DOI:
10.1039/D3MA00952A
(Perspective)
Mater. Adv., 2024,
5, 1454-1461
Carbon-based two-dimensional (2D) materials: a next generation biocidal agent
Received
2nd November 2023
, Accepted 23rd January 2024
First published on 29th January 2024
Abstract
Two-dimensional materials (2D-Ms) such as graphene, carbon nitride (C3N4), and MXene have attracted significant attention due to their excellent physico-chemical properties, including high surface area-to-volume ratio, biocompatibility, mechanical strength, high conductivity, etc. There has been growing interest in utilizing 2D-Ms for antibacterial applications including photo-antibacterial activity. The rise of antibiotic-resistant bacteria has made new antibiotic materials imperative, and 2D-Ms have shown promise in this area. One of the main advantages of 2D-Ms for antibacterial applications is their high surface area-to-volume ratio, which increases contact between the material and bacteria, leading to more effective antibacterial properties. Additionally, some carbon-based 2D-Ms (CB-2D-Ms) have been shown to have intrinsic antibacterial properties, such as graphene and its derivatives, g-C3N4, MXene, etc., as backbone carbon provides mechanical support, which can be further enhanced by functionalization with biocidal agents (metals/metal oxides, surface functional groups, and polymers). This mini-review highlights the latest developments in CB-2D-Ms, such as graphene and its derivatives, C3N4, MXenes, etc., as antibiotic materials to control bacterial infection. Herein, we correlate the exclusive range of 2D properties of CB-2D-Ms with their antimicrobial actions. Lastly, challenges and future perspectives in this area of CB-2D-Ms are also described.
1. Introduction
Bacterial infectious disease is one of the major threats to human society, as various mutations cause the development of hazardous bacteria and viruses, which is of significant concern. Infections grown from these bacteria and viruses are bringing serious threats to the human population globally, causing an increased mortality rate. Numerous antibiotics are available on the market to treat infection from available bacteria and viruses. As these antibiotics are widely used, antibacterial resistance has been developed for these available antibiotics, reducing the efficacy of these antibiotics.1–5 In this context, the discovery and development of novel antibiotic materials are required to fulfil the growing population's demand.
Numerous antibiotics have been developed so far that effectively kill/inhibit bacterial strains. The excessive use of these antibiotics might lead to the development of resistance against these antibiotics that significantly enhance mortality and morbidity.6–10 Therefore, there is a need to develop newer antibiotic materials or modify existing antibiotics so as to inhibit the development of resistance against these drugs. In this aspect, nanomaterials (NMs), mainly metal oxides, metal hydroxides, and metal-doped carbon materials, might be able to control bacterial infection without the development of bacterial resistance.11–13
Two-dimensional materials (2D-Ms) have attracted the scientific community's attention because of their large pore size distribution, high surface area, excellent mechanical properties, and high chemical resistance. 2D-Ms are in demand because of their novel characteristics like easy tunability by incorporation of surface functional groups, metals/metal oxides and polymers, which significantly improve their applicability towards end applications including biocidal agents with minimal toxicity or high biocompatibility. Some widely used 2D-Ms are graphene, graphene oxide (GO), a reduced form of graphene oxide (r-GO), C3N4 nanosheets, boron nitride (BN) nanosheets, MXene, TMDs etc. Among them, CB-2D-Ms, like graphene, GO, rGO, MXene, and C3N4, are of especial interest due to their large mechanical strength. CB-2D-Ms can provide mechanical flexibility together with large surface area and pore size distribution.14–25 Additionally, several studies have shown that CB-2D-Ms can effectively inhibit the growth of various bacteria, including Escherichia coli (E. coli), Staphylococcus aureus (S. aureus), and Pseudomonas aeruginosa (P. aeruginosa). Usually, their antibacterial activity can be attributed to various mechanisms, such as disruption of bacterial cell membranes, oxidative stress, and inhibition of bacterial adhesion. In addition to their antibacterial properties, CB-2D-Ms have other advantages, such as biocompatibility, mechanical flexibility, and ease of functionalization.26–29 These properties make CB-2D-Ms promising candidates for killing/inhibiting various bacterial strains, with prospects for effective use in different applications such as wound healing, medical implants, and water purification. Simultaneously, they are biocompatible and have shown a wide range of antibiotic properties, making them excellent candidates for use as antibacterial agents. CB-2D-Ms are in demand as antibacterial agents as they can provide good support as a substrate due to their tunable surface, and can be easily functionalized and changed for various applications. However, research is still required to change the surface properties for antibacterial applications.30–37 In general, there are plenty of advantages that suggest to use of CB-2D-Ms as biocidal agents, mainly high mechanical stability, high biocompatibility, easy functionalization/tunability, and mechanical flexibility. Fig. 1 shows a schematic illustration of CB-2D-Ms and their biocidal activity. This mini-review covers the recent developments in the field of CB-2D-Ms for antibacterial application. This article also highlights the newer strategies to modify the surface properties of these CB-2D-Ms We also highlight and discuss the various methods adopted to improve the efficiency of these materials.
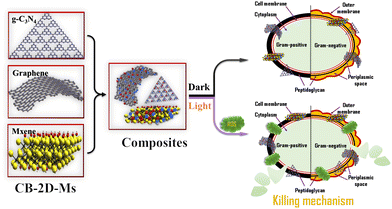 |
| Fig. 1 A schematic illustration of CB-2D-Ms and their biocidal activity. | |
2. Biocidal activity of CB-2D-Ms
CB-2D-Ms are used in several applications, including wastewater treatment, agriculture, energy storage, biomedicine etc. Moreover, applications of CB-2D-Ms as antibacterial agents are one of the growing fields that need to be explored, as most bacterial strains develop resistance against antibiotic drugs.25,38–40 CB-2D-Ms, like graphene, rGO, MXene, and C3N4, can efficiently be used as antibiotic materials without development of bacterial resistance with minimal toxicity.
