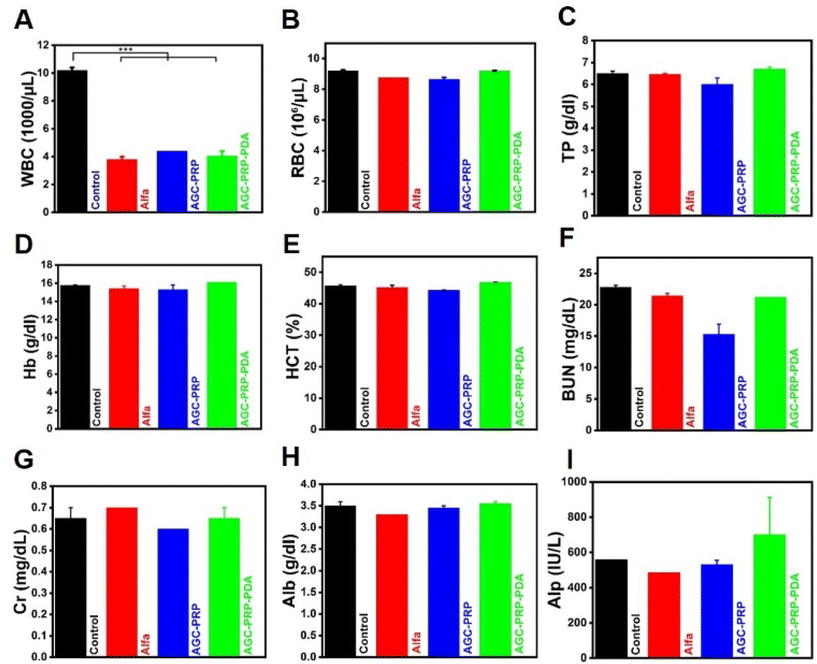 |
| Fig. 11 Hematology and blood biochemical analysis of (A) WBCs, (B) red blood cells (RBCs), (C) blood platelets (PLT), (D) blood urea nitrogen (BUN), (E) creatinine (CRE), (F) lactate dehydrogenase (LDH), (G) haemoglobin (HGB), (H) alkaline phosphatase (Alp), and the (I) hematocrit test (HCT) for the control, Alfa, AGC–PRP, and AGC–PRP–PDA groups (mean ± SD, n = 2, ***p < 0.001). | |
4. Conclusion
Overall, this study highlights the potential of PRP-loaded hydrogels as a promising approach for the treatment of infected full-thickness skin defects. The combination of Alg and gelatin provided a stable and shear-thinning hydrogel with inherent photothermal effects, while the addition of PRP allowed for sustained release of growth factors to promote cell proliferation and angiogenesis. The hydrogel also demonstrated excellent biocompatibility, hemostatic effects, antioxidant properties, and antibacterial activity, making it a multifunctional wound dressing. In a mouse-tail amputation model, the photothermally active AGC–PRP–PDA hydrogel significantly accelerated the healing process of full-thickness skin defects infected by S. aureus. These findings have important implications for the design of novel bio-photoactive injectable hydrogels for wound healing and skin reconstruction.
Author contributions
Vajihe Alinezhad carried out the experiments with the support from Hadi Bagheri and Kimia Esmaeilzadeh. Habib Zeighami supervised the antibacterial studies. Rahim Jafari and Kimia Esmaeilzadeh performed the computational analysis. Pooyan Makvandi performed SEM and rheological analysis. Ali Kalantari-Hesari carried out the histopathological analysis. Yi Xu, Hamid Reza Mohammadi, and Mohammad-Ali Shahbazi revised the manuscript. Aziz Maleki directed and designed the project.
Conflicts of interest
There are no conflicts to declare.
Acknowledgements
This work was supported by the Zanjan University of Medical Sciences (Grant No. A-12-1143-5 and A-12-1291-6).
References
- F. Z. Amourizi, A. Z. Malek-Khatabi and R. Zare-Dorabei, Mater. Chem. Horiz., 2023 DOI:10.22128/MCH.2023.645.1036.
- G. Heidari, M. Hassanpour, F. Nejaddehbashi, M. R. Sarfjoo, S. Yousefiasl, E. Sharifi, A. Bigham, T. Agarwal, A. Borzacchiello and E. Lagreca, Mater. Chem. Horiz., 2022, 1, 35–48 Search PubMed.
- J. Tang, H. Li, H. Peng, Z. Zhang, C. Liu, Y. Cheng, K. Wang, Z. Yu, Z. Lyu and J. Zhang, Mater. Today Bio, 2022, 17, 100498 CrossRef CAS.
