DOI:
10.1039/D0SC04082G
(Perspective)
Chem. Sci., 2020,
11, 12888-12917
Metallodrugs are unique: opportunities and challenges of discovery and development†
Received
26th July 2020
, Accepted 13th October 2020
First published on 12th November 2020
Abstract
Metals play vital roles in nutrients and medicines and provide chemical functionalities that are not accessible to purely organic compounds. At least 10 metals are essential for human life and about 46 other non-essential metals (including radionuclides) are also used in drug therapies and diagnostic agents. These include platinum drugs (in 50% of cancer chemotherapies), lithium (bipolar disorders), silver (antimicrobials), and bismuth (broad-spectrum antibiotics). While the quest for novel and better drugs is now as urgent as ever, drug discovery and development pipelines established for organic drugs and based on target identification and high-throughput screening of compound libraries are less effective when applied to metallodrugs. Metallodrugs are often prodrugs which undergo activation by ligand substitution or redox reactions, and are multi-targeting, all of which need to be considered when establishing structure–activity relationships. We focus on early-stage in vitro drug discovery, highlighting the challenges of evaluating anticancer, antimicrobial and antiviral metallo-pharmacophores in cultured cells, and identifying their targets. We highlight advances in the application of metal-specific techniques that can assist the preclinical development, including synchrotron X-ray spectro(micro)scopy, luminescence, and mass spectrometry-based methods, combined with proteomic and genomic (metallomic) approaches. A deeper understanding of the behavior of metals and metallodrugs in biological systems is not only key to the design of novel agents with unique mechanisms of action, but also to new understanding of clinically-established drugs.
1. Introduction
Metallodrugs have been used for centuries, but only now are methods and techniques becoming available to characterise the drugs precisely, identify their target sites, and elucidate their unique mechanisms of action.
The mixture of 3-amino-4-hydroxyphenyl-AsIII compounds known as Salvarsan (As1), containing acyclic As3 and As5 species,1 was introduced by Ehrlich in the early 20th century. Salvarsan was marketed by Hoechst as a treatment for syphilis and heralded the beginning of modern chemotherapy. More than half a century later, FDA approval in 1978 of cis-[PtIICl2(NH3)2] (cisplatin, Pt1) for the treatment of testicular cancer generated a huge surge of clinical interest in metallodrugs, and the birth of medicinal inorganic chemistry. Since then, a plethora of metallodrugs have been investigated as anticancer agents, antimicrobials, antivirals, and for many other indications.2 However, very few complexes have been successfully translated into the clinic. A histogram showing the number of metal and metalloid active ingredients in therapeutic drugs approved in the US and/or in Europe is reported in Fig. 1 together with a timeline of platinum drug development. The list of active ingredients is in Table S1.†
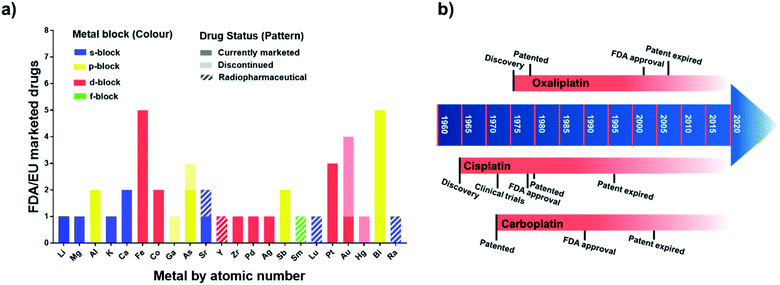 |
| Fig. 1 (a) Metallodrugs approved in US and/or EU countries classified by metal centre. Different formulations of the same active ingredient are not included, nor are pharmaceuticals where the metal represents only the counter ion. Imaging/diagnostic agents, food supplements, agents used as anaesthetics and in implants are also excluded. A comprehensive list of the agents included is in Table S1.† Remarkably, the total number of clinically-approved metallodrugs is about half of that for kinase inhibitors, a single class of organic drugs (see https://www.ppu.mrc.ac.uk/list-clinically-approved-kinase-inhibitors). (b) Timeline describing the development of anticancer drugs cisplatin (Pt1), carboplatin (Pt2) and oxaliplatin (Pt3). | |
Metal-based agents are also less investigated in preclinical screenings and, consequently, in clinical trials, suggesting that their potential remains largely untapped. An international antibiotic screening centre recently noted that although metal-containing agents represent a minority of compounds submitted, they display a 10× higher hit-rate towards ESKAPE pathogens than purely organic molecules.3 Metal compounds often do not follow the guidelines for drug-like properties of organic molecules. For example, Lipinski's rule of 5 predicts oral bioavailability of new agents,4 but includes a requirement of a MW < 500 Da, problematic for many third-row transition metal (TM) compounds,5e.g. the gold drug auranofin (Au1) (MW 678 Da). Molecular volume (MV) rather than MW has been proposed as an alternative for metallodrugs and utilised to build a library of metallofragments for drug discovery.6
Here we highlight some of the major challenges in translating metallodrugs into preclinical development. We will begin with the chemistry of metallodrugs, describing modes of activation, which often generate active pharmacophores, then move to in vitro screening, describing examples of complexes investigated for different indications and discussing the types of assay used to assess their potential for clinical translation. The identification of intracellular targets for metallodrugs is important for elucidating molecular mechanisms of action, but is a major challenge. While early research in the field has focused almost exclusively on DNA as a target for metallodrugs, we show here how, for example, new omics techniques allow identification of different target sites and hence mechanisms of action.
In the final section, we focus on analytical techniques, which can reveal metal speciation in solution and biological systems and track the distribution of metallodrugs in cells and in vivo, exploiting the cutting-edge metal-specific techniques now available. The chemical structures of selected metallodrugs are in Fig. 2, others (those marked with †) are given in Section 6 of the ESI.†
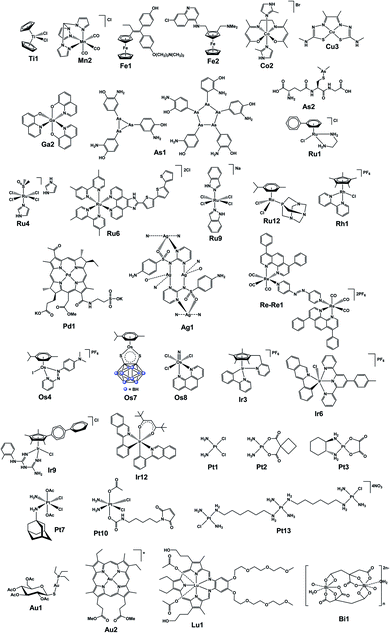 |
| Fig. 2 Structures of selected (candidate) metallodrugs. Others labelled in the text are in the ESI, Section 6.† | |
While this perspective focusses on metallodrugs and their interactions with biological molecules to produce a therapeutic effect, the targeting of metal-containing proteins, such as metalloenzymes, represents another important and complementary area of research. The role of metalloenzymes as medicinal targets and the use of metal-binding pharmacophores (MBPs) to inhibit them has recently been reviewed and will not be discussed here.7 Metallodrugs can also target metalloenzymes, either via incorporation of MBPs in their structure (e.g. as an axial ligand in PtIV agents)8 or directly through their metal centre (e.g. by replacing a metal ion in the active site).9 A few examples of metalloenzyme-targeted metallodrugs will be described.
2. Activation mechanisms
Metal-based therapeutics offer versatile electronic and structural features, including a range of oxidation states, coordination geometries, type and number of ligands. They offer novel chemistry, including different types of ligand substitution, metal- and ligand-based redox processes, and catalytic cycles. Unlike organic drugs, they are often ‘prodrugs’ which undergo activation en route to or at the target site. This typically involves the dissociation or displacement of one or more labile ligands, chelate ring-opening, or a change in oxidation state (or energetic state) of the metal and/or ligand(s). Alternatively, an external stimulus (e.g. light, radiation, sound, heat) can selectively activate metallodrugs at the target site.
2.1 Activation via hydrolysis
Hydrolysis is a common activation mechanism for TM drugs, involving the displacement of weakly bound σ-donor ligands by H2O. Marked differences in lability of ligands for different metal ions is illustrated by their aqua–ligand exchange rates, with time scales spanning 20 orders of magnitude (nanoseconds to years) from alkali metal ions to low-spin heavy TM ions.10 Such inertness of heavier TMs can be exploited in drug design. Importantly, an appropriate choice of ligands can modulate the ‘inertness’ of metal ions.
2.1.1 Square-planar PtII complexes.
Cisplatin (Pt1, cis-[PtIICl2(NH3)2]) undergoes activation via hydrolysis (Fig. 3).11 Extracellularly, where [Cl−] > 100 mM, hydrolysis is suppressed. Inside cells, where [Cl−] is lower (ca. 23 mM in cytoplasm and ∼4 mM in the nucleus),12 aquation occurs more readily, resulting in more reactive mono-aquated species, [PtII(OH2)Cl(NH3)2]+ and di-aquated [PtII(NH3)2(OH2)2]2+, which readily bind to DNA bases G and A, and also less reactive hydroxido species are formed (pKa values of [PtII(OH2)Cl(NH3)2]+ and [PtII(NH3)2(OH2)2]2+ca. 6.4, and 5.4/7.2, respectively).13 Only ca. 1% of intracellular cisplatin reacts with DNA,14 where it causes cell cycle arrest and apoptosis.15 ‘Soft’ PtII binds strongly to ‘soft’ cysteine thiolate groups in proteins.16 The tripeptide L-glutathione (γ-L-Glu-L-Cys-Gly, GSH, 2–10 mM in cells) can detoxify PtII, especially in resistant cancers.17 Such reactions can also result in off-target side-effects.11 Since hydrolysis rates depend on the ligands, a chelated dicarboxylate, relatively inert towards hydrolysis, was introduced in the second-generation drug carboplatin (Pt2), which displays reduced nephrotoxicity.18
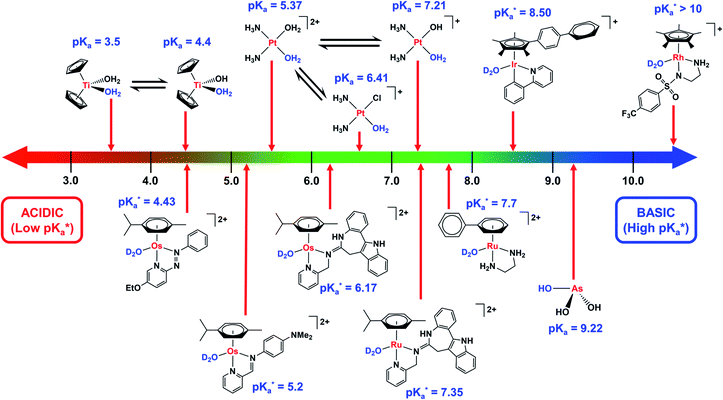 |
| Fig. 3 Comparison of the pKa values of aquated species of metal-based complexes. values refer to M–OD2 complexes based on pH* (pH meter reading not corrected for the effect of deuterium on the electrode). | |
2.1.2 TiIV dichloride complexes.
[TiIVCp2Cl2] (titanocene dichloride, Ti1) was in clinical anticancer trials for about 10 years,19 but its progression into phase II clinical trials was hampered by its strong tendency to hydrolyse,20 and pH-dependent interactions with DNA.21 Not only are the Cl− ligands labile but also Cp is displaced in reactions with serum transferrin, where TiIV binds to the FeIII sites, providing a delivery system for TiIV into cancer cells.22 To reduce hydrolysis rates, later titanocene derivatives included the addition of bulky aryl substituents, tethering Cp rings together, and even tethering Cp rings to labile groups to reduce ligand displacement (Ti2†).23
2.1.3 RuII and OsII half-sandwich complexes.
Similarly to cisplatin, half-sandwich pseudo-octahedral RuII and OsII η6-arene diamine anticancer complexes, [RuII/OsII(η6-arene)(N,N)Cl]+, such as RM175 (Ru1) also hydrolyse and bind to DNA, but monofunctionally as they have only one labile monodentate ligand.24 The choice of ligands plays a vital role in tuning the hydrolysis rate and reactivity. For RuII, hydrolysis rate decreases with the monodentate ligand in the order Cl ≈ Br > I > N3.25 Hydrolysis rate tends to increase with the electron donating ability of the arene, and the bidentate ligand has a strong effect on hydrolysis, increasing drastically when changing from neutral N- to anionic O-donors.12 Whereas aqua ligands are labile, hydroxido ligands are less so and prone to formation of bridged species. This can happen in culture media, giving inert hydroxo-bridged species in the case of [OsII(η6-p-cym)(acac)Cl] (Os1†), which are inactive towards cancer cells.26 OsII analogues of RuII arene chlorido complexes can hydrolyse up to 100× more slowly (e.g.Os2†vs.Ru1),26 and the aqua adducts of OsII arene paullone-based bidentate ligand complexes (e.g.Os3†) are 1.2 pKa units more acidic than their RuII counterparts (Ru2†).27
Strong σ-donor ligands (e.g. en, acac and pico) tend to promote fast hydrolysis and produce basic aqua adducts (pKa > 7). π-Acceptor ligands promote slower hydrolysis and more acidic aqua complexes (Fig. 3). OsII azopyridine (azpy) chlorido complexes can even be stable towards hydrolysis when heated under reflux with AgNO3.28 Complex FY26, [OsII(η6-p-cym)(azpy-NMe2)I]PF6 (Os4), is extremely stable and hydrolysis of the Os–I bond is negligible in aqueous media over 24 h.28131I-radiolabelling studies revealed that rapid cleavage of the Os–I bond is promoted in cancer cells.29 The mechanism appears to involve attack on the azo-bond by GSH (Fig. 4).29 This is accompanied by the generation of ROS.30 Iminopyridine (impy) ligands are similar in character to azpy, exhibiting weaker π-acceptor ability, and aqua adducts possess both DNA-binding and ROS-generating capabilities.31 Ang et al. screened 442 RuII arene impy complexes for their anticancer activity against A2780 ovarian cancer cells.32 All but one of the six lead complexes were stable towards hydrolysis and incorporate bulky substituents on their arene ligands and fused aromatic groups on their impy ligands (e.g.Ru3†).
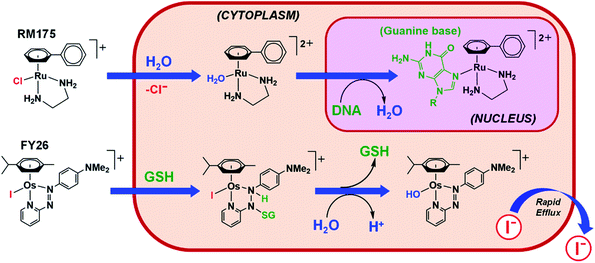 |
| Fig. 4 Hydrolytic activation of two types of half-sandwich complex; RM175 (Ru1), bearing a σ-donor bidentate ligand (en), for which hydrolysis is activated by reduced [Cl−] levels in cells; FY26 (Os4), bearing a strong π-acceptor ligand (azpy), for which hydrolysis is activated by GSH attack on the azo-bond.24,29 | |
2.1.4 RhIII and IrIII Cp complexes.
The residence times for water molecules in the first coordination shell of aqua RhIII and IrIII are extremely long (108 to 1010 s).33 The IrIII analogue of NAMI-A (Ir1†) is inert towards hydrolysis and exhibits poor antiproliferative activity.34 However, introduction of Cp* onto [IrIII(H2O)6]3+ dramatically increases hydrolysis rates by 14 orders of magnitude.35 As a consequence, a range of RhIII and IrIII CpX chlorido anticancer complexes with various N,N-, N,O-, O,O- and C,N-coordinated ligands all hydrolyse rapidly (minutes).36 Similarly to RuII and OsII arene complexes, RhIII exhibits higher pKa values for its aqua species than its heavier congener IrIII. For example, for [RhIII/IrIII(η5-Cp*)(en)H2O]2+ the RhIII complex is 1.7 pKa units less acidic.37,38 Interestingly, deprotonation of Cp* in complexes such as [RhIII(η5-Cp*)(bipy)(OH)]+ (aqua species of Rh1) can occur under physiological conditions to give RhI-fulvene species, which can undergo [4+2] cycloaddition Diels–Alder reactions with biologically relevant dienes (e.g. isoprene and (9Z,11E)-linoleic acid).39 Many chlorido complexes of IrIII Cp* with bipy, phpy, en, pico and acac bidentate ligands, hydrolyse rapidly and are anticancer inactive. However, introduction of extended arenes to Cp* (CpXphen and CpXbip) can reduce the hydrolysis rate, aqua-complex pKa, and switch on activity.40 Similarly, azpy ligands can also render IrIII Cp* complexes inert to hydrolysis, switching on their activity and promoting catalysis of GSH oxidation.41 Switching the monodentate ligand from Cl− to a neutral pyridine (py) significantly slows hydrolysis and the py ligand in [IrIII(Cpxbiph)(phpy)(py)]+ (Ir2†) increases anticancer activity 3-fold.42 Moreover, IrIII complexes with a Cp* ligand tethered to a ‘labile’ pyridine ligand (e.g.Ir3) are extremely potent and interestingly, appear to be activated via chelate ring opening in DMSO43 – a commonly used solvent in biological assays.
2.2 Redox activation
Oxygen is essential for energy production by eukaryotic organisms, but can also be lethal through generation of ROS.44 The disturbance of the redox balance is an effective anticancer strategy, owing to the distinct redox vulnerability of cancer cells, including hypoxia.45–47 Metal complexes can alter the cellular redox balance directly through reduction or oxidation at metal or ligand centres, or indirectly through interaction with biomolecules in redox pathways.47 Ideally, redox activation of metal complexes results in the formation of excess cytotoxic species selectively in cancer cells, thereby reducing side effects.45
2.2.1 Metal reduction.
Hypoxia can facilitate reduction and activation of inert metal complexes, e.g. PtIV, RuIII, CoIII, selectively in tumours.45 In normal, oxygenated cells, these prodrugs are reduced but rapidly re-oxidised and deactivated, whilst in hypoxic cancer cells, re-oxidation occurs slowly.48 Octahedral low-spin 5d6 PtIV complexes are more stable than classical PtII agents in biological media, and can be reduced by bio-reductants such as GSH, ascorbate, NAD(P)H, and cysteine-containing proteins, releasing active ligands and DNA-binding PtII species.45,49 Four PtIV complexes have entered clinical trials (i.e. tetraplatin (Pt4†), iproplatin (Pt5†), satraplatin (Pt6†) and LA-12 (Pt7†)), although none have been approved due to side effects and low efficacy.45,50 A RuII–PtIV conjugate (Ru-Pt1†) bridged by 1,6-diaminohexane undergoes PtIV reduction to release cytotoxic PtII, histone deacetylase inhibitor phenylbutyrate, and photosensitive RuII (480 or 595 nm irradiation), thus exerting a multi-targeting and multi-action effect.51 RuIII complexes NAMI-A (Ru4) and KP1019 (Ru5†) passed phase I clinical trials (NAMI-A was terminated in phase II due to low activity).52,53 The RuII complexes formed upon reduction retain an octahedral structure, and can bind to DNA and proteins.52 In contrast, for CoIII prodrugs cytotoxicity is often attributable to the ligands (e.g. nitrogen mustards) released upon reduction, rather than the metal itself.53
2.2.2 Ligand reduction.
Piano-stool RuII arene complexes [Ru(η6-bip)(azpy-NMe2)X]+ (X = I, OH) are activated by reductive addition of GSH to give GSSG, and elevate the ROS levels in cancer cells.54 The meso-carbon in the porphyrin ring in AuMesoIX (Au2) can undergo nucleophilic aromatic substitution with thiols, and modify cysteine thiols of cancer-associated proteins.55
2.2.3 Metal oxidation.
Ferrocene (Fc) derivatives can undergo Fenton-type reactions in cells, exploit the overproduction of H2O2 in cancer cells and generate HO˙ radicals which cleave DNA.56 Ferrocifen (Fe1), the ferrocenyl analogue of hydroxytamoxifen, is highly active towards breast cancer and independent of hormone expression.57 With the Fc+/Fc redox couple, Fe1 readily loses 2e− and 2H+ to form a quinone methide intermediate that is stable and cytotoxic. ROS are generated in cells treated with ferrocifens, but not hydroxytamoxifen derivatives. The anti-malarial agent Ferroquine (Fe2) also exerts cytotoxicity by formation of FeIII species and HO˙ radicals.58 Ferrocene N-heterocycle-linked RuIIp-cym complexes (e.g.Ru-Fe1†) show a correlation between the cytotoxicity and the Fc+/0 reduction potentials, consistent with facile oxidation to give ferrocenium, and subsequent ROS generation.59
2.2.4 Ligand oxidation.
The hydrolysis product [RuII(η6-bip)(en)H2O]2+ of the anticancer complex [RuII(η6-bip)(en)Cl]+ (Ru1) reacts initially with GSH to form [RuII(η6-bip)(en)(SG)]+.24 However, oxidation to the more labile sulfenato species, [RuII(η6-bip)(en)(OSG)]+, can occur even under hypoxic conditions, leading to facile displacement of the sulfenate by N7-cGMP. Such Ru-induced thiol oxidation (to sulfonate) can also occur in glutathione S-transferase, an enzyme overexpressed in solid tumours.60 The iodide ligand in photoactive trans-[PtIV(en)(OH)2I2] can be attacked by cellular bioreductants (e.g. GSH to generate GSSG) and liberate I−, with reduction of PtIV to PtII.61
2.3 Photoactivation
Metallodrugs can be selectively activated with high spatial resolution in cancer cells in (i) photodynamic therapy (PDT), (ii) photothermal therapy (PTT), and (iii) photoactivated chemotherapy (PACT).
PDT is clinically-approved, minimally invasive, and employs a photosensitiser (PS) and spatially-controlled light (typically red 600–800 nm, the “therapeutic window”) to kill cancer cells in a catalytic manner in the presence of oxygen.62 Longer wavelength light penetrates more deeply and is less toxic to cells. Metal complexes provide an alternative to organic PDT treatments, currently dominated by porphyrins and their analogues.63 The absorption of a photon promotes an electron from the singlet ground state to a singlet excited state which can decay non-radiatively via intersystem crossing to a low-lying triplet state. From this state, an electron can be transferred to biological substrates to form radicals that react with oxygen to generate ROS, causing oxidative stress, leading to cell death (type I). There can also be direct energy transfer from the PS excited triplet state to ground state triplet oxygen (3O2) to generate highly reactive singlet oxygen (1O2), with a diffusion distance <300 nm and lifetime <3 μs in cell nuclei (type II).64 Type II processes are dominant in tissues and clinical trials of a ruthenium-based PS TLD-1433 (Ru6), which mainly relies on type II processes, are ongoing. However, the only approved TM-based PS, TOOKAD®-soluble (palladium bacteriopheophorbide monolysine taurine, Pd1), exclusively undergoes type I photoreactions to generate hydroxyl and superoxide radicals.65 Selected metal-based photosensitisers are in Table 1. For compounds in clinical trials, their National Clinical Trial (NCT) identifier and details of the trials are freely accessible from the database https://www.clinicaltrials.gov/.