2.1. Graphene and its derivatives
Graphene is one of the most in-demand materials in wastewater treatment and energy storage applications. Furthermore, graphene derivatives such as GO and rGO are gaining widespread attention as antibacterial agents. However, antibacterial properties demand surface functionality by chemicals, metals/metal oxides, and polymers.41–44 Several researches show different functionalization strategies to increase the antibacterial properties of graphene and its derivatives. For instance, Khanam et al. synthesized GO using a modified Hummers’ method and then synthesized r-GO using Allium cepa extract as reducing agent. This biosynthesized r-GO was applied as an antimicrobial agent against Gram-negative (E. coli and P. aeruginosa) and Gram-positive (S. faecalis and S. aureus) bacterial strains. The data suggested that the r-GO significantly reduces the cell viability and its efficacy depends on the incubation time.45 Another study by Bykkam synthesized ZnO nanoparticles (NPs), loaded a few layers of graphene and applied for antibacterial use. Bykkam found that the synthesized ZnO-loaded few-layer graphene nanocomposite proved to be an excellent antibacterial agent against E. coli and S. typhi.46 Ali et al. synthesized graphene incorporated chitosan/gelatin nanofibers using electrospinning process for antibacterial and wound healing applications. The data indicate that the prepared nanofibrous mat effectively inhibits/kills E. coli and S. aureus. Moreover, migration of cells significantly improved upon incorporation of graphene, thereby healing wounds.47 Jiang et al. synthesized Ag-loaded graphene, which exhibits excellent antibacterial properties. The authors decorated 40–50 nm Ag-NPs on graphene layers to achieve maximum performance towards bacterial degradation.48 Another study by Krishnamoorthy et al. synthesized graphene nanolayers using the liquid-phase exfoliation method. The exfoliated graphene proved to be an excellent candidate against E. coli and S. typhimurium. The study also mentioned the mechanistic approach of the antibacterial nature of graphene synthesized using liquid-phase exfoliation as free radicals are involved in the antimicrobial activity, especially reactive oxygen species (ROS), which react with the cell wall and leading to bacterial cell death.49
Derivatives of graphene, especially GO, also proved to be excellent antibacterial agents due to their properties like electrical conductivity, high surface area and pore size distribution, excellent physico-chemical properties and extraordinary mechanical strength with great functionality. Several literature studies claim that GO's antibacterial activity lies in its ability to generate ROS, which directly attack bacterial cell walls and leads to cell death.50 GO demonstrates extraordinary properties, including mild cytotoxicity, and a simple synthesis process for large-scale production with economic viability. Another advantage is that bacterial killing involves two mechanisms, physical destruction and chemical oxidation, which reduces bacterial resistance.51 Several literature studies have proved the efficiency of GO and its composites as antibacterial agents, including that of Shamsi et al. who synthesized gallic acid-loaded GO and used the synthesized nanocomposites as an antibacterial agent against S. aureus. The authors evaluated the antibacterial activity using the disc-diffusion method (MRSA and methicillin-sensitive SA (MSSA)) at a fixed dose of gallic acid-modified GO. The authors observed that the antibacterial activity of gallic acid-modified GO against MRSA increased significantly at lower concentrations and proved that the synthesized nanocomposite of gallic acid-modified GO is effective against multi-drug-resistant bacteria.52 Li et al. synthesized CuO-NPs-loaded GO nanosheets and applied them as an antibacterial agent against P. syringae pv. tomato. The authors observed that synthesized CuO-NPs-loaded GO-based nanocomposite has high efficiency in acting as a biocide and has great potential for managing crop diseases.53 Ping Li et al. functionalized GO with guanidine polymer and applied it as an antibacterial agent against both Gram-negative bacteria (E. coli) and Gram-positive bacteria (S. aureus) and found the efficiency of functionalized GO as a potential antibacterial agent.54 Another study by Jaworski et al. decorated GO with Ag-NPs using an ultrasonic method and tested it against Gram-positive bacteria (S. aureus and S. epidermidis), Gram-negative bacteria (E. coli), and pathogenic fungi (Candida albicans (C. albicans)). The authors reported the efficiency of functionalized GO nanocomposite as an antibacterial agent.55 Tang et al. again synthesized the same nanocomposite and studied the mechanism of the synthesized nanocomposite for bacterial degradation. The authors mentioned that the synthesized composite acts as an antibacterial agent and generates ROS to degrade bacterial cell walls.56 Another graphene derivative is r-GO, which can be synthesized using a simple reduction process of GO, as GO consists of several functional groups, which somehow impact the dispersion ability of GO. To overcome this issue, GO undergoes a reduction process, which subsequently improves the dispersion as well as antibacterial performance.
Several studies highlighted the synthesis of r-GO and its composites and applied them as antibacterial agents. For example, a nanocomposite of r-GO and Ag-NPs was synthesized and applied for dual application (1) as an antibacterial and (ii) as a cancer biomarker sensor.57Fig. 2 shows the SEM images of (a) E. coli, (b) exposed E. coli, (c) S. aureus, and (d) exposed S. aureus. The SEM images clearly indicate the killing/inhibition of bacterial strains.
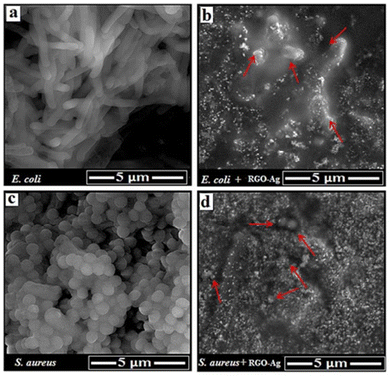 |
| Fig. 2 SEM images of (a) E. coli, (b) exposed e. coli, (c) S. aureus, and (d) exposed S. aureus. Reproduced from ref. 57 with permission from RSC, copyright 2016 under a Creative Commons Attribution 4.0 International License (CC BY 4.0). | |
Another study by Moghayedi et al. synthesized silver NPs doped with phosphomolybdate-modified rGO nanocomposite and studied the kinetics and mechanism of bacterial degradation.58 Alsharaeh et al. synthesized silver NPs-doped rGO nanocomposites and observed the antibacterial behaviour of the synthesized nanocomposites.59 The studies mentioned above clearly indicate the applicability of graphene and its derivatives as excellent antibacterial agents for various Gram-positive and Gram-negative bacteria which can demonstrate 100% bacterial degradation/killing/inhibiting ability within a very short time and low dose showing their excellence towards bacterial degradation by generating ROS.