- H. N. Wilkinson and M. J. Hardman, Open Biol., 2020, 10, 200223 CrossRef CAS.
- A. Maleki, J. He, S. Bochani, V. Nosrati, M.-A. Shahbazi and B. Guo, ACS Nano, 2021, 15, 18895–18930 CrossRef CAS PubMed.
- H. Wang, Z. Xu, M. Zhao, G. Liu and J. Wu, Biomater. Sci., 2021, 9, 1530–1546 RSC.
- S. Bochani, A. Kalantari-Hesari, F. Haghi, V. Alinezhad, H. Bagheri, P. Makvandi, M.-A. Shahbazi, A. Salimi, I. Hirata and V. Mattoli, ACS Appl. Bio Mater., 2022, 5, 4435–4453 CrossRef CAS.
- R. Kushwaha, S. Sharma, S. Kumar and A. Kumar, Mater. Chem. Horiz., 2023, 2, 11–39 Search PubMed.
- Y. Li, R. Fu, Z. Duan, C. Zhu and D. Fan, ACS Nano, 2022, 16, 7486–7502 CrossRef CAS PubMed.
- W. Peng, D. Li, K. Dai, Y. Wang, P. Song, H. Li, P. Tang, Z. Zhang, Z. Li and Y. Zhou, Int. J. Biol. Macromol., 2022, 208, 400–408 CrossRef CAS PubMed.
- Y.-W. Ding, Z.-Y. Wang, Z.-W. Ren, X.-W. Zhang and D.-X. Wei, Biomater. Sci., 2022, 10, 3393–3409 RSC.
- M. Abazari, T. Akbari, M. Hasani, E. Sharifikoloue, M. Raoufi, A. Foroumadi, M. Sharifzadeh, L. Firoozpour and M. Khoobi, Carbohydr. Polym., 2022, 119808 CrossRef CAS PubMed.
- H. Hu and F.-J. Xu, Biomater. Sci., 2020, 8, 2084–2101 RSC.
- P. Jaipan, A. Nguyen and R. J. Narayan, MRS Commun., 2017, 7, 416–426 CrossRef CAS.
- T. C. Tseng, L. Tao, F. Y. Hsieh, Y. Wei, I. M. Chiu and S. h. Hsu, Adv. Mater., 2015, 27, 3518–3524 CrossRef CAS PubMed.
- X.-H. Wang, F. Song, D. Qian, Y.-D. He, W.-C. Nie, X.-L. Wang and Y.-Z. Wang, Chem. Eng. J., 2018, 349, 588–594 CrossRef CAS.
- B. Tao, C. Lin, Y. Deng, Z. Yuan, X. Shen, M. Chen, Y. He, Z. Peng, Y. Hu and K. Cai, J. Mater. Chem. B, 2019, 7, 2534–2548 RSC.
- F. Wahid, Y.-N. Zhou, H.-S. Wang, T. Wan, C. Zhong and L.-Q. Chu, Int. J. Biol. Macromol., 2018, 114, 1233–1239 CrossRef CAS PubMed.
- H. Chen, C. Peng, L. Wang, X. Li, M. Yang, H. Liu, H. Qin and W. Chen, Chem. Eng. J., 2021, 403, 126341 CrossRef CAS.
- M. Li, X. Liu, L. Tan, Z. Cui, X. Yang, Z. Li, Y. Zheng, K. W. K. Yeung, P. K. Chu and S. Wu, Biomater. Sci., 2018, 6, 2110–2121 RSC.
- P. Makvandi, M. Shabani, N. Rabiee, Q. K. Anjani, A. Maleki, E. N. Zare, A. H. B. Sabri, D. De Pasquale, M. Koskinopoulou and E. Sharifi, Adv. Mater., 2023, 2210034 CrossRef CAS PubMed.
- T. Su, M. Zhang, Q. Zeng, W. Pan, Y. Huang, Y. Qian, W. Dong, X. Qi and J. Shen, Bioact. Mater., 2021, 6, 579–588 CrossRef CAS PubMed.
- D. Gan, T. Xu, W. Xing, X. Ge, L. Fang, K. Wang, F. Ren and X. Lu, Adv. Funct. Mater., 2019, 29, 1805964 CrossRef.
- G. Gao, Y.-W. Jiang, H.-R. Jia and F.-G. Wu, Biomaterials, 2019, 188, 83–95 CrossRef CAS PubMed.
- S. Barrientos, O. Stojadinovic, M. S. Golinko, H. Brem and M. Tomic-Canic, Wound Repair Regen., 2008, 16, 585–601 CrossRef PubMed.