Table 1 Selected photoactivatable metal anticancer drugs and drug candidates classified according to their development phase
M |
Agent |
Activation |
Development stage |
Indication |
Ref. |
NCT retrieved from https://www.clinicaltrials.gov/
Not currently in clinical trials.
|
Clinically approved
|
AlIII |
Photosens® (Al1†) |
PDT |
Marketed in Russia |
Stomach, lung, oesophagus and other cancers |
66
|
PdII |
TOOKAD®-soluble (Pd1) |
PDT |
Marketed in Israel, Mexico, EU and EEA |
Localised prostate cancer |
67
|
![[thin space (1/6-em)]](https://www.rsc.org/images/entities/char_2009.gif) |
Clinical trials
|
RuII |
TLD-1433 (Ru6) |
PDT |
Phase II |
Non-Muscle Invasive Bladder Cancer (NMIBC) Refractory to BCG |
67
|
NCT03945162 |
LuIII |
Lutrin® (Lu1) |
PDT |
Phase I |
Locally recurrent prostate cancer |
66
|
NCT00005067b |
Au0 |
AuroLase™ |
PTT |
Multi-centre study |
Prostate cancer |
68
|
Au nanoshell, SiO2 core, PEG coat |
NCT04240639 |
![[thin space (1/6-em)]](https://www.rsc.org/images/entities/char_2009.gif) |
Pre-clinical
|
ReI |
Re-PLPG-NLS (Re1†) |
PACT |
— |
Cervical (HeLa), prostate (PC-3) cancer cell lines |
69
|
OsII |
TLD-1829 (Os5†) |
PDT |
— |
Bladder (HT1376), brain (U87) cancer cell lines |
70
|
IrIII |
Ir-HSA (Ir4†) |
PDT |
— |
Lung (A549), liver (HepG2) cancer cell lines |
71
|
PtII |
Pt-dithienylcyclopentenes (Pt-Pt1†) |
PACT |
— |
Lung (A549), melanoma (A375), breast (MCF7), colon (SW620), ovarian (SKOV3) cancer cell lines |
72
|
In PTT, metallodrugs convert photons to heat, leading to cell death. PTT agents are typically nanomaterials, including graphene oxide sheets and carbon nanotubes, as well as CuII nanocrystals, BiIII nanorods, and Au0 nanoparticles (AuNPs).73,74 AuroLase® is currently in clinical trials for neoplasms of the prostate (NCT04240639). AuNPs are irradiated with near-infrared radiation (750–1400 nm), causing localised surface plasmon resonance which loses energy via radiative and non-radiative processes, resulting in hyperthermic cell death.74 Tumour damage occurs at >41 °C, however temperatures exceeding 50 °C are required for effective ablation.73
In PACT, light activation of an inert prodrug leads to the formation of photoproducts, which contribute to the therapeutic effects.75,76 For example, PtIV azido complexes [PtIVL1L2(N3)2(OH)2] (L1, L2 = am(m)ine ligands) are reduced to cytotoxic PtII species upon visible light irradiation with concomitant release of azidyl radicals.77 A dithienylcyclopentene-PtII complex Pt-Pt1† exhibits increased cytotoxicity towards melanoma and colorectal cancer cells after photoswitching from its open to closed form.72 The oxygen-independent mechanism of PACT agents offers an advantage over PDT in hypoxic tumour environments. However, PACT agents are not catalytic, unlike PDT photosensitisers. This has led to the development of prodrugs which combine PDT with PACT, such as chlorin e6 conjugated PtIV micelles loaded on upconversion nanoparticles capable of producing oxygen upon photodecomposition, which is then self-utilised for PDT.78 Further examples of PACT agents are listed in Table 1. As yet, no metal-based PACT agents have entered clinical trials.
In principle, metal complexes which require activation by short wavelengths (e.g. blue light) can be activated using two photons of longer wavelength that penetrate more deeply (e.g. red/near-infrared light). This treatment can be highly specific (μm accuracy), however, the problem of rapid application to volumes as large as tumours has yet to be solved.79
2.4 Ionising radiation, sonodynamic and thermal activation
Ionising radiation (e.g. X-rays, γ-rays) can also activate or enhance the potency of metallodrugs. A radiosensitiser is a compound whose combination with ionising radiation generates a biological response greater than the sum of their single effects. This synergy was noted early in the clinical use of platinum drugs, which are now frequently combined with external beam radiotherapy.80 The mechanisms of radiosensitisation include enhancement of damage generated by X-ray radiation or inhibition of repair and resistance mechanisms.80 Several TM-based radiosensitisers (mainly groups 6–8) have been investigated preclinically (Table 2). Most recently, a cyclometallated IrIII compound (Ir5†) was reported to localise in mitochondria and to produce increased radiosensitisation in cancer cells compared to cisplatin, attributable to increased ROS production upon X-ray irradiation.81
Table 2 Selected examples of metal-based agents activated by external stimuli
M |
Type |
Activation |
Ref. |
RuII |
Arene RuII complex (Ru7†) |
Radiosensitiser |
92
|
IrIII |
Cyclometallated IrIII complex (Ir5†) |
Radiosensitiser |
81
|
CoII |
CoII porphyrin (Co1†) |
XPDT |
93
|
Cu |
Copper cysteamine nanoparticles |
Scintillation XPDT |
84
|
TiIV |
Hydrophilised TiO2 nanoparticles |
Sonodynamic |
94
|
GaIII |
Gallium porphyrin-antiCEA antibody (Ga1†) |
Sonodynamic |
95
|
RuII |
Arene RuII complex with perfluorinated ligand (Ru8†) |
Hyperthermia |
89
|
Synchrotron stereotactic radiotherapy (SSR) is a type of external-beam irradiation, which exploits high-fluence monochromatic X-ray beams to treat cancer. The presence of heavy elements can enhance the therapeutic effect if the X-ray beam is tuned to the energy of the K-electrons of the sensitiser. Promising results were obtained for brain tumours in rats with SSR and cisplatin, although a similar effect was achieved with traditional linear accelerators.82
X-ray PDT (XPDT), resulting in the excitation of a photosensitiser and generation of singlet oxygen, can be achieved by direct PS excitation with X-rays or indirectly, by X-ray excitation of a luminescent material (scintillator), which in turn can activate the PS.83 Metalloporphyrins have been investigated for the direct approach,83 while inorganic-based nanoparticles have been explored for the indirect approach.84 The main advantage of XPDT is the ability to overcome the limited penetration depth of visible light.85
Moving from electromagnetic to mechanical waves, sonodynamic therapy was initially developed as a proxy for PDT to increase tissue penetration depth. Sonoluminescence, obtained by concentrating large amounts of energy from ultrasound, can excite a PS.86 Porphyrins and metalloporphyrins have shown promising results in vitro, and metal-based nanoparticles and metal–organic frameworks have been explored (Table 2).87,88
Metallodrugs can be selectively activated by heat, e.g. by conjugation to thermoactivatable fragments. For example, the solubility and cytotoxicity of RAPTA-derivatives containing perfluorinated ligands (Ru8†) are enhanced by heating (Table 2).89 Metalloenediynes (e.g.Pt8†) are thermally-activated sources of the enediyne function, whose spontaneous cyclisation produces extremely potent 1,4-benzenoid diradical species.90,91
2.5 Catalytic metallodrugs
TM catalysts might offer low-dose agents that can carry out biocatalytic reactions on endogenous or exogenous substrates.96 Potential catalytic reactions include transfer hydrogenation, C–C bond cross-coupling, bond cleavage by hydrolysis or oxidation, azide–alkyne cycloaddition, and allyl carbamate cleavage.97 Successful catalysts in living systems require high conversion rates under physiological conditions, good enantioselectivity, and tolerance towards aqueous environments and nucleophilic poisoning.
2.5.1 Reduction via transfer hydrogenation.
Reduction of biomolecules in cancer cells by external catalysts can disrupt metabolic cellular processes crucial for cell survival. Nicotinamide adenine dinucleotide (NAD+) and reduced NADH are key cofactors for many reactions in cells involving redox homeostasis.98 A lower ratio of NAD+/NADH in the cytosol is observed in cancer cells compared to normal cells.99 Transfer hydrogenation reactions of NAD+/NADH have been achieved in cells using “piano-stool” metal complexes including IrIII Cp* and RhIII Cp* complexes, as well as RuII arene complexes.100–102 Formate can act as a hydride source at non-toxic concentrations.96 The catalytic mechanism involves initial formation of M-formate adducts, transfer of hydride to the metal and release of CO2 followed by hydride transfer to NAD+.101 Noyori-type RuII arene complexes with sulfonamido ethylenediamines as ligands show a 50-fold increase in cytotoxicity towards human ovarian cancer cells in the presence of formate. This type of catalysis increases the reductant pool and induces non-apoptotic cell death.103
In hypoxic cancer cells, pyruvate is reduced to lactate by lactate dehydrogenase and NADH. Chiral catalytic 16-electron OsII complex Os6† can convert pyruvate to unnatural D-lactate enantioselectively in the presence of formate, and selectively for cancer cells over healthy cells.104
2.5.3 Degradation and cleavage of biomacromolecules.
Strong Lewis acidic metal complexes can be designed for hydrolytic and oxidative cleavage. Metal complexes with tetra-N-methylated cyclam (TMC) ligands can cleave 36–43 amino acid Aβ peptides involved in Alzheimer's disease, with catalytic efficacy: CoII > ZnII > CuII > NiII.109 Amino-terminal copper/nickel (ATCUN) peptide binding motifs such as CuII-GGHK-R (Cu1†, where R is a target recognition sequence) can catalyse the cleavage of viral RNA and G-quadruplex telomeric DNA,110,111 in which the redox-active CuII/CuIII generates ROS to cleave bonds.
3.
In vitro screening
Nowadays, discovery of new organic drugs typically begins with the choice of a protein or enzyme target, followed by target validation, assay development, high throughput screening, hit identification, lead optimisation, and selection of a candidate for clinical development. Rational design of metal complexes as enzyme inhibitors is feasible, as elegantly demonstrated by the work of Meggers, designing kinase inhibitors based on inert octahedral Ru(II) scaffold.112,113
However, metal complexes are usually prodrugs transformed into active species by ligand exchange or redox reactions, and are likely to be multi-targeting. Hence, phenotypic screening (the ability of a molecule to alter a cell's phenotype -an observable characteristic) is usually the method of choice for discovery of metallodrugs. Phenotypic screening offers the potential advantage of revealing truly novel mechanisms of action.114
We discuss some promising metallodrug candidates under investigation for the treatment of cancer, inflammation and infections. For each indication we describe the main methods used for in vitro screening and highlight specific challenges. Nearly all the assays described below involve the culture of cells in a growth medium. Knowledge of the chemical composition of biological media is crucial for understanding the speciation of test metal complexes in screening assays. Metallo-prodrugs may be converted into different species before they interact with the cells during the screening.
3.1 Metallodrug speciation in biological assays
3.1.1 Solubilising agents.
Use of dimethylsulfoxide (DMSO) for solubilising drugs for cell testing is universal, but poses particular problems for TM complexes. DMSO can act as a S- or O-donor ligand, resulting in ligand substitution reactions.115 Even 0.5% v/v DMSO in culture medium is equivalent to 70 mM DMSO. Reactions with DMSO are temperature and time-dependent and the concentration of DMSO may vary between wells in a multi-well culture plate as the stock solution is diluted. For example, DMSO can have a significant effect on the cytotoxicity of platinum anticancer complexes such as cisplatin (vide infra). S-Bound DMSO with its high trans effect readily induces chloride and ammonia loss.116
3.1.2 Culture media.
Growth media contain many components (Tables S2–S4†), which can react with metal complexes (ligand substitution, redox) and transform them into new species (Tables S2–S4†).
Chemically-defined media include RPMI-1640, DMEM, MEM, and many others. RPMI-1640 (Table S2†) typically contains 20 amino acids (at concentrations ranging from 25–318 μM), all strong metal chelators, either bidentate, or tridentate e.g. 150 μM L-Asp, 136 μM L-Glu, 97 μM L-His, and 101 μM L-Met, a thioether. Other soft donors include the only thiol GSH (3.25 μM); Cys is present as the disulfide cystine.
These defined media are usually supplemented with e.g. 10% v/v foetal calf/bovine serum (FCS/FBS) or similar products (e.g. beef extracts for microbial culture), together with antibiotics such as penicillin (a thioether, typically at ca. 170 μM), which further complicates metal speciation. The compositions of these animal-derived products will differ between different batches, suppliers, and regions of the world. Typically, FBS contains ca. 0.3 mM bovine serum albumin (BSA) and 1 mM gamma globulin (Table S3†). Albumin has a range of drug-binding sites and may deactivate drugs, but it also may deliver metallodrugs to cells, even crossing cell membranes.71,115 The thiol content of albumin decreases with age (oxidation of Cys34).117 Albumin contains CuII and ZnII binding sites. The FBS concentration of transferrin, which binds FeIII and a variety of other metal ions strongly, is not always specified.115,118
Detailed metal speciation in these media is very complicated and depends not only on the type of medium used, but also on pH, aeration (CO2/O2), temperature and time. Reactions at low concentrations (e.g. micromolar), may be very slow. However, cell screens typically last from hours to 4 days at 37 °C, sometimes with constant exposure to the metal complex.
3.1.3 Analytical techniques for speciation.
Some analytical techniques have metal-specific features useful for characterising metallodrugs and their metabolites. Examples and the challenges associated with them are summarised in Table S5.† Combined application of several techniques is usually necessary. NMR, EPR, vibrational, UV-vis and CD spectroscopy, as well as MS are discussed briefly in the ESI.† An example of how protein platination can be characterised by MS is shown in Fig. 5.119 Such MS studies often allow identification of specific binding sites and the nature of binding to the protein, including the coordination sphere of platinum which will influence the subsequent biological behaviour of the platinated protein.
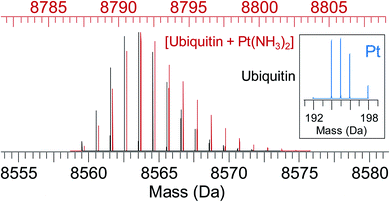 |
| Fig. 5 Simulated isotope pattern for 8.6 kDa protein ubiquitin (black) and [ubiquitin + Pt(NH3)2] (red, shifted by 228 Da, monoisotopic mass of Pt(NH3)2, for ease of comparison). Due to the characteristic isotope pattern of Pt (inset), the isotopic distribution of the protein–metal complex is broader and shifted to higher mass compared to the apo-protein. Automated peak-picking procedures can identify such platinated species in complex mixtures (e.g. cell lysates) providing insight into the binding sites for metallodrugs on proteins.119 | |
3.1.4 HPLC.
Reversed-phase HPLC, including nano-HPLC, can separate a variety of metal species present in biological media. However, care is needed with UV-vis quantification since extinction coefficients change with species and wavelength.24 Fluorescence and gamma/beta ray detection can be very sensitive while LC-MS (vide infra) allows direct identification of chemical species. RP-HPLC analyses often require use of acidic ion-pairing agents (e.g. trifluoroacetic acid) to improve peak sharpness for ionic complexes. Nonetheless, such additives lower the pH of the mobile phase, and may alter the speciation of metallodrug metabolites. For example, organo-OsII hydroxido complexes (Fig. 3) stable at physiological pH exist predominantly as more labile Os–OH2 species under acidic HPLC conditions.29 Furthermore, adducts with trifluoroacetate may form, e.g. for various RuII and PtIV anticancer complexes.120,121
Size exclusion chromatography-inductively coupled plasma-mass spectrometry (SEC-ICP-MS), combines the metabolite-separating ability of SEC with element quantification by ICP-MS,122e.g. for time-dependent binding studies of RuII and OsII anticancer agents to serum proteins in vivo.123
3.2 Anticancer agents
The platinum anticancer drugs cisplatin (Pt1) (FDA approval 1978), carboplatin (Pt2) (1986) and oxaliplatin (Pt3) (1996) are now used in ca. 50% of cancer chemotherapies. Current emphasis is on new complexes which might increase the spectrum of treatable cancers, reduce side-effects, and overcome platinum resistance.124 Comprehensive reviews on metallo-anticancer drugs are available.125–128 Selected examples of metallodrugs in clinical trials as anticancer agents are in Table 3. Although the number and variety of compounds in clinical evaluation well reflects the growing interest in this field, it must be noted that the success rate of such trials is generally quite low (ca. 10%) and even lower for oncology.129
Table 3 Examples of metal-based anticancer drugs that have entered clinical trials, with their stage in the clinical pipeline, administration route, treatment indication and clinical trial identifier. For structures see Fig. 2 and Section 6 of the ESI
M |
Agent |
Stage |
Administration |
Treatment |
Triala |
Ref. |
NCT retrieved from https://www.clinicaltrials.gov/.
Administered as a free ligand. Active as metal complex (Zn/Cu). Complex transmetalated with Cu in lysosomes.138
Combination: cabazitaxel, docetaxel, mitoxantrone or satraplatin (CDMS) plus surgery.
From the European Union Drug Regulating Authorities Clinical Trials Database.
Immunosuppressive.
|
Zn/Cu |
DpC (thiosemicarbazone)b (Zn1†, Zn2†) |
Phase I |
Oral |
Advanced solid tumours |
NCT02688101 |
130
|
As |
Darinaparsin (As2) |
Phase I |
Oral |
Advanced solid tumours |
NCT01139346 |
131
|
Ru |
NKP1339 (Ru9) |
Phase I |
Intravenous |
Colorectal carcinoma, non-small cell lung cancer and gastrointestinal neuroendocrine tumours |
NCT01415297 |
132
|
Ru |
TLD1433 (Ru6) |
Phase II |
Intravescical photodynamic therapy (PDT) |
Photodynamic therapy for non-muscle invasive bladder cancer |
NCT03945162 |
133
|
Pt |
Satraplatin (Pt6†) |
Phase I |
Oral (CDMS)c |
Prostate cancer |
NCT03258320 |
124
|
Refractory solid tumours including brain tumours |
NCT01259479 |
134
|
Pt |
Nanoplatin™ (NC6004) |
Phase II |
Infusion therapy (+pembrolizumab) |
Recurrent or metastatic squamous cell carcinoma of the head and neck |
NCT03771820 |
124 and 135
|
Phase I/II |
(+Gemcitabine) |
Pancreatic cancer |
NCT00910741 |
|
Pt |
Picoplatin (Pt9†) |
Phase I/II |
Infusion therapy (+leucovorin and 5-fluorouracil) |
Colorectal cancer |
NCT00478946 |
|
Phase I |
Oral and intravenous |
Solid tumours |
NCT00465725 |
124 and 135
|
Pt |
BTP-114 (cisplatin pro-drug) (Pt10) |
Phase I |
Intravenous |
Pancreatic, ovarian, breast and prostate neoplasms (BRCA mutations) |
NCT02950064 |
135
|
Pt |
PT-112 (Pt11†) |
Phase I |
Intravenous |
Relapsed or refractory multiple myeloma |
NCT03288480 |
|
Phase I |
Advanced solid tumours |
NCT02266745 |
135
|
Pt |
ProLindac™ (Pt12†) |
Phase I |
Intravenous |
Unresectable and metastatic head and neck cancer |
NCT00415298 |
135
|
Phase II |
Advanced ovarian cancer |
EudraCT: 2010-020030-25d |
136
|
Pt |
Triplatin (Pt13) |
Phase II |
Intravenous |
Inoperable small cell lung cancer, Advanced/metastatic adenocarcinoma of the pancreas |
NCT00014547, NCT00024362 |
137
|
Au |
Auranofin (Au1) |
Phase I/II (+sirolimus)e |
Oral |
Advanced or recurrent non-small cell lung cancer or small cell lung cancer |
NCT01737502 |
|
Phase II |
Chronic lymphocytic leukaemia, small lymphocytic/prolymphocytic lymphoma |
NCT01419691 |
135
|
3.2.1 Cytotoxicity assays.
In vitro evaluation relies on the assessment of the viability of 2D monolayer cultures of human cancer cells, generating dose-response curves to estimate cytotoxicity in terms of half-inhibitory concentrations (IC50). Some of the most commonly used cytotoxicity assays for metallodrugs are summarised in the following paragraphs. However, it should be noted that a low IC50 value is not necessarily predictive of successful preclinical and clinical translation of a metallodrug. Other factors to consider are off-target effects as well as bioavailability/pharmacokinetics in animals, as briefly described in Section 3.7. Importantly, novel in vitro models (e.g. spheroids and organoids) are also becoming available to better mimic in vivo conditions, and will be described in Section 3.2.2.
