DOI:
10.1039/C9SC01711A
(Edge Article)
Chem. Sci., 2019,
10, 5837-5842
Mechanochemistry allows carrying out sensitive organometallic reactions in air: glove-box-and-Schlenk-line-free synthesis of oxidative addition complexes from aryl halides and palladium(0)†
Received
8th April 2019
, Accepted 3rd May 2019
First published on 7th May 2019
Abstract
Organic reactions that employ moisture- and/or oxygen-sensitive reagents or intermediates usually involve the use of glove-box or Schlenk-line techniques as well as dry and degassed solvents. Unfortunately, these requirements may greatly reduce the utility of the targeted organic molecules. Herein, we demonstrate that solvent-free mechanochemical synthetic techniques allow using highly oxygen-sensitive palladium(0) species in air for the stoichiometric oxidative addition of aryl halides. The low diffusion efficiency of gaseous oxygen in crystalline or amorphous solid-state reaction mixtures should be the main reason for the low impact of the presence of atmospheric oxygen on the sensitive oxidative addition reactions under the applied conditions. This study thus illustrates the outstanding potential of mechanochemistry to serve as an operationally simple, glove-box-and-Schlenk-line-free synthetic route to organometallic compounds and other valuable synthetic targets, even when sensitive reagents or intermediates are involved.
Introduction
Many useful reagents and catalysts employed in the preparation of organic compounds and organometallic complexes are highly reactive and therefore incompatible with exposure to the ambient atmosphere.1 The techniques required to conduct reactions with these sensitive compounds usually involve gloveboxes and high-vacuum Schlenk lines and are thus costly and demand special training. In addition, dry and degassed organic solvents are required for these reactions. Chemical processes that do not require such precautions are therefore likely to substantially increase the practical utility of the targeted organic molecules.
Palladium complexes obtained from oxidative addition reactions have been widely used in mechanistic studies,2 as catalyst precursors for several cross-coupling reactions3 and as stoichiometric aryl-group transfer reagents for the diversification of biomolecules and pharmaceuticals.4 Dialkylbiaryl phosphine-ligated palladium(II) complexes developed by Buchwald and co-workers are particularly useful reagents in these applications due to their high stability and ease of handling.2a–e,3a,4 One of the most common synthetic routes to these oxidative addition complexes involves reactions between the palladium(0) precursor (COD)Pd(CH2TMS)2 (COD = 1,5-cyclooctadiene; TMS = trimethylsilyl) (1)5 and aryl halides 2 in the presence of appropriate Buchwald-type ligands (Scheme 1).2a–e,3a,4 According to the developed protocol reported by Buchwald et al., this procedure generally requires glove-box techniques, given that highly air-sensitive palladium(0) species are involved.3a,4c These restrictions may, however, greatly reduce the practical utility of the obtained palladium complexes. The development of scalable, operationally simple and glove-box-and-Schlenk-line-free synthetic methods should therefore be highly beneficial.
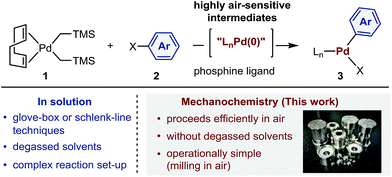 |
| Scheme 1 Synthesis of palladium-based oxidative addition complexes involving air-sensitive palladium(0) intermediates. | |
Mechanochemical solvent-free organic reactions using ball milling have emerged as powerful alternatives to synthetic procedures in solution.6 In particular, Friščić, Bolm, Hernández, James, and Browne have made significant contributions to this research area.6 However, the benefits of mechanochemistry in the context of organometallic chemistry have not yet been explored systematically,6m,7–19 and the potential applicability of highly sensitive reagents or intermediates toward mechanochemical organometallic transformations under ambient conditions remains elusive.14 Herein, we demonstrate that mechanochemistry enables glove-box-and-Schlenk-line-free synthesis of palladium complexes by oxidative additions using readily available starting materials (Scheme 1).20 This reaction is operationally simple and proceeds efficiently in air to afford a variety of synthetically useful palladium complexes in moderate to high yield. We anticipate that the method presented herein could be readily transferred to the development of glove-box-and-Schlenk-line-free synthetic routes to other valuable organometallic complexes and synthetic targets, even when sensitive reagents or intermediates are involved.
Results and discussion
All mechanochemical reactions were conducted in a Retsch MM 400 mill (stainless-steel milling jar; 30 Hz or 25 Hz; stainless-steel balls). To probe the effectiveness of this mechanochemical approach for the preparation of palladium-based oxidative addition complexes, we initially tested the reaction between 1 and 1-bromopyrene (2a) in the presence of 2-dicyclohexylphosphino-2′,6′-diisopropoxybiphenyl (RuPhos), which is a commonly used Buchwald-type ligand (Table 1).21 The corresponding oxidative addition complex (3a) was obtained in moderate yield upon grinding for 30 min in air (40% yield; entry 1). Prolonging the reaction time did not improve the yield (43% yield; entry 2). Subsequently, we attempted milling with a catalytic amount of liquid, the so-called liquid-assisted grinding (LAG), to improve the performance (entries 3–12).22 Unless otherwise noted, the following reactions with liquid additives are all characterized by the addition of 0.20 μL of liquid per mg of reactant. We found that adding a small amount of tetrahydrofuran (THF) greatly improved the yield of 3a (71% yield; entry 3). Other commonly used organic solvents in palladium-catalyzed cross-coupling reactions such as toluene, acetonitrile (CH3CN), dioxane, dimethyl formamide (DMF), and diethyl ether (Et2O) also improved the efficiency of the reaction (entries 4–8). The use of highly polar solvents such as dimethyl sulfoxide (DMSO) or simple alkanes (pentane and cyclohexane) slightly accelerated the oxidative addition (entries 9–11). Protic solvents such as methanol (MeOH) did not promote the reaction (entry 12). Subsequently, we focused on the identification of the optimal milling parameters for this mechanochemical oxidative addition reaction (entries 13–15). The milling frequency can be reduced to 25 Hz (78% yield; entry 13), while increasing the number of stainless-steel balls did not improve the yield (entries 14 and 15). Notably, the reaction time can be reduced to 10 min without affecting the yield when THF was used as a LAG additive (71% yield; entry 16).