2.2. Carbon nitrides and borides
Another class of 2D-M is C3N4, as this is a metal-free class of CB-2D-Ms The synthesis of C3N4 is very simple and easy as it requires only carbon and nitrogen derivatives, mainly compounds of urea and melamine. After polycondensation, a layered structure is formed, assembled through van der Waals interaction. Due to various properties like high chemical inertness and structural stability, these materials are widely used in different applications, including wastewater treatment, solar cells, energy storage, fuel cells, etc.60–62 Moreover, due to low band gap value, these materials are widely considered as semiconductors and are mainly used in photocatalysis. The antibacterial activity of graphitic C3N4 (g-C3N4) has also been studied by several authors and it has been proved that this metal-free carbon nitrogen skeleton could significantly disrupt bacterial cell walls under solar irradiation. Several studies have reported the photo-antibacterial application of C3N4-based semiconductor material. For example, Cui et al. synthesized g-C3N4 nanosheets. Further, the synthesized g-C3N4 nanosheets were subjected to plasma treatment to reduce surface defects and expose nitrogen vacancies. The synthesized g-C3N4 was applied as a photo-antibacterial agent, demonstrating excellent performance against Gram-positive and Gram-negative bacteria.63 Fang et al. synthesized phosphorus and sulfur co-doped C3N4 nanosheets and applied them for photo-antibacterial use. The synthesized material showed excellent activity towards bacterial cell wall degradation.64 Sun et al. synthesized GO-g-C3N4 and tested it against an E. coli bacterial strain. The data indicate that GO-g-C3N4 killed 97.9% E. coli within 120 min under solar irradiation.65 Shoran et al. synthesized CeO2-g-C3N4-based composite materials for photodegradation of Gram-negative (S. abony and E. coli) and Gram-positive (S. aureus and B. cereus) bacteria. The data indicate that the prepared CeO2-g-C3N4-based composite materials effectively photodegrade both types of bacterial strains.66 Wang et al. synthesized Cu2O, g-C3N4, and Cu2O-g-C3N4-based composite materials for photodegradation of bacteria. The data suggested that the prepared Cu2O-g-C3N4-based composite materials have antibacterial activity, which was significantly improved under solar light irradiation.67 Yin et al. synthesized BiFeO3-g-C3N4 mushroom heterojunction for photo-antibacterial and wound healing applications. The data indicate that the prepared composite materials inhibit/kill E. coli and S. aureus and promote healing of wounds.68 Another study synthesized g-C3N4-functionalized self-cleaning membranes for antibacterial applications. The data suggested that the g-C3N4-functionalized self-cleaning membranes effectively degrade bacterial strains and dyes, which provide a newer avenue for water treatment applications.69 This study provides newer opportunities to develop self-cleaning membranes for the removal of contaminants from water. However, further research is required that should be focused on the stability of self-cleaning membranes. Fig. 3 shows a schematic illustration of g-C3N4-based functionalized self-cleaning membranes for the photodegradation of dyes and bacteria.
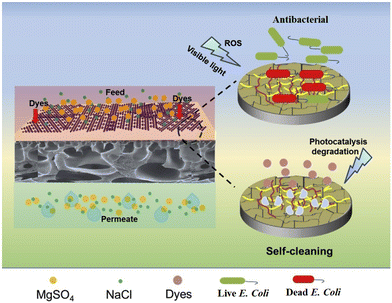 |
| Fig. 3 A schematic illustration of g-C3N4-based self-cleaning membranes for the photocatalytic degradation of dyes and bacteria. Reproduced from ref. 69 with permission from Elsevier, copyright 2019. | |
Gao et al. synthesized Fe3+-doped alkalized C3N4 and tested it against Pseudomonas aeruginosa (P. aeruginosa), E. coli, and S. aureus bacterial strains and found it effective against these bacteria.70Fig. 4 shows a schematic representation of the photo-antibacterial activity of C3N4. These studies support the antibacterial application of C3N4. Under light absorption, due to a large band gap, absorption of light is limited, which limits the overall performance. Moreover, the addition of dopant and organic functionalization can decrease the band gap value and, subsequently, bacterial cell wall degradation. Furthermore, g-C3N4-based nanocomposites have been synthesized, which helps expand visible light absorption ranges and enhance their antibacterial performance. However, some concerns remain, such as the generation of toxins during cell wall degradation, which interfere with protein synthesis and oxidize organics. Another concern is that the response of C3N4 towards bacterial degradation mainly depends on absorption of particular wavelengths. In this context, band gap modulation is required to enhance the absorption in a broad range.
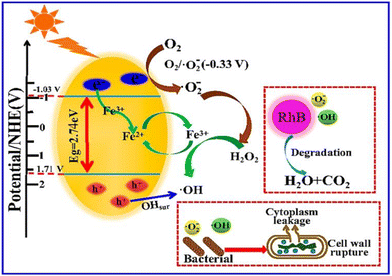 |
| Fig. 4 Schematic representation of photo-antibacterial activity. Reproduced from ref. 70 with permission from MDPI, copyright 2020 under a Creative Commons Attribution 4.0 International License (CC BY 4.0). | |
The g-C3N4 also shows antibacterial activity without exposure to light by incorporating metal/metal oxide NPs. For instance, Qamar et al. synthesized g-C3N4–Cr–ZnO-based composite material with antibacterial activity against Gram-negative (E. coli) and Gram-positive (Bacillus subtilis, S. aureus, and S. salivarius) bacteria. The data suggested that the prepared composite had excellent antibacterial activity due to synergetic effects of the heterojunction between g-C3N4 and Cr–ZnO.71 Another report shows the synthesis of GO-g-C3N4 for photodegradation of amyloid β protein. This study might open newer possibilities to kill bacteria by degradation of bacterial protein.72 These studies suggested that g-C3N4 materials have antibacterial activity against various bacterial strains under solar light irradiation as well as without use of light.