The colorimetric 3-(4,5-dimethyl-2-thiazolyl)-2,5-diphenyl-2H-tetrazolium bromide (MTT) assay is a gold standard. It measures the enzymatic reduction of tetrazolium to insoluble formazan crystals by dehydrogenases in cell organelles including mitochondria and endoplasmic reticulum. The assay is sensitive and can be miniaturised for high-throughput screening. However, it requires formazan solubilisation by DMSO, and formazan crystal conversion is dependent on metabolic function.139 It is also important to note that the reduction to formazan is catalysed by mitochondrial enzymes, potentially distorting the results of the MTT assay for complexes targeting mitochondria. For these types of complexes, including polypyridyl Ru complexes and the organo-OsII azopyridines, alternative cytotoxicity tests are required.140–142
The Cell Counting Kit-8 tetrazolium-8-[2-(2-methoxy-4-nitrophenyl)-3-(4-nitrophenyl)-5-(2,4-disulfophenyl)-2H-tetrazolium] monosodium salt (CCK-8) is a colorimetric assay similar to MTT, that converts highly water-soluble tetrazolium salt WST-8 to a water-soluble orange-coloured formazan, not requiring DMSO. A limitation of colorimetric assays, is that spectroscopic interference needs to be considered when using coloured drugs.143
The Sulforhodamine B (SRB) assay determines protein content by binding stoichiometrically to proteins under acidic conditions, presenting no compound interference. This assay requires several wash steps and is less sensitive for poorly adherent cells.139 Neutral red uptake (NRU) assay determines cell viability by incorporating neutral red dye into lysosomes. For example, the NRU assay was used to determine the cytotoxic activity of two α-amino acid Schiff base-derived Ru and Pt complexes, altering lysosomal function in HepG2 cells, in accordance with MTT data.144 The lactate dehydrogenase (LDH) leakage assay quantifies LDH activity within the extracellular medium. NRU and MTT assays have increased cytotoxic sensitivity compared to the LDH leakage assay for hepatoma cells exposed to CdCl2.145 In particular, the MTT assay detected toxicity before the NRU and LDH assays because CdCl2 is likely to have an impact on the mitochondria in HepG2 cells, followed by lysosomal damage and only then LDH leakage.145 The resazurin reduction assay (RES) converts resazurin to fluorescent resorufin typically within the mitochondria, thus measuring metabolic activity.139 Similar to MTT, RES can produce false responses due to interfering chemical functional groups (e.g. thiols, carboxylic acids). However, MTT displayed a higher number of interferences compared to RES when tested with different concentrations of 19 potentially interfering substances (such as GSH).146
Clonogenic assays are useful to evaluate the long-term effects of a cytotoxic agent, by determining the number of cells able to undergo cell division and form a colony of at least 50 cells after treatment. Most commonly, crystal violet is used to stain the cells prior to counting of colonies.147
The type of assay used, the time of drug incubation, and the extension of cell recovery time after drug removal, can all significantly influence the results of the cytotoxicity screening. Such screens are usually carried out on unsynchronised cells, although the stage of the cell cycle can have an important effect (vide infra).
Care has to be taken when comparing cytotoxicity data reported in the literature even for the same complex in the same cell lines, as illustrated in Fig. 6. We compare the contrasting cytotoxicity data reported by different labs for the same complex (cisplatin) in the same two cell lines. The evident differences in cytotoxicity are particularly striking as cisplatin is often used as a positive control in assessment of the potency of metallodrugs. In this case, the differences are likely related to the differences in the various screening conditions as noted in Table S6† (solvent for compound dissolution, culture medium, treatment time, type of assay).
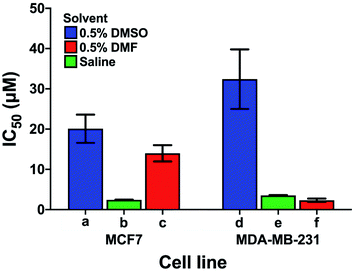 |
| Fig. 6 Examples of the large differences in IC50 values for cisplatin reported by 3 different labs (bars a, d; b, e and c, f) for the same cell lines (MCF7, human breast cancer, and MDA-MB-231, epithelial human breast cancer). The effect of the solvent used to solubilise cisplatin is highlighted; other differences in the screening conditions including type of assay, time of treatment, and composition of culture media are listed in Table S6.† | |
Longer treatment time usually leads to a lower IC50 value. The main influence on the IC50 values is probably due to the use of DMSO for solubilisation of cisplatin with the lowest IC50 values in Fig. 6 resulting from DMSO-free testing. DMSO is used as a standard solvent to ensure solubilisation of compounds for testing (even when not needed). Such effects of DMSO have been previously described by Hall et al.116 who, already 5 years ago, concluded that “practice of dissolving platinum drugs in DMSO must cease, and if the solvent is to be utilised, new platinum agents must demonstrate a lack of interaction with DMSO”.
In general, these data highlight the need for a standardised protocol to compare results from different laboratories, especially for positive controls, which ensure reliability of the data by confirming the identity and proliferation ability of cells. Such protocols should be relevant to clinical translation, which generally involves shorter drug exposure and clearance before a period without the presence of the drug. A possible standardisation that could be implemented across the field for the cisplatin control might be: solubilisation in saline, 24 h cisplatin treatment, and 72 h recovery in cisplatin-free medium prior to the chosen cytotoxicity assay.
The National Cancer Institute screen (NCI-60) determines the effect of compounds on cell viability in a panel of 60 cancer cell lines (breast, prostate, renal, ovarian, melanoma, CNS, colon, non-small lung, leukaemia). The COMPARE programme can classify unknown mechanisms of action (MoAs) for compounds by assessing the similarity between mean graph patterns of IC50 values.148
The Cancer Genome Project in the Wellcome Sanger Institute provides screening similar to NCI-60, but covers ca. 900 cell lines, all with known genomic DNA sequences. It produces GI50 values (concentration of drug for 50% of maximal inhibition of cell proliferation) to evaluate cellular drug sensitivities. Combined with the “Catalogue Of Somatic Mutations In Cancer” (COSMIC), genomic data of cancer mutations in different cell lines, it can be used to elucidate the MoAs of anticancer drugs. For example, the Sanger screen of half-sandwich organo-IrIII complex ZL109 (Ir7†) revealed higher anticancer potency than cisplatin, and similarity to osmium azopyridine complexes and natural anticancer product piperlongumine.149 This suggested that the MoA involves rapid generation of ROS.149
3.2.2 Beyond 2D cell cultures.
Promising antiproliferative activity in 2D cell cultures in vitro often does not translate effectively into in vivo models. 3D multicellular tumour spheroids – spherical self-assembled aggregates of cancer cells, which allow signalling between cells in close contact - represent a more relevant model.150 The activity of Pt (cisplatin: Pt1, oxaliplatin: Pt3 and KP1537: Pt14†), Ru (NKP1339: Ru9), Ga (KP46: Ga2) and La (KP772: La1†) compounds has been compared in 3D spheroids and 2D monolayers, plus invasion and metastasis models. The IC50 values of Ga2 in all three tested cell lines were in the low micromolar range, whereas, for spheroids, the cytotoxicity was reduced by ca. 300-fold.151 This lower cytotoxicity is likely a consequence of multicellular interactions, reduced drug penetration, and possibly hypoxia in the spheroids. Overall, such data highlight the need for a combination of models for screening of compounds.151
Organoids, miniaturised organs produced from stem cells in vitro, are available as 3D culture models. However, there are limitations such as reproducibility as well as inefficient waste and nutrient transport. Microfluidic organs-on-a-chip are potential alternatives. They replicate the features of human organs by providing fine control over the microenvironment and transport via fluid flow, with the potential to be scaled up for high-throughput screening.152
3.3 Anti-inflammatory agents
The AuI phosphine anti-inflammatory drug auranofin (Au1) is used in the treatment of rheumatoid arthritis. Thioredoxin reductase, with its thiol-disulfide catalytic site and selenocysteine residue, is a cellular target for auranofin which inhibits the oxidoreductase pathway implicated in inflammatory responses through the production of pro-inflammatory oxidants.153 However, due to severe side effects with long-term use, auranofin is not used as a first-line treatment.
Non-steroidal anti-inflammatory drugs complexed as ligands to metals may provide alternative treatments, including anticancer. This can be rationalised as inflammation is a hallmark of cancer. Examples are CuII-acemetacin and ZnII-indomethacin which inhibit acute arterial inflammation in in vivo rabbit studies analysing VCAM-1 and ICAM-1 expression.154 Half-sandwich electron-deficient OsII, IrIII and RuII carborane complexes (Os7, Ir8†, Ru10†) inhibit lipopolysaccharide-induced nitric oxide production, a known anti-inflammatory response, without cytotoxicity.155
In vitro screening techniques can be used to assess anti-inflammatory activity. Whole human blood can be stimulated with lipopolysaccharide to induce inflammation and inflammatory responses (e.g. INFγ, TNFα, interleukins) monitored.156 Assays using purified human peripheral blood mononuclear cells can also be used to screen test compounds with the same inflammatory response readout, but offer a protein-free model.156
3.4 Antimicrobial agents
The ancient Greeks and Romans discovered the antimicrobial properties of silver and used silver containers to preserve food and water.157 More recently silver sulfadiazine (Ag1) was approved as a topical cream to prevent bacterial infections in burns.3 Some bismuth compounds are effective in combination treatment of Helicobacter pylori infections.3,158 Tribromophenate, bismuth (Xeroform®) is used as an antibiotic in dressings, but appears to prevent bacteria entering wounds rather than have antimicrobial activity. Metallodrugs are frequently overlooked as potential antibiotics, with none currently in clinical trials. A recent study found that the metal complexes have a significantly higher hit-rate against critical bacterial and fungal pathogens compared to organic compounds.3Ir9 was one of the most active compounds in the study across three strains of Gram-negative and one Gram-positive bacteria, as well as two fungi.3
Many important antibiotics such as penicillins, cephalosporins, and cephamycins are based on β-lactams, which can be degraded by zinc metallo-β-lactamases (MBLs) – enzymes that cause multi-drug resistance. However, bismuth (from colloidal bismuth subcitrate) can displace zinc in New Delhi metallo-β-lactamases (NDMs, a subclass of MBLs), thereby inactivating them and improving in vivo efficacy of β-lactams.9 In addition, a recent screening of a metallofragment library against selected enzyme targets showed that some ferrocene-based compounds effectively inhibit NDM-1 and warrant further exploration as scaffolds for new NDM inhibitors.6
Metals containing bioactive organic ligands can also act as antimicrobial agents. This approach can enhance the activity of antimicrobial ligands, especially towards drug-resistant strains. For example, CuII, CoII and NiII complexes containing S-benzyldithiocarbazate Schiff-base ligands (M1†) display remarkable antifungal activity against a wide spectrum of fluconazole-susceptible and -resistant Candida albicans isolates.159 Notably, these complexes are up to 1000× more active than their corresponding Schiff-base ligands and cause apoptosis via inhibition of ergosterol biosynthesis and membrane disruption. However, more mechanistic studies are required with these ligands to allow more detailed comparisons of their mechanisms of action.160 Metal-quinoline antibiotics can exhibit good antifungal activity, and 1,10-phenanthroline conjugated to MnII, AgI and CuII inhibit growth of drug-resistant biofilms and planktonic fungi species that lead to the Candida haemulonii complex. Their mechanisms of action may include DNA and protein cleavage, mitochondrial disruption and interference with protein synthesis.161,162
In vitro screening of antibacterial and antifungal metallodrugs often follows the same methods used for the assessment of organic compounds; such as disk diffusion, gradient diffusion and broth dilution methods, and flow cytometry has been used to study cell membrane integrity, distinguishing between dead, viable and damaged cells.163 Some of these techniques have been subject to standardisation by the CLSI and EUCAST.163 However, interference caused by absorption or fluorescence of metal complexes can cause problems in these assays.
Unlike eukaryotic cytotoxicity screening, the end point of antimicrobial assays is often visual and evaluated as minimum inhibitory concentration (MIC), the lowest concentration needed to prevent visible bacterial growth, and minimum bacteriocidal concentration (MBC), the lowest concentration to achieve a biocidal effect, e.g. 99.5% over 18 h as colony-forming units CFU mL−1. Both are reported in μg mL−1, requiring conversion of molar units for direct comparisons.
In vitro screening of antiparasitic compounds is not well standardised, except in some cases, e.g. malaria.164 Screening strategies have been reviewed.165 Ferroquine, a ferrocene–chloroquine conjugate (Fe2), is active at nanomolar concentrations against chloroquine-susceptible and resistant strains of P. falciparum.166Fe2 has recently completed a phase II clinical trial in combination with artefenomel (trial NCT03660839). Auranofin (Au1) has also been repurposed as an antiparasitic and is in phase IIa clinical trials for the treatment of amoebic dysentery (amoebiasis) and giardiasis, caused by E. histolytica and G. lamblia, respectively (NCT02736968). Auranofin was the most active in a high throughput screen of 910 compounds, with an EC50 10-fold lower than the currently used metronidazole.167 Research on metal-based antiparasitics has been reviewed recently.168
In general, medicinal inorganic chemistry has the potential to overcome some of the major challenges in antimicrobial research including antibiotic resistance. However, research on novel metal-based antimicrobial agents does not typically extend beyond their synthesis plus MICs, MBCs and haemolysis assay evaluation and, if these are promising, the design of analogues. Whilst this research is necessary, more follow-up studies should be conducted to unravel the mechanisms of action of these metallodrugs and test their efficacy/toxicology in vivo. Only a more translationally-focussed approach can determine whether such compounds are indeed worthy of preclinical development, and if so, move them down the pipeline towards clinical use.
3.5 Antiviral agents
There is increasing interest in the antiviral activity of metal compounds, although no metallodrugs are currently used clinically. Antiviral assays fall into two distinct groups: (i) direct detection of virus population, and (ii) assessment of cell survival as a result of viral infection. Direct detection methods include plaque assays, enzyme-linked immunosorbent assays (ELISA) and quantitative real-time polymerase chain reaction (qRT-PCR). A more detailed description is in the ESI.† Methods for cell viability are discussed above (e.g. MTT, SRB). Selected examples of antiviral metal compounds are summarised in Table 4.
Table 4 Examples of antiviral metal compounds
Compound |
Virus |
Assay |
Ref. |
Polyoxometalates, Cs2K4Na[SiW9Nb3O40], Cs2K4Na[SiW9Nb3O40] (POM93) |
Influenza A, influenza B, HSV-1, HSV-2, HIV-1, HBV |
MTT |
170
|
CoIII Schiff base complexes, CTC-96 (Co2, Doxovir™) |
HSV-1, HSV-2 |
Plaque-forming units |
169
|
ZnII-pyrithione (PT) |
SARS-CoV-1 |
MTT |
178
|
ZnII-cyclams |
HIV-1, HIV-2 |
MTT |
180
|
PtII-(phen)(acyclovir/penciclovir, Pt15†) |
HSV, CMV |
MTT |
171
|
Auranofin (Au1) |
SARS-CoV-2 |
qRT-PCR, plaque-forming units |
181
|
Ranitidine BiIII citrate (based on Bi1) |
SARS-CoV-1 |
qRT-PCR |
173
|
The Schiff base complex [CoIII(bisacetylacetatonato-ethylenediimine)(2-methylimidazole)2]+ (Co2, Doxovir™)169 reached phase II trials in 2011 for treatment of Herpes simplex labialis (causative agent of cold sores) and viral eye infections. Co2 appears to prevent the entry of herpes simplex virus type 1 (HSV-1) into cells by inhibiting membrane fusion events as well as cell-to-cell spread and syncytium formation.169 The antiviral activity of polyoxometallate 3D frameworks has long attracted attention. For example, Cs2K4Na[SiW9Nb3O40] (POM93) has broad and potent in vitro antiviral activity against influenza A/influenza B, herpes simplex virus (HSV; Vero cells), human immunodeficiency virus (HIV-1; MT4-4 cells), and hepatitis B and C (HBV, HCV; HepG2 cells).170 X-ray nanotomography shows that POM93 locates on the cell surface and prevents virus entry into the cell. PtII compounds containing an aromatic diimine and antiviral guanosine-type ligands acyclovir or penciclovir (Pt15†)171 exhibit activity towards HSV and cytomegalovirus.171
The efficacy of BiIII complexes against SARS-CoV-1 discovered in the aftermath of the 2003 outbreak of severe acute respiratory syndrome coronavirus (SARS-CoV-1), suggested that ranitidine bismuth citrate (based on Bi1 and L1†)172 is a strong inhibitor of the ATPase activity of the viral helicase protein (IC50 = 0.3 μM).173 Förster Resonance Energy Transfer (FRET)-based assays showed that Bi1 inhibits the DNA duplex unwinding activity (IC50 = 0.6 μM). BiIII binds to the cysteine-rich region of the N-terminal zinc binding domain of the helicase protein. In cell culture, Bi1 effectively inhibited virus reproduction with an EC50 of 5.9 μM, and a (low) cytotoxicity CC50 of 5 mM.173 qRT-PCR studies showed that Bi1 inhibits the replicative cycle of SARS-CoV-1.173 These data suggest that the efficacy of Bi1 may be due to inhibition of SARS-CoV-1 helicase, which possesses >99.5% sequence similarity to the helicase of SARS-CoV-2.174 Potassium bismuth citrate is currently on clinical trial for treatment of COVID-19 (Trial ChiCTR2000030398 in Wuhan), as well as the zinc ionophore hydroxychloroquine (IRCT20100228003449N28; IRCT20100228003449N29).175
Zinc is particularly interesting for both pathology and treatment of viral diseases. The Zn metallopeptidase angiotensin-converting enzyme 2 (ACE2) serves as the cellular entry point for both SARS-CoV-1 and SARS-CoV-2.176,177 The combination of ZnII and the ionophore, pyrithione, can efficiently impair the replication of SARS-CoV-1 in Vero-E6 cells.178 ZnII inhibits the RNA-synthesising activity of the multiprotein replication and transcription complex in SARS-CoV-1, and activity of viral RNA polymerase during the elongation phase of RNA synthesis.178 Ionophores such as pyrithione or chloroquine might facilitate cellular uptake of ZnII, thereby promoting this type of inhibition in vivo.
Human coronavirus 229E is inactivated on brass and copper-nickel surfaces,179 with CuI and CuII being essential for the inactivation. Superoxide and hydroxyl radical generation may play an important role in the inactivation of coronaviruses on copper alloys, whereas on the pure copper surfaces, the direct effect of copper ions is key.179
3.6 Adjuvants
Metal-based agents are also used as adjuvants to elicit an immune response. These are mainly used in vivo, but sometimes investigated in vitro for CD4+ T cell priming. Aluminium adjuvants have been incorporated into vaccines for over 90 years, in billions of doses administered to millions of people annually. Aluminium adjuvants are the “Gold Standard” of all adjuvants. Typically they are Al(O)OH (e.g. Alhydrogel®, PI 11.4) and Al(OH)x(PO4)y (e.g. Adju-Phos®, PI 5), containing aggregates of 10–50 nm-sized particles. The mechanism of action of these adjuvants is not understood, but probably involves adsorption/binding of antigens to the surface of the nanoparticles, induction of limited necrosis, and dendritic cell activation.182 Adjuvants for cancer immunotherapy are being explored, including the hierarchically porous, and Cu- and Zn-containing γ-Al(O)OH mesostrands that enhance anti-tumour immunity.183
3.7 Clinical translation: ADME and safety screening
Drug candidates need to display good pharmacokinetics and bioavailability as defined by their ADME (absorption, distribution, metabolism and excretion) profiles. Only minor adverse drug reactions are deemed safe for human use. Interactions with proteins/receptors different from the desired target can result in serious effects, e.g. blocking of the hERG potassium channel can cause fatal arrhythmias.184 Pharmaceutical companies have recently proposed a panel of targets (GPCRs, ion channels, nuclear receptors and enzymes), against which new compounds should be tested.185 Table S7† shows examples for the different target classes. As yet, such data are scarce for most clinically approved metallodrugs (although TOOKAD® was tested for hERG inhibition as part of its EMA assessment).186
Genotoxicity (damage to DNA determined by e.g. comet and chromosome aberration assays) and mutagenicity (induced mutations, e.g. Ames' test, mammalian cell HPRT gene mutation assay) also need to be assessed for anticancer agents, and can be confirmed by whole-genome sequencing. Mutagenicity of cisplatin was observed in early studies187 and confirmed in a wide range of cell types.188 Cisplatin induces both base substitution mutations as well as short insertion and deletion mutations around the intrastrand crosslink sites.189 Notably, oxaliplatin has a different mutational profile compared to cisplatin and carboplatin.190,191
More information and protocols for in vitro assays to evaluate health-effects of drug candidates are described in Section 4 of the OECD guidelines for the testing of chemicals.192
4. Cellular mechanisms of action
Through this section we present some approaches for metallodrug target identification and study of their mechanisms of action, discussing cell death mechanisms and highlighting techniques we can use to identify cellular targets. This information is becoming increasingly important to undergo clinical translation.
4.1 Cell death mechanisms
Determination of death mechanisms induced by metallodrugs can sometimes indicate direct or indirect target sites. A few common cell death mechanisms are described here, and examples of specific inhibitors commonly used in screening are in Table S8.†
Apoptosis (programmed cell death) is the most common cell death mechanism for metallodrugs. Caspase proteases are cleaved and activated during apoptosis. Cleavage of caspase-3 is used as a marker. Of the two main apoptotic pathways, the extrinsic pathway is activated by a death ligand binding to a death receptor on the surface of the cell, activating initiator caspase-8. The intrinsic pathway is activated by toxins, radiation, and some chemotherapeutics which cause mitochondrial changes, such as decrease in mitochondrial membrane potential, and activate initiator caspase-9.193
It is generally accepted that cisplatin-induced cell death is through apoptosis, with both intrinsic and extrinsic pathways activated.194 However, caspase-independent cell death mechanisms can also occur in cisplatin treated cells. Carboplatin also induces apoptosis, and examples of cell death pathways induced by other metal complexes are in Table 5.