- T. N. Demidova-Rice, M. R. Hamblin and I. M. Herman, Adv. Skin Wound Care, 2012, 25, 304 CrossRef PubMed.
- S. Wei, P. Xu, Z. Yao, X. Cui, X. Lei, L. Li, Y. Dong, W. Zhu, R. Guo and B. Cheng, Acta Biomater., 2021, 124, 205–218 CrossRef CAS PubMed.
- T. Li, H. Lu, L. Zhou, M. Jia, L. Zhang, H. Wu and L. Shan, Cell Proliferation, 2022, 55, e13212 CrossRef CAS PubMed.
- S. M. Oliveira, V. E. Santo, M. E. Gomes, R. L. Reis and J. F. Mano, Biomaterials, 2015, 48, 56–65 CrossRef CAS PubMed.
- Z. Zheng, M. Li, P. Shi, Y. Gao, J. Ma, Y. Li, L. Huang, Z. Yang and L. Yang, Bioact. Mater., 2021, 6, 2613–2628 CrossRef CAS PubMed.
-
J. Milstone, Fed. Proc., 1964, 23, 742–748 Search PubMed.
-
N. Nayyar, H. Mannasian, L. Yang and H. Liu, Essentials of Blood Product Management in Anesthesia Practice, 2021, pp. 29–35 Search PubMed.
- E. Anitua, P. Nurden, R. Prado, A. T. Nurden and S. Padilla, Biomaterials, 2019, 192, 440–460 CrossRef CAS PubMed.
- Y. Liu, K. Ai, J. Liu, M. Deng, Y. He and L. Lu, Adv. Mater., 2013, 25, 1353–1359 CrossRef CAS PubMed.
- L. Wang, X. Zhang, K. Yang, Y. V. Fu, T. Xu, S. Li, D. Zhang, L. N. Wang and C. S. Lee, Adv. Funct. Mater., 2020, 30, 1904156 CrossRef CAS.
- J. Qu, X. Zhao, P. X. Ma and B. Guo, Acta Biomater., 2017, 58, 168–180 CrossRef CAS PubMed.
- P. Yang, S. Zhang, N. Zhang, Y. Wang, J. Zhong, X. Sun, Y. Qi, X. Chen, Z. Li and Y. Li, ACS Appl. Mater. Interfaces, 2019, 11, 42671–42679 CrossRef CAS PubMed.
- K. Musaie, S. Abbaszadeh, V. Nosrati-Siahmazgi, M. Qahremani, S. Wang, M. R. Eskandari, S. V. Niknezhad, F. Haghi, Y. Li and B. Xiao, Biomater. Sci., 2023, 11, 2486–2503 RSC.
- Y. Liang, X. Zhao, T. Hu, B. Chen, Z. Yin, P. X. Ma and B. Guo, Small, 2019, 15, 1900046 CrossRef PubMed.
- Y. Chen, Y. Gao, Y. Chen, L. Liu, A. Mo and Q. Peng, J. Controlled Release, 2020, 328, 251–262 CrossRef CAS PubMed.
- M.-C. Wu, A. R. Deokar, J.-H. Liao, P.-Y. Shih and Y.-C. Ling, ACS Nano, 2013, 7, 1281–1290 CrossRef CAS PubMed.
- W. Ge, S. Cao, F. Shen, Y. Wang, J. Ren and X. Wang, Carbohydr. Polym., 2019, 224, 115147 CrossRef CAS PubMed.
- A. Chinnathambi, S. Ali Alharbi, D. Joshi and H. Lenin, Bioinorg. Chem. Appl., 2022, 2022 DOI:10.1155/2022/9493816.
- F. Shahidi and Y. Zhong, J. Funct. Foods, 2015, 18, 757–781 CrossRef CAS.
- M.-A. Shahbazi, M. Hamidi, E. M. Mäkilä, H. Zhang, P. V. Almeida, M. Kaasalainen, J. J. Salonen, J. T. Hirvonen and H. A. Santos, Biomaterials, 2013, 34, 7776–7789 CrossRef CAS PubMed.
- X. Zhao, B. Guo, H. Wu, Y. Liang and P. X. Ma, Nat. Commun., 2018, 9, 2784 CrossRef PubMed.
- Q. Huang, T. Wu, Y. Guo, L. Wang, X. Yu, B. Zhu, L. Fan, J. H. Xin and H. Yu, Int. J. Biol. Macromol., 2023, 234, 123722 CrossRef CAS PubMed.
- Y. Zhang, Z.-L. Wang, Z.-P. Deng, Z.-L. Wang, F. Song and L.-L. Zhu, Carbohydr. Polym., 2023, 120973 CrossRef CAS PubMed.