2.3. MXene (metal carbide)
MXene, another CB-2D-M, has been widely used as an antibacterial agent. MXene is synthesized using the MAX phase (compound of metal aluminium carbide). The general formula of the MAX phase is Mn+1XnTx, where M is a transition metal element. This newly discovered material finds significant interest in energy storage due to its large conductivity. However, novel applications include wastewater treatment and solar cells.73–76 In addition, MXene and its derivatives are now gradually entering the biomedical science and drug delivery fields due to their excellent biocompatibility, great physicochemical-biological properties, contact-killing capacity, phototherapy activity and non-toxic behaviour. As reported, MXene can induce oxidative stress, resulting in cell damage, and can be applied as an antibacterial agent against various pathogens.77 Several literature studies report the application of MXene as an antibacterial agent. For example, Gao et al. synthesized Ti3C2Tx MXene nanosheets and tested them against methicillin-resistant Staphylococcus aureus.77 Another study by Tahir et al. synthesized Gd3+-doped vanadium oxide-based 2D-MXene nanosheet composite using the sol–gel method and applied it against Gram-positive S. aureus and Gram-negative P. vulgaris strains of bacteria.78 Shamsabadi et al. synthesized colloidal Ti3C2Tx MXene nanosheets having lateral sizes of 0.09, 0.35, 0.57, and 4.40 μm and tested them against E. coli and B. subtilis bacteria.17 Rasool et al. synthesized an MXene nanosheet-based antibacterial membrane on polyvinylidene fluoride (PVDF) support, tested it against E. coli and B. subtilis, and found 99% growth inhibition of both bacteria over the membrane surface.79 Warsi et al. synthesized an MXene/WO3 nanosheet composite using a simple sonication method. The authors observed that the synthesized nanocomposite showed good antibacterial properties against Gram-positive bacterial strains; however, cell damage of Gram-negative strains is concentration-dependent.80Fig. 5. shows SEM images of (a) WO3, (b) WO3-MXene, and (c) MXene. The interlayer spacing between two adjacent layers confirms the formation of MXene. Talreja et al. synthesized Cu-MXene to control bacterial infection effectively.81 These studies proved the great potential of MXene and MXene-based nanocomposites as antibacterial agents. The MXene nanosheets were found to damage bacterial cells significantly quickly, releasing bacterial DNA followed by bacterial cell dispersion, resulting in bacterial cell death. In conclusion, MXene and its composites are promising antibacterial agents against various pathogens.
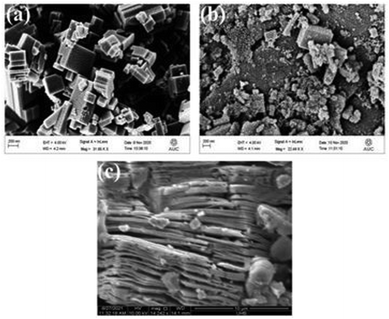 |
| Fig. 5 SEM images of (a) WO3, (b) WO3-MXene, and (c) MXene. Reproduced from ref. 80 with permission from MDPI, copyright 2022 under a Creative Commons Attribution 4.0 International License (CC BY 4.0). | |
Table 1 summarizes the different CB-2D-Ms and their antibacterial activities. The data suggested that the CB-2D-Ms effectively inhibited/killed bacterial strains. The antibacterial activity is mainly due to the metals incorporated within the CB-2D-Ms.
Table 1 Different CB-2D-Ms and their biocidal activities
No. |
CB-2D-M |
Method of synthesis |
Bacterial strain |
Ref. |
1. |
Graphene |
Hydrothermal |
E. coli, S. typhimurium, Enterococcus faecalis, and B. subtilis |
49
|
2. |
Ag/graphene composite |
Chemical reduction |
E. coli
|
48
|
3. |
ZnO decorated graphene |
Hydrothermal |
E. coli and S. typhi |
46
|
4. |
Graphene |
Green synthesis |
E. coli and P. aeruginosa |
45
|
5. |
Gallic acid–GO |
Modified Hummers’ method followed by stirring |
S. aureus
|
52
|
6. |
GO–CuO |
Modified Hummers’ method followed by reduction and immobilization |
P. syringae pv. tomato
|
53
|
7. |
GO–guanidine |
Modified Hummers’ method followed by covalent conjugation |
E. coli and S. aureus |
54
|
8. |
GO–Ag |
Modified Hummers’ method followed by ultrasonication |
E. coli, S. aureus, S. epidermidis, and C. albicans |
55
|
9. |
GO–Ag |
Modified Hummers’ method followed by a reduction |
E. coli and S. aureus |
56
|
10. |
Agphosphomolybdate/rGO |
Modified Hummers’ method followed by hydrothermal method |
E. coli
|
84
|
11. |
Ag-rGO |
Microwave irradiation (MWI) and UV light irradiation |
E. coli
|
59
|
12. |
Phosphorus/sulfur–C3N4 |
Two-step template-free method |
E. coli and S. aureus |
64
|
13. |
Fe3+-doped alkalized C3N4 |
Calcination |
P. aeruginosa, E. coli, and S. aureus |
70
|
14. |
GO-g-C3N4 |
Reduction and heating |
E. Coli
|
65
|
15. |
CeO2-g-C3N4 |
Hydrothermal |
S. abony, E. Coli, S. aureus and B. Cereus |
66
|
16. |
g-C3N4–Cr–ZnO |
Co-precipitation |
E. Coli, Bacillus subtilis, S. aureus, and S. salivarius |
71
|
17. |
g-C3N4-membrane |
Acid etching |
E. coli
|
69
|
18. |
MXene |
Chemical etching |
MRSA |
77
|
19. |
Colloidal MXene |
Chemical etching followed by sonication |
E. coli and Bacillus subtilis |
17
|
3. Mechanism of action
The mechanism of killing or inhibiting bacteria is quite different for CB-2D-Ms due to their unique physical and chemical properties. CB-2D-Ms can effectively inhibit bacterial growth by various aspects. Usually, the main active mechanism involved is the ability of 2D-Ms to damage the bacterial cell membrane physically. The ultra-fine edges of thin nanolayers of CB-2D-Ms allow them to easily penetrate the bacterial cell membrane, causing structural damage and leakage of intracellular components. This disruption of the bacterial membrane ultimately leads to cell death. Another advantage of CB-2D-Ms is their high surface area-to-volume ratio, which allows them to interact with bacterial cells effectively. This property is particularly useful in the case of biofilms, which are notoriously difficult to eradicate using conventional antibiotics. By physically disrupting the biofilm structure and inducing oxidative stress, CB-2D-Ms can effectively inhibit biofilm formation and growth. In addition to their physical action, CB-2D-Ms also exhibit antimicrobial activity through chemical interactions with bacterial cells. For instance, Nanda et al. mentioned that GO can disrupt bacterial membrane potential and induce oxidative stress, leading to bacterial death.82 Similarly, Wang et al. observed that under solar irradiation, C3N4 inhibited bacterial growth by generating ROS, which can damage bacterial DNA and proteins.83Fig. 6 shows a schematic representation of the mechanism of action of CB-2D-Ms Furthermore, using CB-2D-Ms as antibacterial agents offers several advantages over traditional antibiotics. These materials demonstrate a wide spectrum of action against several bacteria, as well as drug-resistant bacteria. Additionally, CB-2D-Ms exhibit low cytotoxicity towards mammalian cells, making them excellent candidates for medical applications.