Table 5 Examples of metallodrug-induced cell death pathways and identification methods
M |
Complex |
Pathway |
Method |
Ref. |
Ru |
NKP1339 (Ru9) |
ICD |
Calreticulin cell surface expression (flow cytometry), HMGB1 release to extracellular space (ELISA) |
195
|
Pt |
Oxaliplatin (Pt3) |
ICD |
HMGB1 release to extracellular space (ELISA) |
196
|
Calreticulin surface expression (flow cytometry), HMGB1 motility (western blot) |
197
|
Fe |
Dinitrosyl Fe complex (Fe3†) |
Apoptosis |
Annexin-V/PI apoptosis assay (FACS) |
198
|
PARP, caspases 3 and 9 (immunohistochemistry) from in vivo tumour tissue |
Cu |
Cu-TSC (Cu2†) |
Apoptosis |
Annexin V-FITC/PI apoptosis detection, caspase-3 assay, caspases 8 and 9 (qPCR) |
199
|
Ru |
RAPTA-C (Ru12) |
Apoptosis |
Cytochrome c release, procaspase-9 (western blot) |
200
|
Annexin-V/PI apoptosis assay (FACS) |
Pd |
[Pd(bipy)(O,O′-dkt)]+ (Pd2†) |
Apoptosis |
Annexin-V/PI apoptosis assay (FACS), mitochondrial membrane potential using JC-1 dye (FACS) |
201
|
Os |
FY26 (Os4) |
Apoptosis |
FlowCellect Cytochrome c Kit (FACS) |
202
|
MitoCapture Apoptosis Detection Kit (confocal) |
Ir |
Ir-TEMPO1 (Ir10†) |
Apoptosis |
Mitochondrial membrane potential using JC-10 dye (FACS) |
203
|
Pt |
Cisplatin (Pt1) |
Apoptosis |
Annexin-V/PI staining (FACS), mitochondrial membrane potential (JC-1), caspase-3 |
204
|
Pt |
Carboplatin (Pt2) |
Apoptosis |
Cytochrome c release, PARP cleavage/caspases (western blot) |
205
|
Au |
[Au(L2b)PPh3] (Au3†) |
Apoptosis |
Mitochondrial membrane potential and caspase-3 activity (FACS) |
206
|
Mn |
Adpa-Mn (Mn1†) |
Apoptosis and ACD |
PARP-cleavage/LC-3/ATG7 expression (western blot) |
207
|
Annexin-V/PI apoptosis assay (FACS) |
As |
As2O3 (As3† in aqueous solution) |
ACD |
MEK/ERK pathways (autophagy inhibitor studies, western blot) |
208
|
Pt |
Mono-Pt (Pt16†) |
ACD |
Anti-LC3 antibody (immunohistochemistry) |
209
|
Ru |
[(p-Cym)Ru(TsEn)Cl] (Ru11†) |
Necrosis |
Annexin-V/PI assay (FACS) |
103
|
Ru |
Cyclometallated Ru |
Necroptosis |
p-RIPK1/p-RIPK3 expression (western blot) |
210
|
Autophagy-induced cell death (ACD) is cell death preceded by autophagy, a normal process in healthy cells for catabolism and recycling of cytoplasmic components. Large numbers of autophagic vacuoles are formed to breakdown the cytoplasm. Many components of the ACD pathway can be screened, e.g. formation of autophagosomes during autophagy by formation of proteins LC3-I and lipid-modified LC3-II.211 Some Pt complexes induce this pathway, which represents a novel mechanism compared to cisplatin.209 Arsenic compounds, As2O3 and NaAsO2 induce ACD.212 Autophagy activation can also occur prior to apoptosis; for example, Adpa-Mn (Mn1†) induces PARP cleavage (apoptosis) and LC-3/ATG7 (autophagy).207
Necrosis involves loss of the membrane integrity through self-lysis of the cell, leading to ATP depletion. It was thought to be unregulated, however, death domain receptors and toll-like receptors may activate necrosis, when caspases are absent.213 This more regulated form of necrosis is also known as necroptosis. The catalyst [(p-cym)Ru(TsEn)Cl] (Ru11†) allows propidium iodide (PI) to enter cells through membrane disruption without the externalisation of phosphatidylserine indicating non-apoptotic cell death.103 Notably, cell death by necrosis can cause a host inflammatory response.
Immunogenic cell death (ICD) is aided by the immune system. The damaged cell releases and expresses damage-associated molecular patterns (DAMPs) which recruit immune cells to the damaged cell and cause cell death through T cell activation. HMGB1 and ATP release and calreticulin relocation are key components of this pathway, examples of DAMPs which can be screened to detect ICD.214 Oxaliplatin (Pt3) induces ICD detectable by HMGB1 in patient serum and released from cell lines.196,197 NKP1339 (Ru9) induces ICD with calreticulin relocation and HMGB1 release.195 Importantly, the anticancer activity of oxaliplatin and related PtIV complexes in in vivo models often requires an intact immune system.215 Effectively some metal complexes may act as vaccines against cancer recurrence. The role of metal-based drugs in the anticancer immune response has been reviewed recently.216
4.2 Effect of cell cycle
Unabated deregulation of the cell cycle is a hallmark for cancer. Metallodrugs halt progression of the cell cycle at different stages, allowing time for cells to repair DNA damage.130 Cell cycle arrest is determined experimentally by Fluorescence-activated Cell Sorting (FACS), and PI staining identifies the cell DNA content by intercalating into DNA. Cell cycle analysis can indicate the MoA of a metal complex, whether this involves DNA binding or not.
In HL-60 leukaemia cells, at lower doses, cisplatin-induced DNA adduct formation arrests cells at the sub-G1, S or G2 phases. At higher doses and treatment times, cell accumulation increases within the sub-G1 phase, indicative of apoptosis and necrosis.217 Innate and acquired cisplatin resistance involves various proteins and pathways (Fig. 7). In A549 lung cancer cells, it is associated with the loss of cisplatin-induced G2/M phase cell cycle arrest and reduced apoptosis. Loss of activation of p53 and pATM (major responders to Pt-DNA adducts) are likely essential for cisplatin resistance.218 The impact of metallodrugs on cell cycle distribution differs according to the exposure time and cell line (Fig. 8). Increasing the exposure time of oxaliplatin to 72 h induces G2/M arrest in HeLa, MCF7 and HT29 cell lines, but A549 cells exhibit elevated G0/G1 populations.219
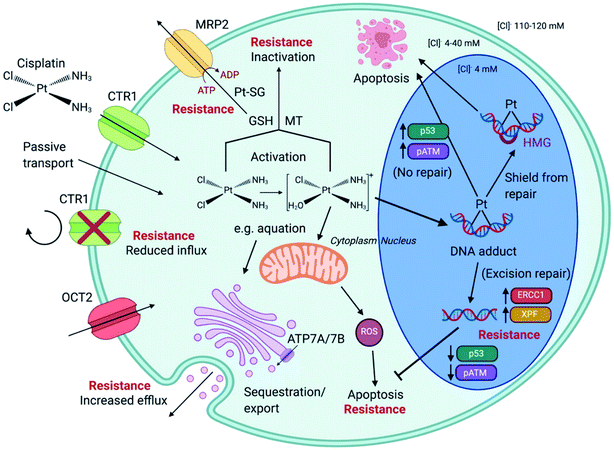 |
| Fig. 7 Cisplatin (CDDP) resistance in cancer cells. CDDP enters cells via active transport (e.g. via transporters CTR1 and OCT2) or by passive transport. Reduced influx and elevated efflux are forms of CDDP resistance. The ATPases ATP7A/B are located at the Golgi apparatus, in the trans-Golgi network, facilitating the sequestration and export of cisplatin within membrane vesicles that are released. Intracellular CDDP activation occurs by aquation (first stage shown). Activated CDDP can react with glutathione (GSH) to form platinum-GSH conjugates (Pt-SG) and leave the cell via MRP2, or interact with metallothionein (MT), contributing to CDDP tolerance. CDDP exposure generates mitochondria-dependent reactive oxygen species (ROS). CDDP enters the nucleus and forms platinum–DNA adducts. Shielding from repair, by high mobility group (HMG) protein protection, or no repair leads to apoptosis. Excision-repair and high expression of proteins such as ERCC1 and XPF, subsequently inhibit apoptosis. Augmented expression of p53 and pATM is associated G2/M arrest of the cell cycle and apoptosis, in contrast to lowered expression, linked to CDDP resistance. Created with https://www.BioRender.com. | |
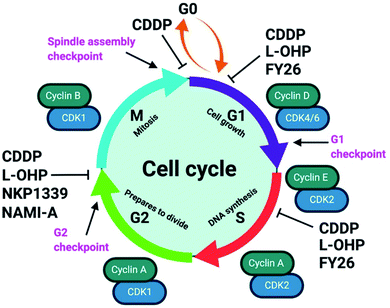 |
| Fig. 8 Examples of cell cycle arrest by metallodrugs. Abrogation of the cell cycle enables the initiation of apoptosis to control cell proliferation. Metal complexes can inhibit cell cycle progression at different stages which vary between different cell lines, doses, and exposure times. The cell cycle checkpoints (pink) determine progression to the next stage, and cell cycle regulators include cyclins (green) and CDKs (cyclin-dependent kinases, blue). Subpopulations of cells enter a quiescent state at the G0 phase. CDDP (cisplatin, Pt1); L-OHP (oxaliplatin, Pt3); NAMI-A (Ru4); NKP1339 (Ru9); FY26 (Os4). Created with https://www.BioRender.com. | |
The organo-IrIII complex ZL109 (Ir7†) stimulates cell cycle arrest in A2780 ovarian cancer cells after 24 h exposure either during replication (S-phase) or after the doubling of DNA content (G2-phase).149 Organo-OsII complex FY26 (Os4) inhibits cell cycle progression at the G1-phase, prior to DNA replication, consistent with its proposed redox modulation (ROS) and not a DNA-focussed MoA.220 ROS can also cause DNA damage indirectly. RuII polypyridyl complexes [Ru(dmb)2(NMIP)](ClO4)2 (Ru13†) and [Ru(phen)2(NMIP)](ClO4)2 (Ru14†) arrest the cell cycle at different stages in BEL-7402 hepatocellular carcinoma cells, at G0/G1 and S phases, respectively (Table S9†).221 Cellular targets for Ru complexes (Table S9†) are not focussed solely on DNA interactions, and include chromatin and histones, disruption of protein–protein interactions, redox modulation, and enzyme inhibition.222
Diastereomeric helical “flexicate” [Fe2L31a]Cl (Fe4†) complexes arrest HCT-116 p53+/+ cells at G2/M. In contrast, [Fe2L32a–b]Cl4 (Fe5†) complexes show no significant G2/M population increase, indicating an alternative binding target and MoA.223
4.3 Chronopharmacology
The circadian timing system (CTS) rhythmically controls cellular and physiological functions, such as xenobiotic detoxification and metabolism, over a 24 hour period. The CTS regulates apoptosis, DNA repair and transitions of the cell cycle. The optimal circadian timing of anticancer drug administration can be determined by the CTS.224
GSH plays a critical role in cisplatin toxicity. Buthionine sulfoximine, which inhibits GSH biosynthesis and induces a 12 h rather than 24 h rhythm, results in potentiated cisplatin toxicity useful for clinical optimisation.225 Wild-type mice have better tolerance towards cisplatin in the evening than in the morning, while Per1/2−/− (clock gene) mice show no clear difference. Nucleotide excision repair appears to remove cisplatin-DNA adducts in a clock-controlled manner.226
The circadian expression of organic cation transporter 2 (OCT2, encoded by SLC22A2), involved in cisplatin renal excretion, is closely associated with time-dependent alterations in cisplatin-induced nephrotoxicity.227 Cisplatin-resistant cells upregulate ATF4 (direct target of clock) and the expression of clock correlates with cisplatin sensitivity. The clock and ATF4 transcription system could mediate multidrug resistance via the GSH-dependent redox system.228
The overexpression of Bmal1 (clock gene) leads to an increased sensitivity (inhibition of proliferation, apoptosis and cell cycle arrest) towards oxaliplatin both in vitro and in vivo.229
Analysing all chronopharmacological aspects of a novel compound is costly and time-consuming, but potentially important for clinical use of metallodrugs. Two randomised phase III trials reported that 278 metastatic colorectal cancer patients receiving chrono-modulated delivery of oxaliplatin exhibited decreased treatment side effects with a 5-fold difference and twice the anti-tumour efficacy.224 The relationship between circadian rhythm and chemotherapy is illustrated for oxaliplatin in Fig. 9.225 Furthermore, organo-osmium complex FY26 (Os4) exhibits temperature rhythm-driven in vitro and in vivo chronotolerance. The tolerability of Os4 is highest near the lowest point of the circadian temperature cycle.230
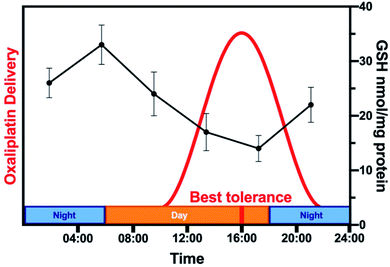 |
| Fig. 9 Relationship between circadian rhythm and chemotherapy. The rhythm of the GSH content of mouse liver (black, based on data from ref. 225) is compared to the schedule of administration of oxaliplatin (Pt3) in a typical chronomodulated chemotherapy. Patients show best tolerance at 16:00 (red). Oxaliplatin is detoxified by conjugation to glutathione, hence, tolerance is linked directly to GSH levels (note the 12 h phase-shift between the highest GSH levels in mice and best tolerance in humans due to nocturnality of mice). | |
4.4 Target identification
Identification of intracellular molecules and biochemical pathways as targets for new therapeutic agents is a key step in medicinal chemistry. However, this aspect has been somewhat neglected in the development of metallodrugs for which DNA is often assumed as the natural target, based on cisplatin (Pt1) activity.15 The techniques now available, briefly reviewed in the following paragraphs, allow new and different targets to be discovered for both novel and clinically established metallodrugs.
4.4.1 CRISPR screening.
Genome-wide CRISPR screening techniques include options to knockout, silence, or activate genes. Current applications to metallodrugs focus on the original Cas9 version, which causes DNA double-strand breaks and result in frame-shift mutations to knockout a gene.
Limitations of genome-wide CRISPR screening include high levels of off-target effects and large background signals using a pooled screen.231 The problem with large background signals is amplified by multi-targeted metal complexes, which necessitates use of a high concentration of complex and multiple replicates. There are only 4 reported genome-wide CRISPR studies of resistance to cisplatin or any metal complex. The role of DNA repair protein MSH2 in cisplatin-mediated cell death in bladder cancer cells has been identified,232 and the transcription factor ZNF587B, and extracellular sulfatase SULF1, were found to contribute to cisplatin-mediated cell death in two ovarian carcinoma cell lines.233 Both negative and positive selection genome-wide screens have identified pathways involved in cisplatin resistance and cisplatin sensitivity in human melanoma cells.234 A combination study with cisplatin and the folate antimetabolite pemetrexed demonstrated that loss of WEE1 sensitises cells to this treatment regime and is a potential therapeutic target.235
4.4.2 RNAi screening.
RNA interference (RNAi) is a natural cellular mechanism, which protects genomes by inhibiting gene expression or translation. RNAi causes the degradation of mRNA, so preventing the production of the protein, resulting in knocking down gene expression. Drugs with similar mechanisms of action are likely to elicit similar shRNA-dependent responses. Though different techniques can be used (e.g. siRNA, shRNA or miRNA), the principle remains the same. A piece of selected dsRNA is constructed and cut by the enzyme Dicer to create siRNA, which then becomes part of an RNA-induced silencing complex that binds to the target mRNA and degrades it.236 RNAi-mediated suppression of cell death regulators in mammalian cells can affect the cellular response to drugs. In this way, the effect of genes known or predicted to have roles in cell death can be investigated. Examples of reported RNAi studies for cisplatin are in Table S10.†
Eight such genes have been investigated by Bruno et al. for their role in the cytotoxicity of cisplatin, carboplatin and oxaliplatin.237 Unlike cisplatin and carboplatin, oxaliplatin (at an LD80 dose) did not induce a DNA-damage response, but instead induces ribosome biogenesis stress. Further work is needed to determine whether specific proteins are targeted by oxaliplatin. Using RNAi signatures for cancer cells, an OsVI nitrido complex Os8 showed a pattern between DNA cross-linkers e.g. cisplatin, and ER stress inducers, e.g. tunicamycin, indicating that it could initiate both DNA damage and ER stress.238 Silencing the ERCC-1 gene in cisplatin-resistant SGC-7901 human gastric cancer cells induces apoptosis and lowers the IC50 after cisplatin treatment for 48 h. ERCC-1 plays a critical role in the DNA repair pathway, removing cisplatin–DNA adducts.239
High throughput RNAi screening can provide a relatively rapid screening method for genome-scale functional studies.240 An RNAi screen for 2400 genes identified genes engaged in DNA repair-enhanced sensitivity towards cisplatin and other anticancer drugs. BRCA pathway genes (BRCA1, BARD1, BRCA2, and RAD51) were the most prominent in enhancing sensitivity towards cisplatin.241 Interference in selected genes may result in an improved outcome through a synergic effect alongside chemotherapy. For example, silencing E6 or E7 protein of human papillomavirus, which degrades tumour-suppressor p53 or retinoblastoma proteins in combination with cisplatin results in induced apoptosis in vitro and inhibition of tumour growth in vivo.242 Silencing survivin and bcl-2 in cisplatin-resistant A549 cells results in increased sensitivity towards cisplatin, in vitro and in vivo.243
4.5 Pathway analysis by omics techniques
Metallomics provides a powerful systems biology approach for elucidating the biomolecules, processes, and pathways involved in the dynamic speciation of metal ions and their quantitative distribution in cells and tissues.244Table 6 gives examples of metallodrugs investigated by multi-omics techniques. Genomics and transcriptomics speed up the study of DNA and RNA (especially mRNA), and can provide insights into biological processes including DNA replication, nucleotide excision repair and transcription. Microarrays are widely applied to fast screening, without complicated sample preparation. Time-series whole-transcriptome sequencing shows that FY26 (Os4) rapidly activates oxidative stress reponse pathways and induces a shift of cellular energetics from glycolysis to oxidative phosphorylation in A2780 ovarian cancer cells.220
Table 6 Examples of metallodrugs investigated by multi-omics techniques
M |
Complex |
Method |
Mechanism of action |
Ref. |
As |
ZIO-101 (As2) |
Metalloproteomics by GE-ICP-MS |
Binds to and disrupts dimerisation of H3.3 |
248
|
Ru |
NAMI-A (Ru4) |
Proteomics by MALDI-TOF or LC-ESI/MS–MS |
NAMI-A and RAPTA-T similar mechanism; NIT2, TMK, HINT1 and PFD3 up-regulated, POLE3 down-regulated |
250
|
Ru |
Plecstatin-1 (Ru15†) |
Proteomics by LC-MS/MS |
Targets plectin |
251
|
Ru |
RAPTA-C (Ru12) |
Proteomics by shotgun and pull-down |
Influences cytokines midkine, pleiotrophin, fibroblast growth factor-binding protein 3, FAM32A |
252
|
Os |
FY26 (Os4) |
RNA transcriptomics and proteomics by reverse-phase protein arrays |
Shifts cellular metabolism to oxidative phosphorylation, up-regulates ATM, p53 and p21 |
220
|
Pt |
Cisplatin (Pt1) |
Genomics by damage-seq, XR-seq, RNA-seq |
Per1 upregulated. Transcription-coupled repair and global repair high in liver |
253
|
Proteomics by nanoLC-MS/MS |
HMGB1 binds to cisplatin cross-linked ODN specifically |
254
|
Metabolomics by GC-MS, LC-MS |
27 potential biomarkers defined related to cisplatin-induced nephrotoxicity |
255
|
Au |
[Au(TPP)]Cl (Au4†) |
Proteomics by MALDI-TOF-MS |
Targets Hsp60 |
256
|
Affinity-based proteome profiling SILAC |
246
|
UPLC and high-resolution MS have improved sensitivity and selectivity in proteomics. Besides the species and expression level, proteomics can reveal post-translational modifications, e.g. ubiquitination, phosphorylation, and glycosylation. Proteomics based on LC-MS/MS identified cisplatin cross-linking at the domain I/II interface zinc binding site (His67 and His247).245 Chemical proteomics with click reactions and photo-crosslinking identified heat-shock protein 60 (Hsp60) as the target of gold anticancer complex [Au(TPP)]Cl (Au4†).246 Hsp60 was confirmed as a target by quantitative proteomics using stable isotope labeling with amino acids in cell culture (SILAC), as well as the cellular thermal-shift assay (CETSA).246
Small metabolites can be analysed by NMR, or MS with sample preseparation by GC or LC/HPLC giving orders of magnitude increase in sensitivity and selectivity. Metabolomic GC-MS profiling shows that mitochondria-targeting [Re2(CO)6(4,7-diphenyl-1,10-phenanthroline)2(L)](PF6)2, where L is 4,4′-azopyridine (Re-Re1) or 4,4′-dithiodipyridine (Re-Re2†), induces oxidative stress by down-regulating 5-oxoproline to (20–31%) and decreases GSH levels to (34–40%) affecting glutathione metabolic pathways in HeLa cells.247 GE-ICP-MS revealed Cys in histone H3.3 as a target for anticancer drug S-dimethylarsino-glutathione (As2), but not for the drug As2O3 (As3† in aqueous solution, Trisenox) in leukaemic cells, disrupting dimer formation and facilitating denaturation of nucleosomes.248
A new deep learning bioinformatics approach based on database integration has revealed disease-associated metal-relevant site mutations in the first and second coordination spheres of metalloproteins.249 For example, the mutation Arg174Gly in the second coordination sphere of the Zn(Cys)3His site in tumour suppressor p53 is a disease-associated mutation.249
5.
In situ analysis and imaging of metallodrugs
In this section we discuss some of the methods commonly used to detect and visualise metallodrugs in cells and tissues and the specific advantages and challenges of each technique. A graphical overview of the techniques discussed is presented in Fig. 10.257
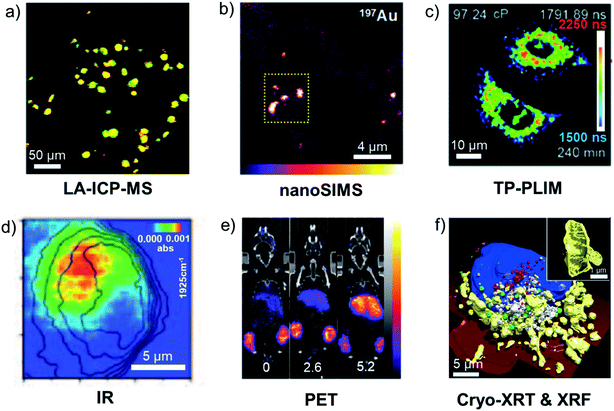 |
| Fig. 10 Examples of metallodrug mapping by the indicated techniques. (a) LA-ICP-MS image of 193Ir (red) and 238U (green) in HeLa cells (overlay = yellow, scale bar 50 μm).258 (b) nanoSIMS image of 197Au in A2780 cells treated with AuMesoIX (Au2, scale bar: 4 μm).55 (c) Mitochondrial viscosity in Ir3-treated A549 cells determined by two-photon phosphorescence lifetime imaging (scale bar: 10 μm).259 (d) IR image of a Re–CO complex at 1925 cm−1 in MDA-MB-231 cells (scale bar 5 μm).260 (e) Biodistribution in a mouse of positron-emitting 89Zr-antibody conjugated to a drug via a Pt complex at different drug : antibody ratios (0, 2.6 and 5.2).261 (f) 3D-reconstructed image of Ir3-treated MCF7 cells, illustrating morphology of suborganelle structures (scale bars: 5 μm and 1 μm) blue: nucleus, red: dense vesicles, green: lipid droplets, white: vacuoles, yellow: mitochondria, dark red: cell membrane.262 Illustrations are adapted from the references given. | |
A major challenge when analysing metallodrugs in cells and tissues is preserving their distribution in organelles, and speciation (oxidation state and coordination environment). Metal speciation is often dynamic in nature and can change with e.g. time and temperature in both intact and preserved samples. Therefore, the possible effects of all handling procedures on the metallodrug and its metabolites need to be considered.