- J. Zhou, Y. Liu, X. Liu, J. Wan, S. Zuo, T. Pan, Y. Liu, F. Sun, M. Gao and X. Yu, Carbohydr. Polym., 2023, 120924 CrossRef CAS PubMed.
- N. Ninan, A. Forget, V. P. Shastri, N. H. Voelcker and A. Blencowe, ACS Appl. Mater. Interfaces, 2016, 8, 28511–28521 CrossRef CAS PubMed.
- P. Atienza-Roca, D. C. Kieser, X. Cui, B. Bathish, Y. Ramaswamy, G. J. Hooper, A. N. Clarkson, J. Rnjak-Kovacina, P. J. Martens and L. M. Wise, Biomater. Sci., 2020, 8, 5005–5019 RSC.
- T. Wang, W. Yi, Y. Zhang, H. Wu, H. Fan, J. Zhao and S. Wang, Colloids Surf., B, 2023, 222, 113096 CrossRef CAS PubMed.
- Y. Zhang, Z.-L. Wang, Z.-P. Deng, Z.-L. Wang, F. Song and L.-L. Zhu, Adv. Polym. Technol., 2022, 2022, 1–11 CrossRef CAS.
- P. Zhang, Q. Xu, X. Li and Y. Wang, Mater. Sci. Eng., C, 2020, 108, 110396 CrossRef CAS PubMed.
- X. Zhang, D. Yao, W. Zhao, R. Zhang, B. Yu, G. Ma, Y. Li, D. Hao and F. J. Xu, Adv. Funct. Mater., 2021, 31, 2009258 CrossRef CAS.
- S. Li, Q. Dong, X. Peng, Y. Chen, H. Yang, W. Xu, Y. Zhao, P. Xiao and Y. Zhou, ACS Nano, 2022, 16, 11346–11359 CrossRef CAS PubMed.
- K. Lu, K. Li, M. Zhang, Z. Fang, P. Wu, L. Feng, K. Deng, C. Yu, Y. Deng and Y. Xiao, Chem. Eng. J., 2021, 424, 130429 CrossRef CAS.
- S. K. Papageorgiou, E. P. Kouvelos, E. P. Favvas, A. A. Sapalidis, G. E. Romanos and F. K. Katsaros, Carbohydr. Res., 2010, 345, 469–473 CrossRef CAS PubMed.
- S. Cao, X. Luo, X. Han, X. Lu and C. Zou, Energies, 2022, 15, 824 CrossRef CAS.
- J. Amirian, T. T. T. Van, S.-H. Bae, H.-I. Jung, H.-J. Choi, H.-D. Cho and B.-T. Lee, Int. J. Biol. Macromol., 2017, 105, 143–153 CrossRef CAS PubMed.
- H. Omidian, J. G. Rocca and K. Park, J. Controlled Release, 2005, 102, 3–12 CrossRef CAS PubMed.
- L. Yueqi, X. Jie, S. Ya, F. Huan, L. Jiaqi, L. Siyao, C. Y. Yee, N. Yi, L. Wenfang and P. Bo, Int. J. Bioprint., 2023, 9 DOI:10.18063/ijb.v9i1.689.
- J. Li and D. J. Mooney, Nat. Rev. Mater., 2016, 1, 1–17 CrossRef PubMed.
- Q. Tang, T. Lim, X.-J. Wei, Q.-Y. Wang, J.-C. Xu, L.-Y. Shen, Z.-Z. Zhu and C.-Q. Zhang, Biomaterials, 2020, 255, 120138 CrossRef CAS PubMed.
- I. Garcia-Orue, E. Santos-Vizcaino, P. Sanchez, F. B. Gutierrez, J. J. Aguirre, R. M. Hernandez and M. Igartua, Biomater. Adv., 2022, 135, 112695 CrossRef PubMed.
- C. Wang, C. Liang, R. Wang, X. Yao, P. Guo, W. Yuan, Y. Liu, Y. Song, Z. Li and X. Xie, Biomater. Sci., 2020, 8, 313–324 RSC.
- Y. Liang, Z. Li, Y. Huang, R. Yu and B. Guo, ACS Nano, 2021, 15, 7078–7093 CrossRef CAS PubMed.
- J. Chen, Y. Liu, G. Cheng, J. Guo, S. Du, J. Qiu, C. Wang, C. Li, X. Yang and T. Chen, Small, 2022, 18, 2201300 CrossRef CAS PubMed.
- B. Tao, C. Lin, Z. Yuan, Y. He, M. Chen, K. Li, J. Hu, Y. Yang, Z. Xia and K. Cai, Chem. Eng. J., 2021, 403, 126182 CrossRef CAS.