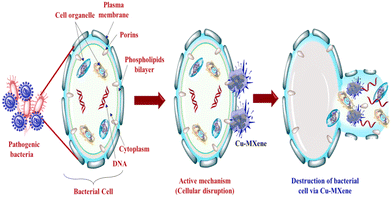 |
| Fig. 6 Schematic representation of the mechanism of action of CB-2D-Ms Reproduced from ref. 81 with permission from Elsevier, copyright 2023. | |
In general, we can say that the mode of action of CB-2D-Ms for antibacterial applications involves both physical and chemical interactions with bacterial cells. Their unique properties, such as high surface area-to-volume ratio and broad-spectrum activity, effectively inhibit bacterial growth and biofilm formation. With further research, 2D materials have the potential to become an important class of antibacterial agents for a wide range of applications.
Table 1 Different CB-2D-Ms and their antibacterial applications.
4. Ways to improve biocidal activity of CB-2D-Ms
CB-2D-Ms have remarkable physico-chemical characteristics, which makes them next-generation materials for numerous applications including biocidal agents. We observed that CB-2D-Ms themselves have less biocidal activity but due to their tunability and easy surface functionalization, they are extensively used for biomedical applications including biocidal agents.31,85–87 In this aspect, researchers have developed a newer strategy to improve biocidal activity by incorporating metals/metal oxides, polymers, and surface functional groups to produce CB-2D-Ms-based hybrid materials. Here we discuss some important aspects of improved biocidal activity. (1) Size: smaller-sized (length) CB-2D-Ms have better biocidal activity compared with larger sizes, as smaller-sized CB-2D-Ms easily penetrate bacterial cell walls. (2) Surface texture: the surface texture of CB-2D-Ms is one of their important characteristics, as rough and porous texture significantly improves the adhesion of bacteria, thereby improving biocidal activity. (3) Surface engineering: incorporating functional groups on the surface of CB-2D-Ms (modulating their surface charge) considerably enhances their affinity towards bacterial membranes, thereby increasing interaction that leads to cellular disruption and subsequently cell death. (4) Modification: incorporation of metals/metal oxides and polymers within CB-2D-Ms via in-situ and ex-situ doping. Usually, metals/metal oxides like Cu, Zn, Ag, Au, etc., and polymers like chitosan, polyethylene glycol, etc., have antibacterial ability.88–92 Although metals/metal oxides have strong antibacterial activity, higher concentrations lead to cellular toxicity. Incorporating metals/metal oxides within CB-2D-Ms significantly improved their biocidal activity with insignificant toxicity. It is important to mention that the biocompatibility of the materials is an important factor and we need to design effective biocidal agents by using these strategies with high biocompatibility.
5. Conclusion and future prospects
In conclusion, CB-2D-Ms are competitive antimicrobial agents. However, challenges are related to the stability and large-scale application of CB-2D-Ms Moreover, the stability and the performance efficacy depend on the thickness, size, and functionality of the surface of CB-2D-Ms Additionally, different synthesis approaches have been adopted to control the various factors subsequent to their performance.
Further, functional CB-2D-Ms with various encapsulated agents to increase the interlayer spacing or adhere on the surface of CB-2D-Ms might provide novel ideas to address the stability challenges and foster antimicrobial activity. Moreover, incorporating metals/metal oxides, polymers, and surface functional groups might improve the antibacterial efficiency of CB-2D-Ms Studies might provide general guidelines for tuning physico-chemical properties that are required for the concept of antibiotic materials. Additionally, in-depth mechanism of action is required to understand the molecular as well as cellular aspects between microorganisms and CB-2D-Ms In summary, this review deepens the knowledge of CB-2D-Ms and their properties to facilitate biocidal activity, which can help to reveal the hidden properties of available 2D-Ms and novel CB-2D-Ms for various biomedical applications including antibiotic materials as well as in wound healing. However, challenges still need to be addressed in the development of CB-2D-Ms for antibacterial applications. These include improving the stability and biocompatibility of the materials, optimizing their antibacterial properties, and ensuring their long-term safety. In conclusion, CB-2D-Ms have shown great potential for antibacterial applications due to their unique properties and intrinsic antibacterial activity. Further research is needed to fully realize the potential of CB-2D-Ms for antibacterial applications and address the challenges associated with their development.
Author contributions
NT & MA conceptualized design, wrote, and revised the manuscript. DC wrote the original draft.
Conflicts of interest
There are no conflicts to declare.
Acknowledgements
The authors did not receive any funds for this work.
References
- W. Sun and F.-G. Wu, Chem. – Asian J., 2018, 13, 3378–3410 CrossRef CAS PubMed.
- M. Ashfaq, N. Verma and S. Khan, Mater. Sci. Eng., C, 2017, 77, 630–641 CrossRef CAS PubMed.
- M. Ashfaq, N. Verma and S. Khan, Mater. Sci. Eng., C, 2016, 59, 938–947 CrossRef CAS PubMed.
- R. E. Baker, A. S. Mahmud, I. F. Miller, M. Rajeev, F. Rasambainarivo, B. L. Rice, S. Takahashi, A. J. Tatem, C. E. Wagner, L.-F. Wang, A. Wesolowski and C. J. E. Metcalf, Nat. Rev. Microbiol., 2022, 20, 193–205 CrossRef CAS PubMed.