Cell preservation. Methods include cryopreservation, chemical fixation, dehydration, resin-embedding, and sectioning (extended reading, S3 ESI†). Common chemical fixatives include aldehydes (e.g. glutaraldehyde) or alcohols (e.g. ethanol) which rapidly preserve cells by crosslinking or precipitation of proteins. Cryopreservation avoids chemical treatment by rapidly preserving cells at low temperatures (e.g. 103 K, liquid ethane) in a near-native state. However, great care has to be taken to vitrify rather than crystallise water during freezing by extremely rapid cooling. With care and temperature control, freeze-drying can be used to dehydrate cells after cryopreservation. Fixed samples embedded in paraffin wax or frozen samples embedded in “optimal cutting temperature compound” (OCT, polymeric mixture) can be sectioned on a microtome, although thin sections can be difficult to handle.
5.1 Inductively coupled plasma (ICP) methods
ICP-MS can quantitate a wide range of elements in biological solutions down to ppb range, ca. 100-fold more sensitive than Optical Emission Spectroscopy (ICP-OES).263 It is a destructive technique, and only provides information on elemental content. This limitation can be partially addressed by ‘hyphenated’ experiments such as SEC-ICP-MS (vide supra).123 The preparation of cell and tissue samples for ICP-MS analysis requires thermal digestion and the use of concentrated acids/bases to ensure complete sample homogenisation.263 Tougher tissues often require H2O2 and/or the use of pressurised microwave heating.264 A compatible solvent matrix for the analyte must be selected to avoid interferences and loss of elements (e.g. Os as volatile OsO4 under acidic conditions).265
Fractionation methods can be used to analyse distinct cellular components (e.g. membranes, nuclei, cytoplasm, mitochondria), extracted by lysis of cells followed by ultracentrifugation to separate components that differ in size/weight.266
5.1.1 Single-cell ICP-MS.
Whereas cell digestion ICP-MS studies provide only an average measurement of an element in a sample of cells, single-cell ICP-MS can determine the time-resolved elemental composition of individual cells amongst a population. For example, the uptake of Pt by A2780 cisplatin-sensitive ovarian cancer cells treated with 10 μM cisplatin (ca. 4 × 106 Pt atoms per cell) was found to be greater than for resistant cells (ca. 3 × 106 Pt atoms per cell).267
5.2 Imaging mass spectrometry
Mass spectrometry combined with ablation techniques can provide information on the spatial distribution of metals and fragments of metallodrugs.
The spatial distribution of Ru in the liver, kidneys, muscles and tumours of CT26 tumour-bearing mice treated with anticancer organoruthenium plecstatin-1 (Ru15†) determined by laser ablation-ICP-MS (LA-ICP-MS) is similar to its isosteric Os analogue, in good agreement with ICP-MS data after digestion.268
Nanoscale secondary ion mass spectrometry (nanoSIMS) offers spatial resolution (down to ca. 50 nm) an order of magnitude better than photon-based ablation.269 Ablation and ionisation in SIMS occur in the same process, and molecular information is typically not preserved. The energy of the primary ion beam is equivalent to X-ray wavelengths (0.06–8 nm). Molecular information can be better preserved in SIMS by use of larger primary ions, e.g. large Ar clusters.270 High ion doses allow depth profiling.270
NanoSIMS studies of 300 nm-thick sections of resin-embedded colon cancer cells treated with 15N-labelled cisplatin (10–150 μM) suggest partial dissociation of Pt–N bonds in at least the nucleoli, based on the Pt/N stoichiometry, and co-localisation of Pt with S and P (detection of 194Pt, 34S and 15N signals), consistent with the affinity of PtII for S-rich proteins and DNA.271
Similarly, correlative nanoSIMS and electron microscopy imaging of AuIII mesoporphyrin IX dimethyl ester (Au2) in A2780 cells suggests that this anticancer complex attacks sulfur-rich proteins in mitochondria (197Au in regions with high 32S/12C14N ratios).55 There is much scope for isotopic labelling of both metals and ligands in such studies.
5.3 Luminescence imaging
The cellular localisation of luminescent metal complexes with triplet metal-to-ligand charge-transfer (3MLCT) excited states can be monitored by fluorescence microscopy.272 Such emissions are often tuneable and intense, and have large Stokes shifts, long emission lifetimes, high signal-to-noise ratios, and high photostability.272,273 For example, RuII complex TLD-1433 (Ru6) exhibits red luminescence (580–680 nm) from an emissive 3MLCT state and generates ROS with a large 1O2 quantum yield (Φ(Δ) ∼ 0.99 in acetonitrile) from a intraligand 3IL state when excited by green light (530 nm).274
Weakly- or non-luminescent metal complexes can be conjugated to fluorescent organic dyes or more-strongly luminescent metal complexes to allow their cellular imaging.78,275 The non-emissive dinuclear (bpy)2RuII-EuIII(cyclen) (Eu-Ru1†) complex releases DNA-targeting RuII and an emissive EuIII(cyclen) species upon irradiation at 488 nm.276 The red emission of EuIII(cyclen) species can be detected in cancer cells with 350 nm one-photon or 700 nm two-photon near-infrared excitation.
Two-photon imaging provides increased light penetration depth and nano-localisation.277,278 Mitochondria-targeting RuII polypyridyl PS Ru6† for example, showed cellular fluorescence with both one- and two-photon excitation, but the deeper spheroid penetration achieved with two-photon excitation highlights the increased depth advantage of the two-photon method.279
The problem of background autofluorescence of cells can be overcome using fluorescence/phosphorescence lifetime imaging (FLIM/PLIM), improving signal-to-noise ratios and providing quantitative information.272 Cyclometalated IrIII anticancer complex Ir11† exhibits a specific response to the local viscosity, allowing mitochondrial viscosity and heterogeneity to be monitored in real-time using two-photon-PLIM.259
5.3.1 Theranostic luminescent complexes.
These can serve the dual purpose of in situ prodrug activation (therapy) and activation-induced luminescence for imaging of cellular localisation (diagnosis). Metal centres can be designed to quench fluorescence after redox activation, usually by the conversion of a diamagnetic to a paramagnetic metal (e.g. RuII to RuIII).280 When theranostic PtIV prodrugs with a conjugated aggregation-induced emission (AIE) group are reduced to active PtII drugs, the AIE group is released and fluoresces, allowing direct measurement of metallodrug activation and oxidation state.281
5.4 Vibrational spectroscopy
Carbonyl stretches for metal carbonyl complexes inside cells are readily observable in the 1800–2200 cm−1 region, which is relatively free of peaks from biomolecules. For example, the uptake and distribution of [(Cp)Re(CO)3] conjugated to a hydroxytamoxifen-like structure (Re2†) in breast cancer cells has been determined by photothermally-induced resonance (PTIR, 20–50 nm resolution) with an AFM coupled to a tuneable pulsed infrared laser. Re2† was found to be localised in the nucleus of whole cells at a 10 μM dose.260 Photoinduced CO releasing molecules (PhotoCORMs) and photoinduced NO releasing molecules (PhotoNORMs) are of therapeutic interest.282,283 3D Raman microscopy of living HT29 colon cancer cells treated with 2 mM of the photoactivated anticancer complex [Mn(tpm)(CO)3]Cl (Mn2) for 3 h, revealed accumulation in the nuclear membrane and nucleus.284
5.5 Radionuclide labelling
Introduction of a radioactive label into a pharmaceutical agent should cause minimal change to its molecular structure. Radiometals are often attached to a targeting vector via a bifunctional metal chelator. By changing a therapeutic (e.g. α or β emitter) to an imaging (e.g. γ or positron emitter for SPECT and PET imaging, respectively) radiometal, the pharmacokinetics of a metal-based radiotherapeutic can be imaged.
A “theranostic pair” consists of a combination of two agents with the same/similar structure, one therapeutic and the other diagnostic. For example, the PET agent 68Ga-DOTA-PSMA (M2†) has been used to monitor the response of the beta emitter 177Lu-DOTA-PSMA and the alpha emitter 225Ac-DOTA-PSMA in prostate cancer patients.285
Non-radioactive metallodrugs can also be radiolabelled, either at the ligand or at the metal centre, to understand their mechanism of action or biodistribution. For example, cancer cells treated with half-sandwich osmium complex FY26 (Os4) radiolabelled with 131I release the iodide ligand, probably after reaction with intracellular GSH.29
An example of metal radiolabelling is [195mPt]cisplatin, the biodistribution of which was investigated in humans in the early 1970s.286 [195mPt]carboplatin distribution has also been investigated in preclinical models.287 Recent improvements in imaging equipment and production of 195mPt radiolabelled complexes have seen a resurgence of such studies with a focus on preclinical imaging.261,288
[Cu(ATSM)] (Cu3) was developed first as a hypoxia imaging agent, [64Cu][Cu(ATSM)],289 but later recognised as a SOD-1 agonist.290 [Cu(ATSM)] is now in clinical trials for motor neuron diseases such as Parkinson's disease (completed, GDCT0292870) and Amyotrophic Lateral Sclerosis (ongoing, GDCT0344437).
Many other radiometals could be used to elucidate the fate of metallodrugs in vitro and in vivo. The chemistries of 68Ga and 89Zr are well developed and should be considered for monitoring the biodistribution of drug candidates containing such metals. In addition, other radiometals, including 52Mn and 62Zn/63Zn, are becoming increasingly available for preclinical investigations.
5.6 Synchrotron X-rays
Synchrotron radiation covers a wide range of the electromagnetic spectrum: from infrared (1.24 meV), to soft and hard (>ca. 10 keV) X-rays. This broad range enables X-ray Fluorescence (XRF); X-ray Absorption Spectroscopy (XAS), X-ray Tomography (XRT) and Infrared Microspectroscopy.
Absorption of a high-energy X-ray photon by an atom causes ejection of an inner-shell electron. When an electron in a higher-energy orbital subsequently drops down to occupy this hole, a photon is emitted, resulting in XRF. The energy of the emitted photons is characteristic of specific elements, allowing simultaneous detection and quantification at trace levels as low as 100 ppb, down to 50 nm resolution.291,292 Endogenous elements such as Zn, P, or S exhibit K-L3 emissions (2p3/2−1 → 1s−1) in the energy range 2–10 keV. In contrast, the K-L3 emissions of exogenous heavy elements (e.g. Pt, Ir, Os) are significantly higher in energy (>60 keV); thus, typically their L3-M5 emissions (3d5/2−1 → 2p3/2−1) are monitored (Fig. 11).
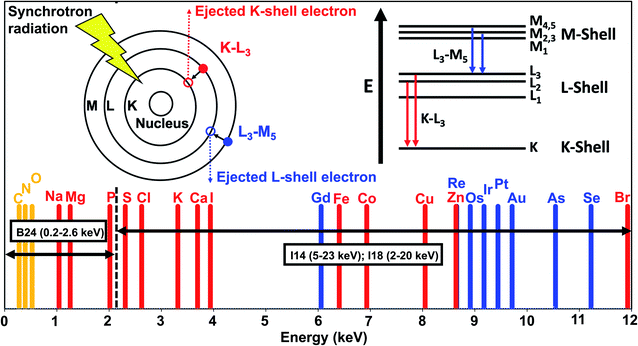 |
| Fig. 11 Characteristic K-L3 (2p3/2−1 → 1 s−1) and L3-M5 (3d5/2−1 → 2p3/2−1) X-ray fluorescence emissions. K-L3 for elements exploited in cryo-soft-X-ray tomography are in yellow, abundant endogenous elements in red; L3-M5 of exogenous elements are blue. Additional elements are reported in Table S11† B24 and I14 refer to beamlines at the Diamond synchrotron (http://www.diamond.ac.uk). | |
Synchrotron-XRF can provide insights into the biological distribution and localisation of metallodrugs, both in vitro (chemically-fixed, sectioned, or freeze-dried cells) and ex vivo (tumour spheroids and xenograft models).292,293 3D XRF-computed tomography has been used to investigate the penetration of Pt complexes (Pt17†, Pt18†) in tumour spheroids, demonstrating >150 μm depth.294 The localisation of Os in small elliptical cytoplasmic compartments of A2780 cancer cells treated with FY26 (Os4) implicated mitochondria as cellular targets.295 Non-metal exogenous elements (e.g. Br or I, low natural abundance) can also be employed to monitor the intracellular fate of metallodrugs by XRF, as demonstrated for Br-labeled Pt agents (Pt19†, Pt20†) and I-labelled rhenium (Re3†) complexes in vitro,296,297 through a dual-mapping approach.
5.6.1 X-ray Absorption Spectroscopy (XAS).
Absorption of an X-ray photon induces the transition of a core electron (typically 1s, 2s or 2p) into a vacant state according to Fermi's Golden Rule. X-ray Absorption Near Edge Structure (XANES) focuses on the region within 50–100 eV of the absorption edge.292 It is highly sensitive to the absorbing environment of the metal, and considers multiple scattering contributions. XANES can inform on the oxidation state, electronic structure and the coordination environment, but is typically limited to concentrated samples, and can be destructive and time-consuming.292 XANES studies can provide insights into the speciation and stability of metallodrugs in biological media. Pt XANES (L3 = 11.57 keV) has demonstrated the stability of PtIV prodrugs in human blood serum but rapid in-cell activation.298 Similarly, Ru XANES (K = 22.1 keV) has revealed potential interactions between RuIII drugs Ru4 (NAMI-A) and Ru5 (KP1019)† and the sulfur, amine and carboxylate groups of proteins in biological media.299
Extended X-ray Absorption Fine Structure (EXAFS) is observed ca. 50 to 1000 eV above the absorption edge, providing a unique fingerprint region of the XAS spectrum. EXAFS usually considers only single scattering contributions and is highly sensitive to neighbouring environments, thus providing information on metal–ligand distances (up to ca. 4 Å) and the number and type of neighbouring atoms.292 However, EXAFS requires relatively high concentrations, which hinders its use for in cellula, ex vivo or in vivo analysis. Instead, EXAFS is commonly used to study metallodrug interactions with relevant biomolecules, e.g. to demonstrate the catalytic activation of Os4via the formation of GSH adducts.300
5.6.2 Soft X-ray Tomography (XRT).
‘Soft’ X-rays are less penetrating than hard X-rays and more strongly absorbed, providing greater absorption contrast for lighter, biological materials (C, N, O). Also, a soft X-ray beam is easier to focus to spot sizes down to a few tens of nm.301 At this extremely high spatial resolution, depth of field is often <1 μm, requiring very thin samples and careful optimisation of sample position. Measurements are performed in vacuo since soft X-rays are efficiently absorbed by air and water. Conversely, attenuation of hard X-rays by air is negligible. An important consideration with X-ray spectroscopy is beam damage. The photon dose needs to be carefully controlled, using known standards and/or repeat measurements.302
Cryo-XRT allows 3D morphological and ultrastructural analysis of intact frozen-hydrated cells or tissues,303 using energies between the K-edges of C (284 eV) and O (543 eV). Organic materials absorb strongly in this energy range, the ‘water window’, whilst the X-ray beam is minimally attenuated by ice. Consequently, carbon-dense materials (e.g. mitochondria or lipid droplets), provide natural contrast against water from vitrified ice on the samples and in the cytoplasm down to a spatial resolution of 30–40 nm.304 Cryo-XRT has been used to probe the effectiveness of cisplatin in the presence of chelating tricine, which causes significant cell damage and numerous apoptotic bodies.305 Similarly, cryo-XRT revealed significant mitochondrial damage in photoirradiated cancer cells treated with a cyclometallated IrIII photosensitiser Ir12.306 Cryo-XRT can be coupled to other techniques such as structured illumination microscopy (cryo-SIM)304 or hard-XRF, which enables the direct detection of metal complexes overlaid with the 3D subcellular morphology. Notably, cryo-XRT and cryo-XRF of (near-native) cancer cells treated with a potent half-sandwich IrIII complex (Ir3) have revealed the unambiguous mitochondrial localisation of Ir (L3-M5 = 9.18 keV).262 The capability of obtaining native-state “snapshots” of biological systems emphasizes the notable advantage of cryo-XRT over conventional microscopy techniques.
5.6.3 Synchrotron-IR microspectroscopy.
Live, single-cell analysis can be carried out, providing morphological and biochemical insights of biological specimens in their native states,307 for example, live fibroblasts.308 Synchrotron-IR is also invaluable for monitoring metallodrugs, by either directly probing the metallodrug modality, or the drug-induced cellular changes.309
6. Conclusions and future outlook
This perspective is being written during the COVID-19 pandemic, which has emphasised more than ever the urgent need for new medicines. Medicinal inorganic chemistry can play a major role in such development, providing tools complementary to those of organic medicinal chemistry. Inorganic compounds and metals in particular, can introduce novel, unique mechanisms of action, which cannot be duplicated by organic compounds.
A comprehensive survey of current literature on the development of metal complexes as antiviral drugs by Bergamini et al. has just been published, asking the question “What is holding back the development of antiviral metallodrugs”.310
SARS-CoV-2 enters cells via the ACE2 receptor.177 ACE2 is a zinc carboxypeptidase (which cleaves C-terminal Phe from angiotensin II, DRVYIHPF).176 Dexamethasone, a corticosteroid used for treating critically ill COVID-19 patients, induces the Cys-rich protein metallothionein which binds clusters of ZnII and CuI ions.311 Potassium BiIII citrate is in phase III clinical trials (ChiCTR2000030398) for treating the disease. Bismuth can displace ZnII in proteins vital for the life cycle of coronaviruses.173 Importantly, Sun et al. have now shown that the antiulcer drug ranitidine bismuth citrate (RBC) exhibits low cytotoxicity and protects SARS-CoV-2-infected cells with a high selectivity and suppresses SARS-CoV-2 replication, leading to decreased viral loads in both upper and lower respiratory tracts, and relieves virus-associated pneumonia in a golden Syrian hamster model.312
Moreover, a high selenium status appears to improve the cure rate for COVID-19 patients.313 Vaccines for SARS-CoV-2 are being developed. The potency of many vaccines is enhanced by AlIII adjuvants, but the molecular basis for this is not understood.
In the field of antimicrobials, the hit-rate for metal compounds in some screens is 10× higher towards ESKAPE pathogens than that for organic compounds.3 It has long been known that metal surfaces (e.g. Cu and its alloys, Ag) are potently antiseptic, but the molecular basis is poorly understood. High-throughput screening has revealed the potent antiparasitic activity of the antiarthritic gold drug auranofin.167
The success of platinum complexes, the drugs used in about 50% of all cancer chemotherapies, raises the question as to why more metallodrugs are not being approved for clinical use. It is remarkable that 71 kinase inhibitors, a single class of organic drugs, are clinically approved (with >100 others in late-stage development),314 about twice as many as the total number of currently approved metallodrugs (Fig. 1).
We have focussed on in vitro screens since they are a convenient discovery platform for medicinal chemists, and on the need to identify metallodrug target sites, one of the key areas for advancement towards more clinical approvals. However, new procedures are needed for metallodrugs compared to screens for organic drugs. Metallodrugs are usually prodrugs. They can undergo ligand exchange and redox reactions on their way to or at target sites. Furthermore, they are often multitargeting. Cell culture media contain a complicated mixture of components, sometimes ill-defined and variable, which can influence the behaviour of metallodrugs. The synthesised metal complex may be readily transformed into new species in the culture media, or even in the DMSO often used as a universal solubilising medium. However, with modern physical techniques, the nature of the species in culture media and even in cells can now be investigated. In addition, the instability of metallodrugs can be turned to an advantage if their activation (e.g. by hydrolysis, metal or ligand-based redox reactions, external forces such as photo-irradiation) can be controlled.
The need to select carefully the screening procedure for metallodrugs is highlighted by the anticancer activity of oxaliplatin and some other PtIV complexes in solid tumour models being dependent on an intact immune system. Moreover, the efficacy and toxicity of oxaliplatin is dependent on stages of the cell cycle and the body clock (day or night administration).
The unique properties of alpha-, beta- or gamma-emitting radioactive metals, their increased availability for therapy, diagnosis and tracing, and reliable methods for their chelation and conjugation to vectors delivering them to receptors and other targets is an exciting area of current research. Omics (genomics, proteomics, and metallomics) technologies now allow exploration of the multiple pathways of metallodrug actions. They have revealed, for example, that despite the structural similarity of the clinical drugs cisplatin, carboplatin and oxaliplatin, the latter targets ribosome biogenesis and not genomic DNA.237 The combined application of hyphenated chromatography-mass spectrometry methods can reveal specific target sites for metallodrugs on proteins and other targets. Use of the wide range of energies of electromagnetic radiation from gamma and X-rays, to UV-vis and IR to micro- and radio-waves, allows the characteristic properties of metal complexes to be used for detection and imaging of metallodrugs and their metabolites in intact biological samples, including frozen cells.
The uniqueness of the roles of metals in drugs has been illustrated here with examples especially from anticancer and antimicrobial agents. Although not discussed here, salts of essential metals such as Na, K, Mg and Ca (e.g. see Table 2 in ref. 2) are used daily on a massive scale in a variety of medicines and clearly can also not be replaced by organic compounds. In addition to metallodrugs, metal ions may be involved in the mechanisms of action for organic drugs, since these often contain up to 10 H-bond acceptors (a Lipinski rule) which will also act as metal-binding sites.
Metallodrugs are challenging to design and develop. However, their different and unique properties provide invaluable opportunities for the discovery of drugs with novel mechanisms of action, able to address the ever-complex medical challenges that our world is facing.
Conflicts of interest
There are no conflicts to declare.
Acknowledgements
We thank the Biotechnology and Biological Sciences Research Council, BBSRC (EJA), the Warwick Collaborative Postgraduate Research Scholarship (EMB), Engineering Physical Sciences Research Council EPSRC (studentships for EMB, HEB, OWLC, ECL, and grants EP/N033191/1 and EP/P030572/1), Diamond Light Source (EMB), the Global Challenges Research Fund (HEB) the US-UK Fulbright Commission (JMD), the Wellcome Trust (209173/Z/17/Z, CI), the LOEWE project TRABITA funded by the Ministry of Higher Education, Research and the Arts (HMWK) of the state of Hesse (FL) the Warwick EU Chancellor's Scholarship (MP) Warwick Chancellor's Scholarship (WZ) and the Chinese Scholarship Council, CSC (FXW and ZZ). We also acknowledge support from Anglo American Platinum (RJN, HS), Bruker (ECL) and GoldenKeys High-tech Materials Co., Ltd (OWLC).