- X. Zhu, W. Feng, J. Chang, Y.-W. Tan, J. Li, M. Chen, Y. Sun and F. Li, Nat. Commun., 2016, 7, 10437 CrossRef CAS PubMed.
- J. Huo, Q. Jia, H. Huang, J. Zhang, P. Li, X. Dong and W. Huang, Chem. Soc. Rev., 2021, 50, 8762–8789 RSC.
- Q. Wei, Y. Wang, L. Jia, G. Ma, X. Shi, W. Zhang and Z. Hu, Biomater. Sci., 2023, 11, 170–180 RSC.
- M. Schäfer and S. Werner, Pharmacol. Res., 2008, 58, 165–171 CrossRef PubMed.
- Y. R. Girish, K. S. Sharathkumar, K. Prashantha, S. Rangappa and M. S. Sudhanva, Mater. Chem. Horiz., 2023 DOI:10.22128/MCH.2023.647.1037.
- J. Hu, L. Yang, P. Yang, S. Jiang, X. Liu and Y. Li, Biomater. Sci., 2020, 8, 4940–4950 RSC.
- F. Mollica, R. Lucernati and R. Amorati, J. Mater. Chem. B, 2021, 9, 9980–9988 RSC.
- L. He, G. Huang, H. Liu, C. Sang, X. Liu and T. Chen, Sci. Adv., 2020, 6, eaay9751 CrossRef CAS PubMed.
- V. Castrejón-Comas, C. Alemán and M. M. Pérez-Madrigal, Biomater. Sci., 2023, 11, 2266–2276 RSC.
- J. I. Paez, A. de Miguel-Jiménez, R. Valbuena-Mendoza, A. Rathore, M. Jin, A. Glaser, S. Pearson and A. Del Campo, Biomacromolecules, 2021, 22, 2874–2886 CrossRef CAS PubMed.
- M. Jin, G. Koçer and J. I. Paez, ACS Appl. Mater. Interfaces, 2022, 14, 5017–5032 CrossRef CAS PubMed.
- K. S. Lim, Y. Ramaswamy, J. J. Roberts, M. H. Alves, L. A. Poole-Warren and P. J. Martens, Macromol. Biosci., 2015, 15, 1423–1432 CrossRef CAS PubMed.
- Y. Liang, J. He and B. Guo, ACS Nano, 2021, 15, 12687–12722 CrossRef CAS PubMed.
- A. G. Goswami, S. Basu, F. Huda, J. Pant, A. Ghosh Kar, T. Banerjee and V. K. Shukla, Growth Factors, 2022, 40, 73–88 CrossRef CAS PubMed.
- M. K. Lichtman, M. Otero-Vinas and V. Falanga, Wound Repair Regen., 2016, 24, 215–222 CrossRef PubMed.
- Z. Qian, H. Wang, Y. Bai, Y. Wang, L. Tao, Y. Wei, Y. Fan, X. Guo and H. Liu, ACS Appl. Mater. Interfaces, 2020, 12, 55659–55674 CrossRef CAS PubMed.
- C.-C. Liang, A. Y. Park and J.-L. Guan, Nat. Protoc., 2007, 2, 329–333 CrossRef CAS PubMed.
- E. Aiba-Kojima, N. H. Tsuno, K. Inoue, D. Matsumoto, T. Shigeura, T. Sato, H. Suga, H. Kato, T. Nagase and K. Gonda, Wound Repair Regen., 2007, 15, 511–520 CrossRef PubMed.
- M. Simons, E. Gordon and L. Claesson-Welsh, Nat. Rev. Mol. Cell Biol., 2016, 17, 611–625 CrossRef CAS PubMed.
- Q. Zeng, Y. Qian, Y. Huang, F. Ding, X. Qi and J. Shen, Bioact. Mater., 2021, 6, 2647–2657 CrossRef CAS PubMed.
- J. Paez-Mayorga, S. Capuani, M. Farina, M. L. Lotito, J. A. Niles, H. F. Salazar, J. Rhudy, L. Esnaola, C. Y. X. Chua and F. Taraballi, Adv. Healthcare Mater., 2020, 9, 2000670 CrossRef CAS PubMed.
- Y. Liang, H. Xu, Z. Li, A. Zhangji and B. Guo, Nano-Micro Lett., 2022, 14, 185 CrossRef CAS PubMed.
- S. He, J. Fang, C. Zhong, F. Ren and M. Wang, Acta Biomater., 2022, 140, 149–162 CrossRef CAS PubMed.
|
This journal is © The Royal Society of Chemistry 2023 |