- M. L. Cohen, Nature, 2000, 406, 762–767 CrossRef CAS PubMed.
- B. Aslam, W. Wang, M. I. Arshad, M. Khurshid, S. Muzammil, M. H. Rasool, M. A. Nisar, R. F. Alvi, M. A. Aslam, M. U. Qamar, M. K. F. Salamat and Z. Baloch, Infect. Drug Resist., 2018, 11, 1645–1658 CrossRef CAS PubMed.
- D. Chinemerem Nwobodo, M. C. Ugwu, C. Oliseloke Anie, M. T. S. Al-Ouqaili, J. Chinedu Ikem, U. Victor Chigozie and M. Saki, J. Clin. Lab. Anal., 2022, 36, e24655 CrossRef PubMed.
- A. León-Buitimea, C. R. Garza-Cárdenas, J. A. Garza-Cervantes, J. A. Lerma-Escalera and J. R. Morones-Ramírez, Front. Microbiol., 2020, 11, 1669 CrossRef PubMed.
- C. L. Ventola, P T, 2015, 40, 277–283 Search PubMed.
- Z. Pang, R. Raudonis, B. R. Glick, T.-J. Lin and Z. Cheng, Biotechnol. Adv., 2019, 37, 177–192 CrossRef CAS PubMed.
- R. Hussain, M. Hasan, K. J. Iqbal, A. Zafar, T. Tariq, M. S. Saif, S. G. Hassan, X. Shu, G. Caprioli and S. I. Anjum, J. Biotechnol., 2023, 365, 1–10 CrossRef CAS PubMed.
- T. Munawar, F. Mukhtar, M. S. Nadeem, K. Mahmood, M. Hasan, A. Hussain, A. Ali, M. I. Arshad and F. Iqbal, Mater. Chem. Phys., 2020, 253, 123249 CrossRef CAS.
- T. Munawar, M. S. Nadeem, F. Mukhtar, M. N. ur Rehman, M. Riaz, S. Batool, M. Hasan and F. Iqbal, Environ. Sci. Pollut. Res., 2022, 29, 90995–91016 CrossRef CAS PubMed.
- N. Baig, Composites, Part A, 2023, 165, 107362 CrossRef CAS.
- V. V. Tatarskiy, O. V. Zakharova, P. A. Baranchikov, D. S. Muratov, D. V. Kuznetsov and A. A. Gusev, Int. J. Mol. Sci., 2023, 24, 2783 CrossRef CAS PubMed.
- S. Iravani and R. S. Varma, RSC Adv., 2023, 13, 9665–9677 RSC.
- A. Arabi Shamsabadi, M. Sharifian Gh, B. Anasori and M. Soroush, ACS Sustainable Chem. Eng., 2018, 6, 16586–16596 CrossRef CAS.
- M. Ashfaq, N. Talreja, N. Singh and D. Chauhan, Electronics, 2023, 12, 570 CrossRef CAS.
- M. Ashfaq, N. Talreja, D. Chauhan and M. R. Viswanathan, New J. Chem., 2022, 46, 5581–5587 RSC.
- H. Mohammed, A. Kumar, E. Bekyarova, Y. Al-Hadeethi, X. Zhang, M. Chen, M. S. Ansari, A. Cochis and L. Rimondini, Front. Bioeng. Biotechnol., 2020, 8, 465 CrossRef PubMed.
- A. Radhi, D. Mohamad, F. S. Abdul Rahman, A. M. Abdullah and H. Hasan, J. Mater. Res. Technol., 2021, 11, 1290–1307 CrossRef CAS.
-
K. Hossain, M. Rafatullah, S. Z. Abbas, A. Ahmad, N. Ismail and A. Y. Maruthi, in Graphene-Based Nanotechnologies for Energy and Environmental Applications, ed. M. Jawaid, A. Ahmad and D. Lokhat, Elsevier, 2019 DOI:10.1016/B978-0-12-815811-1.00016-8, pp. 293–314.
- X. Huang, A. Zafar, K. Ahmad, M. Hasan, T. Tariq, S. Gong, S. G. Hassan, J. Guo, H. U. Javed and X. Shu, Appl. Surf. Sci. Adv., 2023, 17, 100446 CrossRef.
- M. Ashfaq, T. Wongpakham, N. Talreja, D. Chauhan, T. Tharasanit and W. Srituravanich, Mater. Today Commun., 2022, 33, 104786 CrossRef CAS.
- M. Ashfaq, N. Talreja, D. Chauhan, S. Afreen, A. Sultana and W. Srituravanich, J. Drug Delivery Sci. Technol., 2022, 70, 103268 CrossRef CAS.
- M. K. Chug and E. J. Brisbois, ACS Mater. Au, 2022, 2, 525–551 CrossRef CAS PubMed.
- H. Liu, F. Xing, Y. Zhou, P. Yu, J. Xu, R. Luo, Z. Xiang, P. Maria Rommens, M. Liu and U. Ritz, Mater. Des., 2023, 233, 112231 CrossRef CAS.
- V. Puspasari, A. Ridhova, A. Hermawan, M. I. Amal and M. M. Khan, Bioprocess Biosyst. Eng., 2022, 45, 1421–1445 CrossRef CAS PubMed.
- S. Hua, B. Huang, Z. Le and Q. Huang, Mater. Des., 2023, 231, 112033 CrossRef CAS.
- S. Hao, H. Han, Z. Yang, M. Chen, Y. Jiang, G. Lu, L. Dong, H. Wen, H. Li, J. Liu, L. Wu, Z. Wang and F. Wang, Nano-Micro Lett., 2022, 14, 178 CrossRef CAS PubMed.
- H. Li, R. Fan, B. Zou, J. Yan, Q. Shi and G. Guo, J. Nanobiotechnol., 2023, 21, 73 CrossRef PubMed.
- N. Talreja, D. Chauhan and M. Ashfaq, Antibiotics, 2023, 12, 398 CrossRef CAS PubMed.
- M.-H. Chan, R.-S. Liu and M. Hsiao, Nanoscale, 2019, 11, 14993–15003 RSC.