References
- N. C. Lloyd, H. W. Morgan, B. K. Nicholson and R. S. Ronimus, Angew. Chem., Int. Ed., 2005, 44, 941–944 CrossRef CAS.
-
C. Imberti and P. J. Sadler, in Advances in Inorganic Chemistry, ed. P. J. Sadler and R. van Eldik, Academic Press, 2020, vol. 75, pp. 3–56 Search PubMed.
- A. Frei, J. Zuegg, A. G. Elliott, M. Baker, S. Braese, C. Brown, F. Chen, C. G. Dowson, G. Dujardin, N. Jung, A. P. King, A. M. Mansour, M. Massi, J. Moat, H. A. Mohamed, A. K. Renfrew, P. J. Rutledge, P. J. Sadler, M. H. Todd, C. E. Willans, J. J. Wilson, M. A. Cooper and M. A. T. Blaskovich, Chem. Sci., 2020, 11, 2627–2639 RSC.
- C. A. Lipinski, F. Lombardo, B. W. Dominy and P. J. Feeney, Adv. Drug Deliv. Rev., 2001, 46, 3–26 CrossRef CAS.
- K. D. Mjos and C. Orvig, Chem. Rev., 2014, 114, 4540–4563 CrossRef CAS.
- C. N. Morrison, K. E. Prosser, R. W. Stokes, A. Cordes, N. Metzler-Nolte and S. M. Cohen, Chem. Sci., 2020, 11, 1216–1225 RSC.
- A. Y. Chen, R. N. Adamek, B. L. Dick, C. V. Credille, C. N. Morrison and S. M. Cohen, Chem. Rev., 2019, 119, 1323–1455 CrossRef CAS.
- R. R. Ye, C. P. Tan, B. C. Chen, R. T. Li and Z. W. Mao, Front. Chem., 2020, 8, 402 CrossRef CAS.
- R. M. Wang, T. P. Lai, P. Gao, H. M. Zhang, P. L. Ho, P. C. Y. Woo, G. X. Ma, R. Y. T. Kao, H. Y. Li and H. Z. Sun, Nat. Commun., 2018, 9, 439 CrossRef.
-
R. J. Needham and P. J. Sadler, in The Periodic Table II: Catalytic, Materials, Biological and Medical Applications, ed. D. M. P. Mingos, Springer International Publishing, Cham, 2019, pp. 175–201 Search PubMed.
-
F. Arnesano, M. Losacco and G. Natile, in Binding, Transport and Storage of Metal Ions in Biological Cells, ed. W. Maret and A. Wedd, The Royal Society of Chemistry, 2014, pp. 429–460 Search PubMed.
-
A. Pizarro, A. Habtemariam and P. Sadler, in Medicinal Organometallic Chemistry, ed. G. Jaouen and N. Metzler-Nolte, Springer, Berlin/Heidelberg, 2010, vol. 32, pp. 21–56 Search PubMed.
- S. J. Berners-Price, T. A. Frenkiel, U. Frey, J. D. Ranford and P. J. Sadler, J. Chem. Soc., Chem. Commun., 1992, 789–791 RSC.
- A. Mandic, J. Hansson, S. Linder and M. C. Shoshan, J. Biol. Chem., 2003, 278, 9100–9106 CrossRef CAS.
- Z. H. Siddik, Oncogene, 2003, 22, 7265–7279 CrossRef CAS.
- E. Volckova, L. Dudones and R. Bose, Pharm. Res., 2002, 19, 124–131 CrossRef CAS.
- H. H. W. Chen and M. T. Kuo, Met.-Based Drugs, 2010, 2010, 430939 Search PubMed.
- F. Martinez, G. Deray, M. Dubois, H. Beaufils, C. Jacquiaud, R. Bourbouze, M. Benhmida, M.-C. Jaudon and C. Jacobs, Anti-Cancer Drugs, 1993, 4, 85–90 CrossRef CAS.
- G. Lümmen, H. Sperling, H. Luboldt, T. Otto and H. Rübben, Cancer Chemother. Pharmacol., 1998, 42, 415–417 CrossRef.
- J. H. Toney and T. J. Marks, J. Am. Chem. Soc., 1985, 107, 947–953 CrossRef CAS.
- M. L. McLaughlin, J. M. Cronan Jr, T. R. Schaller and R. D. Snelling, J. Am. Chem. Soc., 1990, 112, 8949–8952 CrossRef CAS.
- M. Guo, H. Sun, H. J. McArdle, L. Gambling and P. J. Sadler, Biochemistry, 2000, 39, 10023–10033 CrossRef CAS.
-
E. Y. Tshuva and M. Miller, in Coordination Complexes of Titanium(IV) for Anticancer Therapy, ed. A. Sigel, H. Sigel, E. Freisinger and R. K. O. Sigel, De Gruyter, Berlin, 2018, ch. 8, vol. 18, pp. 219–250 Search PubMed.
- F. Wang, J. J. Xu, A. Habtemariam, J. Bella and P. J. Sadler, J. Am. Chem. Soc., 2005, 127, 17734–17743 CrossRef CAS.
- F. Wang, A. Habtemariam, E. P. L. van der Geer, R. Fernández, M. Melchart, R. J. Deeth, R. Aird, S. Guichard, F. P. A. Fabbiani, P. Lozano-Casal, I. D. H. Oswald, D. I. Jodrell, S. Parsons and P. J. Sadler, Proc. Natl. Acad. Sci. U. S. A., 2005, 102, 18269–18274 CrossRef CAS.
- A. F. A. Peacock, A. Habtemariam, R. Fernández, V. Walland, F. P. A. Fabbiani, S. Parsons, R. E. Aird, D. I. Jodrell and P. J. Sadler, J. Am. Chem. Soc., 2006, 128, 1739–1748 CrossRef CAS.
- W. F. Schmid, R. O. John, G. Mühlgassner, P. Heffeter, M. A. Jakupec, M. Galanski, W. Berger, V. B. Arion and B. K. Keppler, J. Med. Chem., 2007, 50, 6343–6355 CrossRef CAS.
- Y. Fu, A. Habtemariam, A. M. Pizarro, S. H. van Rijt, D. J. Healey, P. A. Cooper, S. D. Shnyder, G. J. Clarkson and P. J. Sadler, J. Med. Chem., 2010, 53, 8192–8196 CrossRef CAS.
- R. J. Needham, C. Sanchez-Cano, X. Zhang, I. Romero-Canelón, A. Habtemariam, M. S. Cooper, L. Meszaros, G. J. Clarkson, P. J. Blower and P. J. Sadler, Angew. Chem., Int. Ed., 2017, 56, 1017–1020 CrossRef CAS.
- I. Romero-Canelón, M. Mos and P. J. Sadler, J. Med. Chem., 2015, 58, 7874–7880 CrossRef.
- Y. Fu, M. J. Romero, A. Habtemariam, M. E. Snowden, L. Song, G. J. Clarkson, B. Qamar, A. M. Pizarro, P. R. Unwin and P. J. Sadler, Chem. Sci., 2012, 3, 2485–2494 RSC.
- M. J. Chow, C. Licona, D. Y. Qiang Wong, G. Pastorin, C. Gaiddon and W. H. Ang, J. Med. Chem., 2014, 57, 6043–6059 CrossRef CAS.
- L. Helm and A. E. Merbach, Coord. Chem. Rev., 1999, 187, 151–181 CrossRef CAS.
- L. Messori, G. Marcon, P. Orioli, M. Fontani, P. Zanello, A. Bergamo, G. Sava and P. Mura, J. Inorg. Biochem., 2003, 95, 37–46 CrossRef CAS.
- T. Poth, H. Paulus, H. Elias, C. Dücker-Benfer and R. van Eldik, Eur. J. Inorg. Chem., 2001, 2001, 1361–1369 CrossRef.
- Z. Liu and P. J. Sadler, Acc. Chem. Res., 2014, 47, 1174–1185 CrossRef CAS.
- J. J. Soldevila-Barreda, A. Habtemariam, I. Romero-Canelón and P. J. Sadler, J. Inorg. Biochem., 2015, 153, 322–333 CrossRef CAS.
- Z. Liu, A. Habtemariam, A. M. Pizarro, S. A. Fletcher, A. Kisova, O. Vrana, L. Salassa, P. C. A. Bruijnincx, G. J. Clarkson, V. Brabec and P. J. Sadler, J. Med. Chem., 2011, 54, 3011–3026 CrossRef CAS.
- S. Banerjee, J. J. Soldevila-Barreda, J. A. Wolny, C. A. Wootton, A. Habtemariam, I. Romero-Canelón, F. Chen, G. J. Clarkson, I. Prokes, L. Song, P. B. O'Connor, V. Schünemann and P. J. Sadler, Chem. Sci., 2018, 9, 3177–3185 RSC.
- Z. Liu, A. Habtemariam, A. M. Pizarro, G. J. Clarkson and P. J. Sadler, Organometallics, 2011, 30, 4702–4710 CrossRef CAS.
- W.-Y. Zhang, S. Banerjee, G. M. Hughes, H. E. Bridgewater, J.-I. Song, B. G. Breeze, G. J. Clarkson, J. P. C. Coverdale, C. Sanchez-Cano, F. Ponte, E. Sicilia and P. J. Sadler, Chem. Sci., 2020, 11, 5466–5480 RSC.
- Z. Liu, I. Romero-Canelón, B. Qamar, J. M. Hearn, A. Habtemariam, N. P. E. Barry, A. M. Pizarro, G. J. Clarkson and P. J. Sadler, Angew. Chem., Int. Ed., 2014, 53, 3941–3946 CrossRef CAS.
- A. C. Carrasco, V. Rodríguez-Fanjul, A. Habtemariam and A. M. Pizarro, J. Med. Chem., 2020, 63, 4005–4021 CrossRef CAS.
- V. Purohit, D. M. Simeone and C. A. Lyssiotis, Cancers, 2019, 11, 955 CrossRef CAS.
- N. Graf and S. J. Lippard, Adv. Drug Deliv. Rev., 2012, 64, 993–1004 CrossRef CAS.
- I. Romero-Canelón and P. J. Sadler, Inorg. Chem., 2013, 52, 12276–12291 CrossRef.
- P. Zhang and P. J. Sadler, Eur. J. Inorg. Chem., 2017, 2017, 1541–1548 CrossRef CAS.
- J. M. Brown, Cancer Res., 1999, 59, 5863–5870 CAS.
- D. Gibson, J. Inorg. Biochem., 2019, 191, 77–84 CrossRef CAS.
- N. J. Wheate, S. Walker, G. E. Craig and R. Oun, Dalton Trans., 2010, 39, 8113–8127 RSC.
- J. Karges, T. Yempala, M. Tharaud, D. Gibson and G. Gasser, Angew. Chem., Int. Ed., 2020, 59, 7069–7075 CrossRef CAS.
- A. Bergamo, C. Gaiddon, J. H. Schellens, J. H. Beijnen and G. Sava, J. Inorg. Biochem., 2012, 106, 90–99 CrossRef CAS.
- A. K. Renfrew, N. S. Bryce and T. W. Hambley, Chem. Sci., 2013, 4, 3731–3739 RSC.
- S. J. Dougan, A. Habtemariam, S. E. McHale, S. Parsons and P. J. Sadler, Proc. Natl. Acad. Sci. U. S. A., 2008, 105, 11628–11633 CrossRef CAS.
- K. C. Tong, C. N. Lok, P. K. Wan, D. Hu, Y. M. E. Fung, X. Y. Chang, S. Huang, H. Jiang and C. M. Che, Proc. Natl. Acad. Sci. U. S. A., 2020, 117, 1321–1329 CrossRef CAS.
- M. Patra and G. Gasser, Nat. Rev. Chem., 2017, 1, 0066 CrossRef CAS.
- E. Hillard, A. Vessières, L. Thouin, G. Jaouen and C. Amatore, Angew. Chem., Int. Ed., 2005, 45, 285–290 CrossRef.
- F. Dubar, J. Khalife, J. Brocard, D. Dive and C. Biot, Molecules, 2008, 13, 2900–2907 CrossRef CAS.
- C. Mu, K. E. Prosser, S. Harrypersad, G. A. MacNeil, R. Panchmatia, J. R. Thompson, S. Sinha, J. J. Warren and C. J. Walsby, Inorg. Chem., 2018, 57, 15247–15261 CrossRef CAS.
- Y. Lin, Y. Huang, W. Zheng, F. Wang, A. Habtemariam, Q. Luo, X. Li, K. Wu, P. J. Sadler and S. Xiong, J. Inorg. Biochem., 2013, 128, 77–84 CrossRef CAS.
- N. A. Kratochwil, Z. J. Guo, P. D. S. Murdoch, J. A. Parkinson, P. J. Bednarski and P. J. Sadler, J. Am. Chem. Soc., 1998, 120, 8253–8254 CrossRef CAS.
- Q. Chen, H. Dan, F. Tang, J. Wang, X. Li, J. Cheng, H. Zhao and X. Zeng, Int. J. Oral Sci., 2019, 11, 14 CrossRef.
- L. Benov, Med. Princ. Pract., 2015, 24, 14–28 CrossRef.
- E. Skovsen, J. W. Snyder, J. D. C. Lambert and P. R. Ogilby, J. Phys. Chem. B, 2005, 109, 8570–8573 CrossRef CAS.
- I. Ashur, R. Goldschmidt, I. Pinkas, Y. Salomon, G. Szewczyk, T. Sarna and A. Scherz, J. Phys. Chem. A, 2009, 113, 8027–8037 CrossRef CAS.
-
B. Rodríguez-Amigo, O. Planas, R. Bresolí-Obach, J. Torra, R. Ruiz-González, and S. Nonell, in Photodynamic Medicine: FromBench to Clinic, ed. H. Kostron and T. Hasan, Royal Society of Chemistry, Cambridge, 2016 Search PubMed.
- S. A. McFarland, A. Mandel, R. Dumoulin-White and G. Gasser, Curr. Opin. Chem. Biol., 2020, 56, 23–27 CrossRef CAS.
- A. R. Rastinehad, H. Anastos, E. Wajswol, J. S. Winoker, J. P. Sfakianos, S. K. Doppalapudi, M. R. Carrick, C. J. Knauer, B. Taouli, S. C. Lewis, A. K. Tewari, J. A. Schwartz, S. E. Canfield, A. K. George, J. L. West and N. J. Halas, Proc. Natl. Acad. Sci. U. S. A., 2019, 116, 18590–18596 CrossRef CAS.
- A. Leonidova, V. Pierroz, R. Rubbiani, Y. Lan, A. G. Schmitz, A. Kaech, R. K. O. Sigel, S. Ferrari and G. Gasser, Chem. Sci., 2014, 5, 4044–4056 RSC.
- S. Lazic, P. Kaspler, G. Shi, S. Monro, T. Sainuddin, S. Forward, K. Kasimova, R. Hennigar, A. Mandel, S. McFarland and L. Lilge, Photochem. Photobiol., 2017, 93, 1248–1258 CrossRef CAS.
- P. Zhang, H. Huang, S. Banerjee, G. J. Clarkson, C. Ge, C. Imberti and P. J. Sadler, Angew. Chem., Int. Ed., 2019, 58, 2350–2354 CrossRef CAS.
- A. Presa, G. Vázquez, L. A. Barrios, O. Roubeau, L. Korrodi-Gregório, R. Pérez-Tomás and P. Gamez, Inorg. Chem., 2018, 57, 4009–4022 CrossRef CAS.
- A. C. V. Doughty, A. R. Hoover, E. Layton, C. K. Murray, E. W. Howard and W. R. Chen, Materials, 2019, 12, 779 Search PubMed.
- Z. Li, Y. Chen, Y. Yang, Y. Yu, Y. Zhang, D. Zhu, X. Yu, X. Ouyang, Z. Xie, Y. Zhao and L. Li, Front. Bioeng. Biotechnol., 2019, 7, 293 CrossRef.
- S. Bonnet, Dalton Trans., 2018, 47, 10330–10343 RSC.
- C. Imberti, P. Zhang, H. Huang and P. J. Sadler, Angew. Chem., Int. Ed., 2020, 59, 61–73 CrossRef CAS.
- H. Shi, C. Imberti and P. J. Sadler, Inorg. Chem. Front., 2019, 6, 1623–1638 RSC.
- S. Xu, X. Zhu, C. Zhang, W. Huang, Y. Zhou and D. Yan, Nat. Commun., 2018, 9, 2053 CrossRef.
- B. Gu, W. B. Wu, G. X. Xu, G. X. Feng, F. Yin, P. H. J. Chong, J. L. Qu, K. T. Yong and B. Liu, Adv. Mater., 2017, 29, 1701076 CrossRef.
- M. R. Gill and K. A. Vallis, Chem. Soc. Rev., 2019, 48, 540–557 RSC.
- Z. Zhao, P. Gao, L. Ma and T. Chen, Chem. Sci., 2020, 11, 3780–3789 RSC.
- J. Rousseau, R. F. Barth, M. Fernandez, J. F. Adam, J. Balosso, F. Esteve and H. Elleaume, J. Neuro-Oncol., 2010, 98, 287–295 CrossRef CAS.
- L. Larue, A. Ben Mihoub, Z. Youssef, L. Colombeau, S. Acherar, J. C. Andre, P. Arnoux, F. Baros, M. Vermandel and C. Frochot, Photochem. Photobiol. Sci., 2018, 17, 1612–1650 RSC.
- A. Karnkaew, F. Chen, Y. Zhan, R. L. Majewski and W. Cai, ACS Nano, 2016, 10, 3918–3935 CrossRef.
- M. Y. Yang, T. Yang and C. B. Mao, Angew. Chem., Int. Ed., 2019, 58, 14066–14080 CrossRef CAS.
- I. Rosenthal, J. Z. Sostaric and P. Riesz, Ultrason. Sonochem., 2004, 11, 349–363 CAS.
- X. Pan, L. Bai, H. Wang, Q. Wu, H. Wang, S. Liu, B. Xu, X. Shi and H. Liu, Adv. Mater., 2018, 30, 1800180 CrossRef.
- V. Choi, M. A. Rajora and G. Zheng, Bioconjugate Chem., 2020, 31, 967–989 CrossRef CAS.
- C. M. Clavel, P. Nowak-Sliwinska, E. Paunescu, A. W. Griffioen and P. J. Dyson, Chem. Sci., 2015, 6, 2795–2801 RSC.
- J. E. Garrett, E. Metzger, K. Schmitt, S. Soto, S. Northern, L. Kryah, M. Irfan, S. Rice, M. Brown, J. M. Zaleski and J. R. Dynlacht, Radiat. Res., 2020, 193, 107–118 CAS.
- E. J. Keller, M. Porter, J. E. Garrett, M. Varie, H. Wang, K. E. Pollok, J. J. Turchi, J. M. Zaleski and J. R. Dynlacht, Radiat. Res., 2018, 190, 107–116 CrossRef CAS.
- R. Carter, A. Westhorpe, M. J. Romero, A. Habtemariam, C. R. Gallevo, Y. Bark, N. Menezes, P. J. Sadler and R. A. Sharma, Sci. Rep., 2016, 6, 20596 CrossRef CAS.
- J. A. Ohara, E. B. Douple, M. J. Abrams, D. J. Picker, C. M. Giandomenico and J. F. Vollano, Int. J. Radiat. Oncol., Biol., Phys., 1989, 16, 1049–1052 CrossRef CAS.
- D. G. You, V. G. Deepagan, W. Um, S. Jeon, S. Son, H. Chang, H. I. Yoon, Y. W. Cho, M. Swierczewska, S. Lee, M. G. Pomper, I. C. Kwon, K. Kim and J. H. Park, Sci. Rep., 2016, 6, 12 CrossRef.
- H. Abe, M. Kuroki, K. Tachibana, T. L. Li, A. Awasthi, A. Ueno, H. Matsumoto, T. Imakiire, Y. Yamauchi, H. Yamada, A. Ariyoshi and M. Kuroki, Anticancer Res., 2002, 22, 1575–1580 CAS.
- J. J. Soldevila-Barreda and N. Metzler-Nolte, Chem. Rev., 2019, 119, 829–869 CrossRef CAS.
- J. J. Soldevila-Barreda and P. J. Sadler, Curr. Opin. Chem. Biol., 2015, 25, 172–183 CrossRef CAS.
- W. Ying, Antioxid. Redox Signaling, 2007, 10, 179–206 CrossRef.
- Y. Zhao, A. Wang, Y. Zou, N. Su, J. Loscalzo and Y. Yang, Nat. Protoc., 2016, 11, 1345–1359 CrossRef CAS.
- F. Chen, J. J. Soldevila-Barreda, I. Romero-Canelón, J. P. C. Coverdale, J.-I. Song, G. J. Clarkson, J. Kasparkova, A. Habtemariam, V. Brabec, J. A. Wolny, V. Schuenemann and P. J. Sadler, Dalton Trans., 2018, 47, 7178–7189 RSC.
- J. J. Soldevila-Barreda, P. C. A. Bruijnincx, A. Habtemariam, G. J. Clarkson, R. J. Deeth and P. J. Sadler, Organometallics, 2012, 31, 5958–5967 CrossRef CAS.
- W.-Y. Zhang, H. E. Bridgewater, S. Banerjee, J. J. Soldevila-Barreda, G. J. Clarkson, H. Shi, C. Imberti and P. J. Sadler, Eur. J. Inorg. Chem., 2020, 2020, 1052–1060 CrossRef CAS.
- J. J. Soldevila-Barreda, I. Romero-Canelón, A. Habtemariam and P. J. Sadler, Nat. Commun., 2015, 6, 6582 CrossRef CAS.
- J. P. C. Coverdale, I. Romero-Canelón, C. Sanchez-Cano, G. J. Clarkson, A. Habtemariam, M. Wills and P. J. Sadler, Nat. Chem., 2018, 10, 347–354 CrossRef CAS.
- S. Betanzos-Lara, Z. Liu, A. Habtemariam, A. M. Pizarro, B. Qamar and P. J. Sadler, Angew. Chem., Int. Ed., 2012, 51, 3897–3900 CrossRef CAS.
- H. Huang, S. Banerjee, K. Qiu, P. Zhang, O. Blacque, T. Malcomson, M. J. Paterson, G. J. Clarkson, M. Staniforth, V. G. Stavros, G. Gasser, H. Chao and P. J. Sadler, Nat. Chem., 2019, 11, 1041–1048 CrossRef CAS.
- C. Hwang, A. J. Sinskey and H. F. Lodish, Science, 1992, 257, 1496 CrossRef CAS.
- P. Starha, A. Habtemariam, I. Romero-Canelón, G. J. Clarkson and P. J. Sadler, Inorg. Chem., 2016, 55, 2324–2331 CrossRef CAS.
- J. S. Derrick, J. Lee, S. J. C. Lee, Y. Kim, E. Nam, H. Tak, J. Kang, M. Lee, S. H. Kim, K. Park, J. Cho and M. H. Lim, J. Am. Chem. Soc., 2017, 139, 2234–2244 CrossRef CAS.
- Z. Yu, M. Han and J. A. Cowan, Angew. Chem., Int. Ed., 2015, 54, 1901–1905 CrossRef CAS.
- Z. Yu and J. A. Cowan, Curr. Opin. Chem. Biol., 2018, 43, 37–42 CrossRef CAS.
- L. Feng, Y. Geisselbrecht, S. Blanck, A. Wilbuer, G. E. Atilla-Gokcumen, P. Filippakopoulos, K. Kraling, M. A. Celik, K. Harms, J. Maksimoska, R. Marmorstein, G. Frenking, S. Knapp, L. O. Essen and E. Meggers, J. Am. Chem. Soc., 2011, 133, 5976–5986 CrossRef CAS.
- E. Meggers, Chem. Commun., 2009, 1001–1010 RSC.
- J. G. Moffat, J. Rudolph and D. Bailey, Nat. Rev. Drug Discovery, 2014, 13, 588–602 CrossRef CAS.
- A. Levina, D. C. Crans and P. A. Lay, Coord. Chem. Rev., 2017, 352, 473–498 CrossRef CAS.
- M. D. Hall, K. A. Telma, K. E. Chang, T. D. Lee, J. P. Madigan, J. R. Lloyd, I. S. Goldlust, J. D. Hoeschele and M. M. Gottesman, Cancer Res., 2014, 74, 3913–3922 CrossRef CAS.
- K. Oettl and R. Stauber, Br. J. Pharmacol., 2007, 151, 580–590 CrossRef CAS.
- H. Haase, S. Hebel, G. Engelhardt and L. Rink, Metallomics, 2015, 7, 102–111 RSC.
- C. A. Wootton, Y. P. Y. Lam, M. Willetts, M. A. van Agthoven, M. P. Barrow, P. J. Sadler and P. B. O'Connor, Analyst, 2017, 142, 2029–2037 RSC.
- F. Wang, H. Chen, S. Parsons, I. D. H. Oswald, J. E. Davidson and P. J. Sadler, Chem.–Eur. J., 2003, 9, 5810–5820 CrossRef CAS.
- E. Shaili, M. Fernández-Giménez, S. Rodríguez-Astor, A. Gandioso, L. Sandín, C. García-Vélez, A. Massaguer, G. J. Clarkson, J. A. Woods, P. J. Sadler and V. Marchán, Chem.–Eur. J., 2015, 21, 18474–18486 CrossRef CAS.
- M. Groessl, M. Terenghi, A. Casini, L. Elviri, R. Lobinski and P. J. Dyson, J. Anal. At. Spectrom., 2010, 25, 305–313 RSC.
- M. H. M. Klose, A. Schöberl, P. Heffeter, W. Berger, C. G. Hartinger, G. Koellensperger, S. M. Meier-Menches and B. K. Keppler, Monatsh. Chem., 2018, 149, 1719–1726 CrossRef CAS.
- U. Ndagi, N. Mhlongo and M. E. Soliman, Drug Des., Dev. Ther., 2017, 11, 599 CrossRef CAS.
- S. M. Meier-Menches, C. Gerner, W. Berger, C. G. Hartinger and B. K. Keppler, Chem. Soc. Rev., 2018, 47, 909–928 RSC.
- T. C. Johnstone, K. Suntharalingam and S. J. Lippard, Chem. Rev., 2016, 116, 3436–3486 CrossRef CAS.
- N. Muhammad and Z. J. Guo, Curr. Opin. Chem. Biol., 2014, 19, 144–153 CrossRef CAS.
- A. Notaro and G. Gasser, Chem. Soc. Rev., 2017, 46, 7317–7337 RSC.
- C. H. Wong, K. W. Siah and A. W. Lo, Biostatistics, 2019, 20, 273–286 CrossRef.
-
Metallo-drugs: development and action of anticancer agents, ed. A. Sigel, H. Sigel, E. Freisinger and R. K. O. Sigel, Walter de Gruyter GmbH & Co KG, 2018 Search PubMed.
- C. S. Allardyce and P. J. Dyson, Dalton Trans., 2016, 45, 3201–3209 RSC.
- H. A. Burris, S. Bakewell, J. C. Bendell, J. Infante, S. F. Jones, D. R. Spigel, G. J. Weiss, R. K. Ramanathan, A. Ogden and D. Von Hoff, ESMO Open, 2016, 1, e000154 CrossRef.
- S. Monro, K. L. Colón, H. Yin, J. Roque III, P. Konda, S. Gujar, R. P. Thummel, L. Lilge, C. G. Cameron and S. A. McFarland, Chem. Rev., 2018, 119, 797–828 CrossRef.
- S. Akshintala, L. Marcus, K. E. Warren, R. F. Murphy, T. M. Sissung, A. Srivastava, W. J. Goodspeed, A. Goodwin, C. C. Brewer and C. Zalewski, Pediatr. Blood Cancer, 2015, 62, 603–610 CrossRef CAS.
-
I. Pötsch, D. Baier, B. K. Keppler and W. Berger, in Metal-based anticancer agents, 2019, pp. 308–347 Search PubMed.
-
C. Marmion, I. Romero-Canelón, A. Vessières, A. Kellett, C.-M. Che, M. Contel, D. Crans, S. Gibaud, A. Casini and S. M. Meier-Menches, Metal-based anticancer agents, Royal Society of Chemistry, 2019 Search PubMed.
- N. P. Farrell, Chem. Soc. Rev., 2015, 44, 8773–8785 RSC.
- A. E. Stacy, D. Palanimuthu, P. V. Bernhardt, D. S. Kalinowski, P. J. Jansson and D. R. Richardson, J. Med. Chem., 2016, 59, 4965–4984 CrossRef CAS.
- A. Van Tonder, A. M. Joubert and A. D. Cromarty, BMC Res. Notes, 2015, 8, 47 CrossRef.
- A. Ballesta, F. Billy, J. P. C. Coverdale, J. I. Song, C. Sanchez-Cano, I. Romero-Canelón and P. J. Sadler, Metallomics, 2019, 11, 1648–1656 RSC.
- M. S. Novak, G. E. Buchel, B. K. Keppler and M. A. Jakupec, J. Biol. Inorg Chem., 2016, 21, 347–356 CrossRef CAS.
- L. Zeng, P. Gupta, Y. Chen, E. Wang, L. Ji, H. Chao and Z.-S. Chen, Chem. Soc. Rev., 2017, 46, 5771–5804 RSC.
- L. Cai, X. J. Qin, Z. H. Xu, Y. Y. Song, H. J. Jiang, Y. Wu, H. J. Ruan and J. Chen, ACS Omega, 2019, 4, 12036–12042 CrossRef CAS.
- A. Alsalme, S. Laeeq, S. Dwivedi, M. S. Khan, K. Al Farhan, J. Musarrat and R. A. Khan, Spectrochim. Acta, Part A, 2016, 163, 1–7 CrossRef CAS.
- G. Fotakis and J. A. Timbrell, Toxicol. Lett., 2006, 160, 171–177 CrossRef CAS.
- B. H. Neufeld, J. B. Tapia, A. Lutzke and M. M. Reynolds, Anal. Chem., 2018, 90, 6867–6876 CrossRef CAS.
- N. A. P. Franken, H. M. Rodermond, J. Stap, J. Haveman and C. van Bree, Nat. Protoc., 2006, 1, 2315–2319 CrossRef CAS.
- M. Selby, R. Delosh, J. Laudeman, C. Ogle, R. Reinhart, T. Silvers, S. Lawrence, R. Kinders, R. Parchment and B. A. Teicher, SLAS Discovery, 2017, 22, 473–483 CAS.
- J. M. Hearn, G. M. Hughes, I. Romero-Canelón, A. F. Munro, B. Rubio-Ruiz, Z. Liu, N. O. Carragher and P. J. Sadler, Metallomics, 2018, 10, 93–107 RSC.
- G. Lazzari, P. Couvreur and S. Mura, Polym. Chem., 2017, 8, 4947–4969 RSC.
- E. Schreiber-Brynzak, E. Klapproth, C. Unger, I. Lichtscheidl-Schultz, S. Goschl, S. Schweighofer, R. Trondl, H. Dolznig, M. A. Jakupec and B. K. Keppler, Invest. New Drugs, 2015, 33, 835–847 CrossRef CAS.
- F. Yu, W. Hunziker and D. Choudhury, Micromachines, 2019, 10, 165 CrossRef.
- A. A. Saei, H. Gullberg, P. Sabatier, C. M. Beusch, K. Johansson, B. Lundgren, P. I. Arvidsson, E. S. J. Arner and R. A. Zubarev, Redox Biol., 2020, 32, 101491 CrossRef CAS.
- R. Puranik, S. Bao, A. M. Bonin, R. Kaur, J. E. Weder, L. Casbolt, T. W. Hambley, P. A. Lay, P. J. Barter and K. A. Rye, Cell Biosci., 2016, 6, 9 CrossRef.
- J. W. Zhang, A. Pitto-Barry, L. J. Shang and N. P. E. Barry, R. Soc. Open Sci., 2017, 4, 170786 CrossRef.
- G. Bondar, M. Cadeiras, N. Wisniewski, J. Maque, J. Chittoor, E. Chang, M. Bakir, C. Starling, K. Shahzad, P. Ping, E. Reed and M. Deng, PLoS One, 2014, 9, e115097 CrossRef.
- J. W. Alexander, Surg. Infect., 2009, 10, 289–292 CrossRef.
- D. J. Barillo, A. R. Barillo, S. Korn, K. Lam and P. S. Attar, Burns, 2017, 43, 1189–1194 CrossRef.
- M. A. Malik, S. A. Lone, M. Y. Wani, M. I. A. Talukdar, O. A. Dar, A. Ahmad and A. A. Hashmi, Bioorg. Chem., 2020, 98, 103771 CrossRef CAS.
- O. A. Dar, S. A. Lone, M. A. Malik, M. Y. Wani, M. I. A. Talukdar, A. S. Al-Bogami, A. A. Hashmi and A. Ahmad, Appl. Organomet. Chem., 2019, 33, e5128 CrossRef CAS.
- V. Uivarosi, Molecules, 2013, 18, 11153–11197 CrossRef CAS.
- R. M. Gandra, P. Mc Carron, M. F. Fernandes, L. S. Ramos, T. P. Mello, A. C. Aor, M. H. Branquinha, M. McCann, M. Devereux and A. L. S. Santos, Front. Microbiol., 2017, 8, 1257 CrossRef.
- M. Balouiri, M. Sadiki and S. K. Ibnsouda, J. Pharm. Anal., 2016, 6, 71–79 CrossRef.
-
W. A. R. Network, CDISC malaria therapeutic area data standard (TAUG-malaria), https://www.wwarn.org/working-together/partner-projects/cdisc-malaria-therapeutic-area-data-standard-taug-malaria, accessed 10/07/2020, 2020 Search PubMed.
- A. G. Bayih, A. Debnath, E. Mitre, C. D. Huston, B. Laleu, D. Leroy, B. Blasco, B. Campo, T. N. C. Wells, P. A. Willis, P. Sjo, W. C. Van Voorhis and D. R. Pillai, Clin. Microbiol. Rev., 2017, 30, 647–669 CrossRef CAS.
- O. Domarle, G. Blampain, H. Agnaniet, T. Nzadiyabi, J. Lebibi, J. Brocard, L. Maciejewski, C. Biot, A. J. Georges and P. Millet, Antimicrob. Agents Chemother., 1998, 42, 540–544 CrossRef CAS.
- A. Debnath, D. Parsonage, R. M. Andrade, C. He, E. R. Cobo, K. Hirata, S. Chen, G. Garcia-Rivera, E. Orozco, M. B. Martinez, S. S. Gunatilleke, A. M. Barrios, M. R. Arkin, L. B. Poole, J. H. McKerrow and S. L. Reed, Nat. Med., 2012, 18, 956–960 CrossRef CAS.
-
M. Navarro and G. Visbal, in Trace Metals and Infectious Diseases, ed. J. O. Nriagu and E. P. Skaar, The MIT Press, 2015, vol. 16, pp. 161–172 Search PubMed.
- J. A. Schwartz, E. K. Lium and S. J. Silverstein, J. Virol., 2001, 75, 4117–4128 CrossRef CAS.
- J. Wang, Y. Liu, K. Xu, Y. Qi, J. Zhong, K. Zhang, J. Li, E. Wang, Z. Wu and Z. Kang, ACS Appl. Mater. Interfaces, 2014, 6, 9785–9789 CrossRef CAS.
- N. Margiotta, A. Bergamo, G. Sava, G. Padovano, E. de Clercq and G. Natile, J. Inorg. Biochem., 2004, 98, 1385–1390 CrossRef CAS.
- P. J. Sadler and H. Sun, J. Chem. Soc., Dalton Trans., 1995, 1395–1401 RSC.
- N. Yang, J. A. Tanner, B. J. Zheng, R. M. Watt, M. L. He, L. Y. Lu, J. Q. Jiang, K. T. Shum, Y. P. Lin, K. L. Wong, M. C. Lin, H. F. Kung, H. Sun and J. D. Huang, Angew. Chem., Int. Ed., 2007, 46, 6464–6468 CrossRef CAS.
- F. Wu, S. Zhao, B. Yu, Y. M. Chen, W. Wang, Z. G. Song, Y. Hu, Z. W. Tao, J. H. Tian, Y. Y. Pei, M. L. Yuan, Y. L. Zhang, F. H. Dai, Y. Liu, Q. M. Wang, J. J. Zheng, L. Xu, E. C. Holmes and Y. Z. Zhang, Nature, 2020, 579, 265–269 CrossRef CAS.
- M. P. Lythgoe and P. Middleton, Trends Pharmacol. Sci., 2020, 41, 363–382 CrossRef CAS.
- A. J. Turner, J. A. Hiscox and N. M. Hooper, Trends Pharmacol. Sci., 2004, 25, 291–294 CrossRef CAS.
- P. Zhou, X.-L. Yang, X.-G. Wang, B. Hu, L. Zhang, W. Zhang, H.-R. Si, Y. Zhu, B. Li, C.-L. Huang, H.-D. Chen, J. Chen, Y. Luo, H. Guo, R.-D. Jiang, M.-Q. Liu, Y. Chen, X.-R. Shen, X. Wang, X.-S. Zheng, K. Zhao, Q.-J. Chen, F. Deng, L.-L. Liu, B. Yan, F.-X. Zhan, Y.-Y. Wang, G.-F. Xiao and Z.-L. Shi, Nature, 2020, 579, 270–273 CrossRef CAS.
- A. J. te Velthuis, S. H. van den Worm, A. C. Sims, R. S. Baric, E. J. Snijder and M. J. van Hemert, PLoS Pathog., 2010, 6, e1001176 CrossRef.
-
S. L. Warnes, Z. R. Little and C. W. Keevil, mBio, 2015, 6, e01697-15 Search PubMed.
- A. Ross, J. H. Choi, T. M. Hunter, C. Pannecouque, S. A. Moggach, S. Parsons, E. De Clercq and P. J. Sadler, Dalton Trans., 2012, 41, 6408–6418 RSC.
- H. A. Rothan, S. Stone, J. Natekar, P. Kumari, K. Arora and M. Kumar, Virology, 2020, 547, 7–11 CrossRef CAS.
- H. HogenEsch, D. T. O'Hagan and C. B. Fox, npj Vaccines, 2018, 3, 1–11 CrossRef.
- X. Li, M. A. Shenashen, X. Wang, A. Ito, A. Taniguchi and S. A. Ei-Safty, Sci. Rep., 2017, 7, 1–10 CrossRef.
- B. T. Priest, I. M. Bell and M. L. Garcia, Channels, 2008, 2, 87–93 CrossRef.
- J. Bowes, A. J. Brown, J. Hamon, W. Jarolimek, A. Sridhar, G. Waldron and S. Whitebread, Nat. Rev. Drug Discovery, 2012, 11, 909–922 CrossRef CAS.
-
Committee for Medicinal Products for Human Use, TOOKAD – Assessment report, EMA, 2017 Search PubMed.
- L. J. N. Bradley, K. J. Yarema, S. J. Lippard and J. M. Essigmann, Biochemistry, 1993, 32, 982–988 CrossRef CAS.
- B. J. S. Sanderson, L. R. Ferguson and W. A. Denny, Mutat. Res., 1996, 355, 59–70 CrossRef.
- B. Szikriszt, A. Poti, O. Pipek, M. Krzystanek, N. Kanu, J. Molnar, D. Ribli, Z. Szeltner, G. E. Tusnady, I. Csabai, Z. Szallasi, C. Swanton and D. Szuts, Genome Biol., 2016, 17, 99 CrossRef.
- N. Allgayer, R. A. de Campos, L. P. Facciola Gonzalez, M. d. A. Flores, R. R. Dihl and M. Lehmann, Food Chem. Toxicol., 2019, 133, 110782 CrossRef CAS.
- A. P. de Souza, M. Lehmann and R. R. Dihl, Drug Chem. Toxicol., 2017, 40, 410–415 CrossRef CAS.
-
OECD, OECD Guidelines for the Testing of Chemicals, Section 4, https://www.oecd-ilibrary.org/environment/oecd-guidelines-for-the-testing-of-chemicals-section-4-health-effects_20745788?page=2, accessed 10/07/2020, 2020 Search PubMed.
- S. Elmore, Toxicol. Pathol., 2007, 35, 495–516 CrossRef CAS.
- I. Paul, A. D. Chacko, I. Stasik, S. Busacca, N. Crawford, F. McCoy, N. McTavish, B. Wilson, M. Barr, K. J. O'Byrne, D. B. Longley and D. A. Fennell, Cell Death Dis., 2012, 3, e449 CrossRef CAS.
- D. Wernitznig, K. Kiakos, G. Del Favero, N. Harrer, H. Machat, A. Osswald, M. A. Jakupec, A. Wernitznig, W. Sommergruber and B. Keppler, Metallomics, 2019, 11, 1044–1048 RSC.
- S. J. Bains, H. Abrahamsson, K. Flatmark, S. Dueland, K. H. Hole, T. Seierstad, K. R. Redalen, S. Meltzer and A. H. Ree, Cancer Immunol. Immunother., 2020, 69, 355–364 CrossRef CAS.
- F. Sun, L. Cui, T. Li, S. Chen, J. Song and D. Li, J. Recept. Signal Transduct., 2019, 39, 208–214 CrossRef CAS.
- S. Wu, C. Lu, Y. Chen, F. Lo, T. Wang, Y. Chen, S. Yuan, W. Liaw and Y. Wang, Inorg. Chem., 2016, 55, 9383–9392 CrossRef CAS.
- F. G. Parsa, M. A. H. Feizi, R. Safaralizadeh, S. A. Hosseini-Yazdi and M. Mahdavi, J. Biol. Inorg. Chem., 2020, 25, 383–394 CrossRef CAS.
- S. Chatterjee, S. Kundu, A. Bhattacharyya, C. G. Hartinger and P. J. Dyson, J. Biol. Inorg. Chem., 2008, 13, 1149–1155 CrossRef CAS.
- X. Wei, Y. Yang, J. Ge, X. Lin, D. Liu, S. Wang, J. Zhang, G. Zhou and S. Li, J. Inorg. Biochem., 2020, 202, 110857 CrossRef CAS.
- S. H. van Rijt, I. Romero-Canelón, Y. Fu, S. D. Shnyder and P. J. Sadler, Metallomics, 2014, 6, 1014–1022 RSC.
- V. Venkatesh, R. Berrocal-Martin, C. J. Wedge, I. Romero-Canelón, C. Sanchez-Cano, J. I. Song, J. P. C. Coverdale, P. Zhang, G. J. Clarkson, A. Habtemariam, S. W. Magennis, R. J. Deeth and P. J. Sadler, Chem. Sci., 2017, 8, 8271–8278 RSC.
- I. L. Cregan, A. M. Dharmarajan and S. A. Fox, Int. J. Oncol., 2013, 42, 444–452 CrossRef CAS.
- B. Shen, W. Mao, J. C. Ahn, P. S. Chung and P. He, Mol. Med. Rep., 2018, 18, 4978–4986 CAS.
- I. Marmol, P. Castellnou, R. Alvarez, M. C. Gimeno, M. J. Rodriguez-Yoldi and E. Cerrada, Eur. J. Med. Chem., 2019, 183, 111661 CrossRef CAS.
- J. Liu, W. Guo, J. Li, X. Li, J. Geng, Q. Chen and J. Gao, Int. J. Mol. Med., 2015, 35, 607–616 CrossRef CAS.
- D. J. Goussetis, J. K. Altman, H. Glaser, J. L. McNeer, M. S. Tallman and L. C. Platanias, J. Biol. Chem., 2010, 285, 29989–29997 CrossRef CAS.
- W. Guo, Y. Zhang, L. Zhang, B. Huang, F. Tao, W. Chen, Z. Guo, Q. Xu and Y. Sun, Autophagy, 2013, 9, 996–1008 CrossRef CAS.
- K. Xiong, C. Qian, Y. Yuan, L. Wei, X. Liao, L. He, T. W. Rees, Y. Chen, J. Wan, L. Ji and H. Chao, Angew. Chem., Int. Ed., 2020, 59, 16631–16637 CrossRef CAS.
- S. R. Yoshii and N. Mizushima, Int. J. Mol. Sci., 2017, 18, 1865 CrossRef.
- R. Chiarelli and M. C. Roccheri, Cells, 2012, 1, 597–616 CrossRef CAS.