- S. Deshmukh, K. Pawar, V. Koli and P. Pachfule, ACS Appl. Bio Mater., 2023, 6, 1339–1367 CrossRef CAS PubMed.
- D. Tyagi, H. Wang, W. Huang, L. Hu, Y. Tang, Z. Guo, Z. Ouyang and H. Zhang, Nanoscale, 2020, 12, 3535–3559 RSC.
- Y. M. Hunge, A. A. Yadav, S.-W. Kang and H. Kim, J. Colloid Interface Sci., 2022, 606, 454–463 CrossRef CAS PubMed.
- L. Lu, Q. Yang, Q. Xu, Y. Sun, S. Tang, X. Tang, H. Liang and Y. Yu, Crit. Rev. Environ. Sci. Technol., 2022, 52, 3493–3524 CrossRef.
- L. Chen, X. Dai, W. Feng and Y. Chen, Acc. Mater. Res., 2022, 3, 785–798 CrossRef CAS.
- A. Maleki, M. Ghomi, N. Nikfarjam, M. Akbari, E. Sharifi, M.-A. Shahbazi, M. Kermanian, M. Seyedhamzeh, E. Nazarzadeh Zare, M. Mehrali, O. Moradi, F. Sefat, V. Mattoli, P. Makvandi and Y. Chen, Adv. Funct. Mater., 2022, 32, 2203430 CrossRef CAS.
- R. Raccichini, A. Varzi, S. Passerini and B. Scrosati, Nat. Mater., 2015, 14, 271–279 CrossRef CAS PubMed.
- P. Kumar, P. Huo, R. Zhang and B. Liu, Nanomaterials, 2019, 9, 737 CrossRef CAS PubMed.
- S. Yaragalla, K. B. Bhavitha and A. Athanassiou, Coatings, 2021, 11, 1197 CrossRef CAS.
- H. Ji, H. Sun and X. Qu, Adv. Drug Delivery Rev., 2016, 105, 176–189 CrossRef CAS PubMed.
- A. Lukowiak, A. Kedziora and W. Strek, Adv. Colloid Interface Sci., 2016, 236, 101–112 CrossRef CAS PubMed.
- P. Noorunnisa Khanam and A. Hasan, Int. J. Biol. Macromol., 2019, 126, 151–158 CrossRef CAS PubMed.
- S. Bykkam, S. Narsingam, M. Ahmadipour, T. Dayakar, K. Venkateswara Rao, C. Shilpa Chakra and S. Kalakotla, Superlattices Microstruct., 2015, 83, 776–784 CrossRef CAS.
- I. H. Ali, A. Ouf, F. Elshishiny, M. B. Taskin, J. Song, M. Dong, M. Chen, R. Siam and W. Mamdouh, ACS Omega, 2022, 7, 1838–1850 CrossRef CAS PubMed.
- B. Jiang, C. Tian, G. Song, W. Chang, G. Wang, Q. Wu and H. Fu, J. Mater. Sci., 2013, 48, 1980–1985 CrossRef CAS.
- K. Krishnamoorthy, M. Veerapandian, L.-H. Zhang, K. Yun and S. J. Kim, J. Phys. Chem. C, 2012, 116, 17280–17287 CrossRef CAS.
- J. Zhao, S. Huang, P. Ravisankar and H. Zhu, ACS Appl. Bio Mater., 2020, 3, 8188–8210 CrossRef CAS PubMed.
- M. Yousefi, M. Dadashpour, M. Hejazi, M. Hasanzadeh, B. Behnam, M. de la Guardia, N. Shadjou and A. Mokhtarzadeh, Mater. Sci. Eng., C, 2017, 74, 568–581 CrossRef CAS PubMed.
- S. Shamsi, N. Elias, S. Narti Edayu Sarchio and F. Md Yasin, Mater. Today: Proc., 2018, 5, S160–S165 CAS.
- Y. Li, D. Yang and J. Cui, RSC Adv., 2017, 7, 38853–38860 RSC.
- P. Li, S. Sun, A. Dong, Y. Hao, S. Shi, Z. Sun, G. Gao and Y. Chen, Appl. Surf. Sci., 2015, 355, 446–452 CrossRef CAS.
- S. Jaworski, M. Wierzbicki, E. Sawosz, A. Jung, G. Gielerak, J. Biernat, H. Jaremek, W. Łojkowski, B. Woźniak, J. Wojnarowicz, L. Stobiński, A. Małolepszy, M. Mazurkiewicz-Pawlicka, M. Łojkowski, N. Kurantowicz and A. Chwalibog, Nanoscale Res. Lett., 2018, 13, 116 CrossRef PubMed.
- J. Tang, Q. Chen, L. Xu, S. Zhang, L. Feng, L. Cheng, H. Xu, Z. Liu and R. Peng, ACS Appl. Mater. Interfaces, 2013, 5, 3867–3874 CrossRef CAS PubMed.
- R. Geetha Bai, K. Muthoosamy, F. N. Shipton, A. Pandikumar, P. Rameshkumar, N. M. Huang and S. Manickam, RSC Adv., 2016, 6, 36576–36587 RSC.
- M. Moghayedi, E. K. Goharshadi, K. Ghazvini, H. Ahmadzadeh, L. Ranjbaran, R. Masoudi and R. Ludwig, Colloids Surf., B, 2017, 159, 366–374 CrossRef CAS PubMed.
- E. Alsharaeh, S. Alazzam, F. Ahmed, N. Arshi, M. Al-Hindawi and G. K. Sing, Acta Metall. Sin. (Engl. Lett.), 2017, 30, 45–52 CrossRef CAS.
- Q. Wang, Y. Li, F. Huang, S. Song, G. Ai, X. Xin, B. Zhao, Y. Zheng and Z. Zhang, Molecules, 2023, 28, 432 CrossRef CAS PubMed.
- M. Ismael, J. Alloys Compd., 2020, 846, 156446 CrossRef CAS.
- M. Mahmud, A. F. M. M. Rahman, K. S. Salem, M. L. Bari and H. Qiu, ACS Appl. Bio Mater., 2022, 5, 5126–5139 CrossRef CAS PubMed.
- H. Cui, Z. Gu, X. Chen, L. Lin, Z. Wang, X. Dai, Z. Yang, L. Liu, R. Zhou and M. Dong, Nanoscale, 2019, 11, 18416–18425 RSC.