- G. Kroemer, L. Galluzzi, P. Vandenabeele, J. Abrams, E. Alnemri, E. Baehrecke, M. Blagosklonny, W. El-Deiry, P. Golstein, D. Green, M. Hengartner, R. Knight, S. Kumar, S. Lipton, W. Malorni, G. Nuñez, M. Peter, J. Tschopp, J. Yuan, M. Piacentini, B. Zhivotovsky and G. Melino, Cell Death Differ., 2009, 16, 3–11 CrossRef CAS.
- J. Zhou, G. Wang, Y. Chen, H. Wang, Y. Hua and Z. Cai, Int. J. Mol. Med., 2019, 23, 4854–4865 Search PubMed.
- S. Goschl, E. Schreiber-Brynzak, V. Pichler, K. Cseh, P. Heffeter, U. Jungwirth, M. A. Jakupec, W. Berger and B. K. Keppler, Metallomics, 2017, 9, 309–322 RSC.
- B. Englinger, C. Pirker, P. Heffeter, A. Terenzi, C. R. Kowol, B. K. Keppler and W. Berger, Chem. Rev., 2019, 119, 1519–1624 CrossRef CAS.
- V. Velma, S. R. Dasari and P. B. Tchounwou, Biomark. Insights, 2016, 11, 113–121 CAS.
- N. Sarin, F. Engel, G. V. Kalayda, M. Mannewitz, J. Cinatl Jr, F. Rothweiler, M. Michaelis, H. Saafan, C. A. Ritter, U. Jaehde and R. Frotschl, PLoS One, 2017, 12, e0181081 CrossRef.
- S. William-Faltaos, D. Rouillard, P. Lechat and G. Bastian, Fundam. Clin. Pharmacol., 2007, 21, 165–172 CrossRef CAS.
- J. M. Hearn, I. Romero-Canelón, A. F. Munro, Y. Fu, A. M. Pizarro, M. J. Garnett, U. McDermott, N. O. Carragher and P. J. Sadler, Proc. Natl. Acad. Sci. U. S. A., 2015, 112, E3800–E3805 CrossRef CAS.
- S. Liu, J. Zhu, H. Xu, Y. Wang, Y. Liu, J. Liang, G. Zhang, D. Cao, Y. Lin, Y. Wu and Q. Guo, Spectrochim. Acta, Part A, 2016, 161, 77–82 CrossRef CAS.
- J. P. Coverdale, T. Laroiya-McCarron and I. Romero-Canelón, Inorganics, 2019, 7, 31 CrossRef CAS.
- V. Brabec, S. E. Howson, R. A. Kaner, R. M. Lord, J. Malina, R. M. Phillips, Q. M. Abdallah, P. C. McGowan, A. Rodger and P. Scott, Chem. Sci., 2013, 4, 4407–4416 RSC.
- F. Levi, A. Okyar, S. Dulong, P. F. Innominato and J. Clairambault, Annu. Rev. Pharmacol. Toxicol., 2010, 50, 377–421 CrossRef CAS.
- X. Li, G. Metzger, E. Filipski, N. Boughattas, G. Lemaigre, B. Hecquet, J. Filipski and F. Levi, Toxicol. Appl. Pharmacol., 1997, 143, 281–290 CrossRef CAS.
- P. P. Dakup, K. I. Porter, A. A. Little, R. P. Gajula, H. Zhang, E. Skornyakov, M. G. Kemp, H. P. Van Dongen and S. Gaddameedhi, Oncotarget, 2018, 9, 14524–14538 CrossRef.
- M. Oda, S. Koyanagi, Y. Tsurudome, T. Kanemitsu, N. Matsunaga and S. Ohdo, Mol. Pharmacol., 2014, 85, 715–722 CrossRef.
- T. Igarashi, H. Izumi, T. Uchiumi, K. Nishio, T. Arao, M. Tanabe, H. Uramoto, K. Sugio, K. Yasumoto and Y. Sasaguri, Oncogene, 2007, 26, 4749–4760 CrossRef CAS.
- Z.-l. Zeng, H.-y. Luo, J. Yang, W.-j. Wu, D.-l. Chen, P. Huang and R.-h. Xu, Clin. Cancer Res., 2014, 20, 1042–1052 CrossRef CAS.
-
K. Abraham, Q. Huang, H. Monje Xandri, A. Ballestra, C. Cano-Sanchez, S. Perrier, R. Dallmann, P. Sadler and F. Levi, XVI Congress of the European Biological Rhythms Society, Lyon, France, 2019, p. 126 Search PubMed.
- A. Schuster, H. Erasimus, S. Fritah, P. V. Nazarov, E. van Dyck, S. P. Niclou and A. Golebiewska, Trends Biotechnol., 2019, 37, 38–55 CrossRef CAS.
- A. Goodspeed, A. Jean and J. C. Costello, Eur. Urol., 2019, 75, 242–250 CrossRef CAS.
- Q. Ouyang, Y. Liu, J. Tan, J. Li, D. Yang, F. Zeng, W. Huang, Y. Kong, Z. Liu, H. Zhou and Y. Liu, Am. J. Cancer Res., 2019, 9, 988–998 CAS.
- T. Ko and S. Li, FASEB J., 2019, 33, 7143–7154 CrossRef CAS.
- D. Xu, S.-Q. Liang, H. Yang, R. Bruggmann, S. Berezowska, Z. Yang, T. M. Marti, S. R. R. Hall, Y. Gao, G. J. Kocher, R. A. Schmid and R.-W. Peng, Mol. Cancer Ther., 2020, 19, 661–672 CrossRef CAS.
-
R. E. Farrell, in RNA Methodologies, ed. R. E. Farrell, Academic Press, San Diego, 4th edn, 2010, ch. 23, pp. 539–560 Search PubMed.
- P. M. Bruno, Y. Liu, G. Y. Park, J. Murai, C. E. Koch, T. J. Eisen, J. R. Pritchard, Y. Pommier, S. J. Lippard and M. T. Hemann, Nat. Med., 2017, 23, 461–471 CrossRef CAS.
- K. Suntharalingam, W. Lin, T. C. Johnstone, P. M. Bruno, Y.-R. Zheng, M. T. Hemann and S. J. Lippard, J. Am. Chem. Soc., 2014, 136, 14413–14416 CrossRef CAS.
- W. Li, Z. Jie, Z. Li, Y. Liu, Q. Gan, Y. Mao and X. Wang, Mol. Med. Rep., 2014, 9, 2423–2428 CrossRef CAS.
- S. E. Mohr and N. Perrimon, Wiley Interdiscip. Rev. RNA, 2012, 3, 145–158 CrossRef CAS.
- S. R. Bartz, Z. Zhang, J. Burchard, M. Imakura, M. Martin, A. Palmieri, R. Needham, J. Guo, M. Gordon, N. Chung, P. Warrener, A. L. Jackson, M. Carleton, M. Oatley, L. Locco, F. Santini, T. Smith, P. Kunapuli, M. Ferrer, B. Strulovici, S. H. Friend and P. S. Linsley, Mol. Cell. Biol., 2006, 26, 9377–9386 CrossRef CAS.
- H. S. Jung, O. C. Erkin, M. J. Kwon, S. H. Kim, J. I. Jung, Y.-K. Oh, S. W. Her, W. Ju, Y.-L. Choi, S. Y. Song, J. K. Kim, Y. D. Kim, G. Y. Shim and Y. K. Shin, Int. J. Cancer, 2012, 130, 1925–1936 CrossRef CAS.
- S. Ganesh, A. K. Iyer, J. Weiler, D. V. Morrissey and M. M. Amiji, Mol. Ther.--Nucleic Acids, 2013, 2, e110 CrossRef.
- H. Haraguchi, Metallomics, 2017, 9, 1001–1013 RSC.
- W. Hu, Q. Luo, K. Wu, X. Li, F. Wang, Y. Chen, X. Ma, J. Wang, J. Liu, S. Xiong and P. J. Sadler, Chem. Commun., 2011, 47, 6006–6008 RSC.
- D. Hu, Y. Liu, Y.-T. Lai, K.-C. Tong, Y.-M. Fung, C.-N. Lok and C.-M. Che, Angew. Chem., Int. Ed., 2016, 55, 1387–1391 CrossRef CAS.
- F.-X. Wang, J.-H. Liang, H. Zhang, Z.-H. Wang, Q. Wan, C.-P. Tan, L.-N. Ji and Z.-W. Mao, ACS Appl. Mater. Interfaces, 2019, 11, 13123–13133 CrossRef CAS.
- X. Xu, H. Wang, H. Li, X. Hu, Y. Zhang, X. Guan, P. H. Toy and H. Sun, Chem. Commun., 2019, 55, 13120–13123 RSC.
- M. Koohi-Moghadam, H. Wang, Y. Wang, X. Yang, H. Li, J. Wang and H. Sun, Nat. Mach. Intell., 2019, 1, 561–567 CrossRef.
- F. Guidi, A. Modesti, I. Landini, S. Nobili, E. Mini, L. Bini, M. Puglia, A. Casini, P. J. Dyson, C. Gabbiani and L. Messori, J. Inorg. Biochem., 2013, 118, 94–99 CrossRef CAS.
- S. M. Meier, D. Kreutz, L. Winter, M. H. M. Klose, K. Cseh, T. Weiss, A. Bileck, B. Alte, J. C. Mader, S. Jana, A. Chatterjee, A. Bhattacharyya, M. Hejl, M. A. Jakupec, P. Heffeter, W. Berger, C. G. Hartinger, B. K. Keppler, G. Wiche and C. Gerner, Angew. Chem., Int. Ed., 2017, 56, 8267–8271 CrossRef CAS.
- M. V. Babak, S. M. Meier, K. V. M. Huber, J. Reynisson, A. A. Legin, M. A. Jakupec, A. Roller, A. Stukalov, M. Gridling, K. L. Bennett, J. Colinge, W. Berger, P. J. Dyson, G. Superti-Furga, B. K. Keppler and C. G. Hartinger, Chem. Sci., 2015, 6, 2449–2456 RSC.
- A. Yimit, O. Adebali, A. Sancar and Y. Jiang, Nat. Commun., 2019, 10, 309 CrossRef.
- W. Zeng, Z. Du, Q. Luo, Y. Zhao, Y. Wang, K. Wu, F. Jia, Y. Zhang and F. Wang, Anal. Chem., 2019, 91, 6035–6042 CrossRef CAS.
- P. Zhang, J. Chen, Y. Wang, Y. Huang, Y. Tian, Z. Zhang and F. Xu, Chem. Res. Toxicol., 2016, 29, 776–783 Search PubMed.
- Y. Wang, Q.-Y. He, C.-M. Che and J.-F. Chiu, Proteomics, 2006, 6, 131–142 CrossRef CAS.
- R. McRae, P. Bagchi, S. Sumalekshmy and C. J. Fahrni, Chem. Rev., 2009, 109, 4780–4827 CrossRef CAS.
- S. J. M. Van Malderen, T. Van Acker, B. Laforce, M. De Bruyne, R. de Rycke, T. Asaoka, L. Vincze and F. Vanhaecke, Anal. Bioanal. Chem., 2019, 411, 4849–4859 CrossRef CAS.
- L. Hao, Z. W. Li, D. Y. Zhang, L. He, W. Liu, J. Yang, C. P. Tan, L. N. Ji and Z. W. Mao, Chem. Sci., 2019, 10, 1285–1293 RSC.
- C. Policar, J. B. Waern, M.-A. Plamont, S. Clède, C. Mayet, R. Prazeres, J.-M. Ortega, A. Vessières and A. Dazzi, Angew. Chem., Int. Ed., 2011, 50, 860–864 CrossRef CAS.
- J. A. Muns, V. Montserrat, H. J. Houthoff, K. Codee-van der Schilden, O. Zwaagstra, N. J. Sijbrandi, E. Merkul and G. van Dongen, J. Nucl. Med., 2018, 59, 1146–1151 CrossRef CAS.
- J. J. Conesa, A. C. Carrasco, V. Rodríguez-Fanjul, Y. Yang, J. L. Carrascosa, P. Cloetens, E. Pereiro and A. M. Pizarro, Angew. Chem., Int. Ed., 2020, 59, 1270–1278 CrossRef CAS.
- S. C. Wilschefski and M. R. Baxter, Clin. Biochem. Rev., 2019, 40, 115–133 Search PubMed.
- S. Ashoka, B. M. Peake, G. Bremner, K. J. Hageman and M. R. Reid, Anal. Chim. Acta, 2009, 653, 191–199 CrossRef CAS.
- C. Venzago, M. Popp, J. Kovac and A. Kunkel, J. Anal. At. Spectrom., 2013, 28, 1125–1129 RSC.
-
B. Alberts, A. Johnson and J. Lewis, et al., Molecular Biology of the Cell, Garland Science, New York, 4th edn, 2002 Search PubMed.
- M. Corte Rodríguez, R. Álvarez-Fernández García, E. Blanco, J. Bettmer and M. Montes-Bayón, Anal. Chem., 2017, 89, 11491–11497 CrossRef.
- M. H. M. Klose, S. Theiner, C. Kornauth, S. M. Meier-Menches, P. Heffeter, W. Berger, G. Koellensperger and B. K. Keppler, Metallomics, 2018, 10, 388–396 RSC.
- R. M. A. Heeren, Int. J. Mass Spectrom., 2015, 377, 672–680 CrossRef CAS.
- P. Massonnet and R. M. A. Heeren, J. Anal. At. Spectrom., 2019, 34, 2217–2228 RSC.
- A. A. Legin, A. Schintlmeister, M. A. Jakupec, M. Galanski, I. Lichtscheidl, M. Wagner and B. K. Keppler, Chem. Sci., 2014, 5, 3135–3143 RSC.
-
K. K. W. Lo, Luminescent and photoactive transition metal complexes as biomolecular probes and cellular reagents, Springer, Berlin, Heidelberg, 2015 Search PubMed.
- D.-L. Ma, H.-Z. He, K.-H. Leung, D. S.-H. Chan and C.-H. Leung, Angew. Chem., Int. Ed., 2013, 52, 7666–7682 CrossRef CAS.
- Y. Arenas, S. Monro, G. Shi, A. Mandel, S. McFarland and L. Lilge, Photodiagnosis Photodyn. Ther., 2013, 10, 615–625 CrossRef CAS.
- E. V. Puttock, M. T. Walden and J. A. G. Williams, Coord. Chem. Rev., 2018, 367, 127–162 CrossRef CAS.
- H. Li, C. Xie, R. Lan, S. Zha, C.-F. Chan, W.-Y. Wong, K.-L. Ho, B. D. Chan, Y. Luo, J.-X. Zhang, G.-L. Law, W. C. S. Tai, J.-C. G. Bünzli and K.-L. Wong, J. Med. Chem., 2017, 60, 8923–8932 CrossRef CAS.
- Y. Chen, R. Guan, C. Zhang, J. Huang, L. Ji and H. Chao, Coord. Chem. Rev., 2016, 310, 16–40 CrossRef CAS.
- K. K.-W. Lo and S. P.-Y. Li, RSC Adv., 2014, 4, 10560–10585 RSC.
- J. Liu, Y. Chen, G. Li, P. Zhang, C. Jin, L. Zeng, L. Ji and H. Chao, Biomaterials, 2015, 56, 140–153 CrossRef CAS.
- M. J. Rose, N. L. Fry, R. Marlow, L. Hinck and P. K. Mascharak, J. Am. Chem. Soc., 2008, 130, 8834–8846 CrossRef CAS.
- Y. Yuan, Y. Chen, B. Z. Tang and B. Liu, Chem. Commun., 2014, 50, 3868–3870 RSC.
- P. C. Ford, Coord. Chem. Rev., 2018, 376, 548–564 CrossRef CAS.
- S. Garcia-Gallego and G. J. L. Bernardes, Angew. Chem., Int. Ed., 2014, 53, 9712–9721 CrossRef CAS.
- K. Meister, J. Niesel, U. Schatzschneider, N. Metzler-Nolte, D. A. Schmidt and M. Havenith, Angew. Chem., Int. Ed., 2010, 49, 3310–3312 CrossRef CAS.
- N. P. Lenzo, D. Meyrick and J. H. Turner, Diagnostics, 2018, 8, 16 CrossRef.
- R. C. Lange, R. P. Spencer and H. C. Harder, J. Nucl. Med., 1973, 14, 191–195 CAS.
- N. Tinker, B. De Spiegeleer, H. Sharma, H. Jackson, C. McAuliffe and J. P. Reman, Nucl. Med. Biol., 1990, 17, 427–436 CAS.
- E. A. Aalbersberg, B. J. de Wit-van der Veen, O. Zwaagstra, K. Codee-van der Schilden, E. Vegt and W. V. Vogel, Eur. J. Nucl. Med. Mol. Imaging, 2017, 44, 1347–1354 CrossRef CAS.
- J. S. Lewis, D. W. McCarthy, T. J. McCarthy, Y. Fujibayashi and M. J. Welch, J. Nucl. Med., 1999, 40, 177–183 CAS.
- J. B. Hilton, S. W. Mercer, N. K. H. Lim, N. G. Faux, G. Buncic, J. S. Beckman, B. R. Roberts, P. S. Donnelly, A. R. White and P. J. Crouch, Sci. Rep., 2017, 7, 42292 CrossRef CAS.
- B. De Samber, M. J. Niemiec, B. Laforce, J. Garrevoet, E. Vergucht, R. De Rycke, P. Cloetens, C. F. Urban and L. Vincze, PLoS One, 2016, 11, e0165604 CrossRef.
- A. Hummer and A. Rompel, Metallomics, 2013, 5, 597–614 RSC.
- M. J. Pushie, I. J. Pickering, M. Korbas, M. J. Hackett and G. N. George, Chem. Rev., 2014, 114, 8499–8541 CrossRef CAS.
- J. Z. Zhang, N. S. Bryce, A. Lanzirotti, C. K. J. Chen, D. Paterson, M. D. de Jonge, D. L. Howard and T. W. Hambley, Metallomics, 2012, 4, 1209–1217 RSC.
- C. Sanchez-Cano, I. Romero-Canelón, Y. Yang, I. J. Hands-Portman, S. Bohic, P. Cloetens and P. J. Sadler, Chem.–Eur. J., 2017, 23, 2512–2516 CrossRef CAS.
- M. D. Hall, R. A. Alderden, M. Zhang, P. J. Beale, Z. Cai, B. Lai, A. P. Stampfl and T. W. Hambley, J. Struct. Biol., 2006, 155, 38–44 CrossRef CAS.
- C. C. Konkankit, J. Lovett, H. H. Harris and J. J. Wilson, Chem. Commun., 2020, 56, 6515–6518 RSC.
- C. K. J. Chen, P. Kappen, D. Gibson and T. W. Hambley, Dalton Trans., 2020, 49, 7722–7736 RSC.
- A. Levina, J. Aitken, Y. Gwee, Z. J. Lim, M. Liu, A. Singharay, P. Wong and P. Lay, Chem.–Eur. J., 2013, 19, 3609–3619 CrossRef CAS.
- X. Zhang, F. Ponte, E. Borfecchia, A. Martini, C. Sanchez-Cano, E. Sicilia and P. J. Sadler, Chem. Commun., 2019, 55, 14602–14605 RSC.
- J. F. Collingwood and F. Adams, Spectrochim. Acta, B, 2017, 130, 101–118 CrossRef CAS.
- F. Lermyte, J. Everett, J. Brooks, F. Bellingeri, K. Billimoria, P. J. Sadler, P. B. O'Connor, N. D. Telling and J. F. Collingwood, Cells, 2019, 8, 1231 CrossRef CAS.
- A. E. Weston, H. E. J. Armer and L. M. Collinson, J. Chem. Biol., 2010, 3, 101–112 CrossRef.
- J. Groen, J. J. Conesa, R. Valcárcel and E. Pereiro, Biophys. Rev., 2019, 11, 611–619 CrossRef.
- S. Gil, E. Solano, F. Martínez-Trucharte, J. Martínez-Esaín, A. J. Pérez-Berná, J. J. Conesa, C. Kamma-Lorger, M. Alsina and M. Sabés, PLoS One, 2020, 15, e0230022 CrossRef CAS.
- E. M. Bolitho, C. Sanchez-Cano, H. Huang, I. Hands-Portman, M. Spink, P. D. Quinn, M. Harkiolaki and P. J. Sadler, J. Biol. Inorg Chem., 2020, 25, 295–303 CrossRef CAS.
- J. Doherty, A. Raoof, A. Hussain, M. Wolna, G. Cinque, M. Brown, P. Gardner and J. Denbigh, Analyst, 2019, 144, 997–1007 RSC.
- L. Quaroni, T. Zlateva, B. Sarafimov, H. W. Kreuzer, K. Wehbe, E. L. Hegg and G. Cinque, Biophys. Chem., 2014, 189, 40–48 CrossRef CAS.
- R. R. Vernooij, T. Joshi, E. Shaili, M. Kubeil, D. R. T. Appadoo, E. I. Izgorodina, B. Graham, P. J. Sadler, B. R. Wood and L. Spiccia, Inorg. Chem., 2016, 55, 5983–5992 CrossRef CAS.
- R. E. F. de Paiva, A. Marçal Neto, I. A. Santos, A. C. G. Jardim, P. P. Corbi and F. R. G. Bergamini, Dalton Trans., 2020 10.1039/d0dt02478c.
-
RECOVERY collaborative group, N. Engl. J. Med., 2020, DOI:10.1056/nejmoa2021436.
- S. Yuan, R. Wang, J. F.-W. Chan, A. J. Zhang, T. Cheng, K. K.-H. Chik, Z.-W. Ye, S. Wang, A. C.-Y. Lee, L. Jin, H. Li, D.-Y. Jin, K.-Y. Yuen and H. Sun, Nat. Microbiol., 2020 DOI:10.1038/s41564-020-00802-x.
- J. S. Zhang, E. W. Taylor, K. Bennett, R. Saad and M. P. Rayman, Am. J. Clin. Nutr., 2020, 111, 1297–1299 CrossRef.
-
MRC protein phosphorylation and ubiquitylation unit, List of clinically approved kinase inhibitors, https://www.ppu.mrc.ac.uk/list-clinically-approved-kinase-inhibitors, accessed 10/07/2020 Search PubMed.
Footnote |
† Electronic supplementary information (ESI) available: The biographies of the authors, extended reading (S1–S3 in Section 3), extended data in Tables S1–S14 and Fig. S1 (Sections 2 and 3), additional references in Section 5, and structures of metallodrugs mentioned in the text in Section 6. See DOI: 10.1039/d0sc04082g |
|
This journal is © The Royal Society of Chemistry 2020 |