- Y. Fang, S. Pei, L. Zhuo, P. Cheng, H. Yuan and L. Zhang, Appl. Surf. Sci., 2022, 586, 152761 CrossRef CAS.
- L. Sun, T. Du, C. Hu, J. Chen, J. Lu, Z. Lu and H. Han, ACS Sustainable Chem. Eng., 2017, 5, 8693–8701 CrossRef CAS.
- S. Shoran, S. Chaudhary and A. Sharma, Environ. Sci. Pollut. Res., 2023, 30, 98682–98700 CrossRef CAS PubMed.
- B. Wang, L. Wu, A. Sun, T. Liu, L. Sun and W. Li, New J. Chem., 2023, 47, 13797–13809 RSC.
- Y. Yin, J. Wang, B. Chen, P. Zhang, G. Li, W. Sun, F. X. Hu and C. M. Li, Nanoscale, 2022, 14, 2686–2695 RSC.
- R. Li, Y. Ren, P. Zhao, J. Wang, J. Liu and Y. Zhang, J. Hazard. Mater., 2019, 365, 606–614 CrossRef CAS PubMed.
- Y. Gao, J. Duan, X. Zhai, F. Guan, X. Wang, J. Zhang and B. Hou, Nanomaterials, 2020, 10, 1751 CrossRef CAS PubMed.
- M. A. Qamar, S. Shahid, M. Javed, S. Iqbal, M. Sher and M. B. Akbar, J. Photochem. Photobiol., A, 2020, 401, 112776 CrossRef CAS.
- J. Wang, Z. Zhang, H. Zhang, C. Li, M. Chen, L. Liu and M. Dong, ACS Appl. Mater. Interfaces, 2019, 11, 96–103 CrossRef CAS PubMed.
- Y. Gogotsi and B. Anasori, ACS Nano, 2019, 13, 8491–8494 CrossRef CAS PubMed.
- X. Li, Z. Huang, C. E. Shuck, G. Liang, Y. Gogotsi and C. Zhi, Nat. Rev. Chem., 2022, 6, 389–404 CrossRef PubMed.
- I. C. Lee, Y.-C. E. Li, J. L. Thomas, M. H. Lee and H.-Y. Lin, Mater. Horiz., 2024 10.1039/D3MH01588B.
- N. Talreja, M. Ashfaq, D. Chauhan and M. R. Viswanathan, Environ. Res., 2023, 233, 116439 CrossRef CAS PubMed.
- Y. Gao, Y. Dong, S. Yang, A. Mo, X. Zeng, Q. Chen and Q. Peng, J. Colloid Interface Sci., 2022, 617, 533–541 CrossRef CAS PubMed.
- T. Tahir, K. Chaudhary, M. F. Warsi, M. S. Saif, I. A. Alsafari, I. Shakir, P. O. Agboola, S. Haider and S. Zulfiqar, Ceram. Int., 2022, 48, 1969–1980 CrossRef CAS.
- K. Rasool, K. A. Mahmoud, D. J. Johnson, M. Helal, G. R. Berdiyorov and Y. Gogotsi, Sci. Rep., 2017, 7, 1598 CrossRef PubMed.
- A.-Z. Warsi, F. Aziz, S. Zulfiqar, S. Haider, I. Shakir and P. O. Agboola, Nanomaterials, 2022, 12, 713 CrossRef CAS PubMed.
- N. Talreja, M. Ashfaq, D. Chauhan and R. V. Mangalaraja, Mater. Chem. Phys., 2023, 294, 127029 CrossRef CAS.
- S. S. Nanda, D. K. Yi and K. Kim, Sci. Rep., 2016, 6, 28443 CrossRef CAS PubMed.
- T. Wang, J. Zheng, J. Cai, Q. Liu and X. Zhang, Sci. Total Environ, 2022, 839, 155955 CrossRef CAS PubMed.
- M. Moghayedi, E. K. Goharshadi, K. Ghazvini, H. Ahmadzadeh and M. N. Jorabchi, Mater. Sci. Eng., B, 2020, 262, 114709 CrossRef CAS.
- V. K. Truong, M. Al Kobaisi, K. Vasilev, D. Cozzolino and J. Chapman, Curr. Opin. Biomed. Eng., 2022, 23, 100399 CrossRef CAS.
- A. Jayakumar, A. Surendranath and M. Pv, Int. J. Pharm., 2018, 551, 309–321 CrossRef CAS PubMed.
-
A. Singhwane, K. Chaturvedi, R. K. Mohapatra, A. K. Srivastava and S. Verma, Age of MXenes, Volume 2. Applications in Diagnostics, Therapeutics, and Environmental Remediation, American Chemical Society, 2023, vol. 1443, ch. 1, pp.1–17 Search PubMed.
- T. Manouras, E. Koufakis, E. Vasilaki, I. Peraki and M. Vamvakaki, ACS Appl. Mater. Interfaces, 2021, 13, 17183–17195 CrossRef CAS PubMed.
- P. Raizada, V. Soni, A. Kumar, P. Singh, A. A. Parwaz Khan, A. M. Asiri, V. K. Thakur and V.-H. Nguyen, J. Materiomics, 2021, 7, 388–418 CrossRef.
- P. D. Rakowska, M. Tiddia, N. Faruqui, C. Bankier, Y. Pei, A. J. Pollard, J. Zhang and I. S. Gilmore, Commun. Mater., 2021, 2, 53 CrossRef CAS.
- B. Balasubramaniam, Prateek, S. Ranjan, M. Saraf, P. Kar, S. P. Singh, V. K. Thakur, A. Singh and R. K. Gupta, ACS Pharmacol. Transl. Sci., 2021, 4, 8–54 CrossRef CAS PubMed.
- A. Spoială, C.-I. Ilie, R.-D. Truşcă, O.-C. Oprea, V.-A. Surdu, B. Ş. Vasile, A. Ficai, D. Ficai, E. Andronescu and L.-M. Diţu, Materials, 2021, 14, 4747 CrossRef PubMed.
|
This journal is © The Royal Society of Chemistry 2024 |
Click here to see how this site uses Cookies. View our privacy policy here.