Table 1 Optimization of the reaction conditionsa
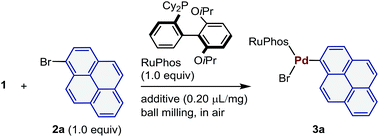
|
Entry |
LAG |
Milling frequency (Hz) |
Time (min) |
NMR yieldb (%) |
Conditions: 1 (0.05 mmol), 2a (0.05 mmol), RuPhos (0.05 mmol), and the LAG additive (0.2 μL mg−1) in a stainless-steel ball-milling jar (1.5 mL) with a stainless-steel ball (3 mm).
Determined by 1H NMR analysis of the crude reaction mixture using an internal standard.
Two stainless-steel balls (3 mm) were used.
Three stainless-steel balls (3 mm) were used.
Isolated yield.
|
1 |
None |
30 |
30 |
40 |
2 |
None |
30 |
60 |
43 |
3 |
THF |
30 |
30 |
71 |
4 |
Toluene |
30 |
30 |
61 |
5 |
CH3CN |
30 |
30 |
71 |
6 |
Dioxane |
30 |
30 |
64 |
7 |
DMF |
30 |
30 |
64 |
8 |
Et2O |
30 |
30 |
66 |
9 |
DMSO |
30 |
30 |
55 |
10 |
Pentane |
30 |
30 |
58 |
11 |
Cyclohexane |
30 |
30 |
47 |
12 |
MeOH |
30 |
30 |
48 |
13 |
THF |
25 |
30 |
78e |
14c |
THF |
25 |
30 |
55 |
15d |
THF |
25 |
30 |
67 |
16 |
THF |
25 |
10 |
71 |
With the optimized conditions in hand, we investigated the effect of different halides and a pseudohalide on the oxidative addition of palladium complexes (Scheme 2). Even though the corresponding aryl chloride (2b) undergoes oxidative addition in solution, the desired oxidative addition complex (3b) was not formed under mechanochemical conditions.4 When bromide (2c) and the iodide substrates (2d) were used, the products were obtained in 62% and 75% yield, respectively. Although Ondruschka and co-workers reported that aryl bromides were more reactive than aryl iodides in mechanochemical palladium-catalyzed Suzuki–Miyaura coupling reactions,23 the iodide substrate (2d) provided higher yield in the mechanochemical stoichiometric oxidative addition process. The corresponding aryl triflate (2e) afforded 3e in moderate yield (58%).
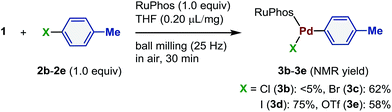 |
| Scheme 2 Mechanochemical synthesis of palladium-based oxidative addition complexes from aryl halides and an aryl pseudohalide. | |
The aforementioned optimized conditions were used for further evaluation of the substrate scope (Table 2). The reaction of both electron-rich and -poor aryl bromides (2f–2j) proceeded smoothly to give the corresponding oxidative addition complexes (3f–3j) in good yields (57–76%). 4-Bromophenol (2k) also afforded the desired product (3k) in moderate yield (35%). The present protocol could also be applied to heteroaryl bromides such as indole (2l), thiophene (2m), and dibenzofuran (2n) derivatives. Palladium(II) complexes containing aromatic hydrocarbon cores (3a, 3o, and 3p) were obtained in 78–92% yield. The reaction of aryl bromides bearing aldehyde 2q and benzophenone moieties 2r afforded the desired palladium(II) complexes (3q and 3r), which are used in bioconjugation applications,4 in 79% and 61% yield, respectively.
Conditions: 1 (0.12 mmol), 2 (0.12 mmol), RuPhos (0.12 mmol), and THF (0.2 μL mg−1) in a stainless-steel ball-milling jar (1.5 mL) with a stainless-steel ball (3 mm).
|
|
Polymetalated transition-metal complexes have attracted considerable attention due to their unique reactivity and physical properties.4e,24 The mechanochemical oxidative addition protocol developed herein provides an efficient route for the synthesis of polymetalated palladium complexes (Table 3). The reaction of dibromides 2s and 2t in the presence of 2 equiv. of 1 and RuPhos afforded di-palladated 3s and 3t, which are useful reagents for macrocyclization of peptides,4e in 88% and 85% yield, respectively. The tri-palladated arylamine 3u and the tetra-palladated pyrene 3v were synthesized in 91% and 61% yield, respectively under the applied mechanochemical conditions in air. The molecular structure of 3v in the solid state was unequivocally determined by a single-crystal X-ray diffraction analysis (Fig. 1). The results revealed that two of the four palladium atoms in 3v adopt a C-bound conformation, while the other two adopt an O-bound conformation in the solid state. The orientation of the phosphine ligands on adjacent palladium atoms is in opposing directions relative to each other, which is probably due to the steric congestion between substituents on the phosphine ligands in the solid state.
Table 3 Multiple oxidative additions using mechanochemistrya
See the ESI for the details of the reaction conditions.
|
|
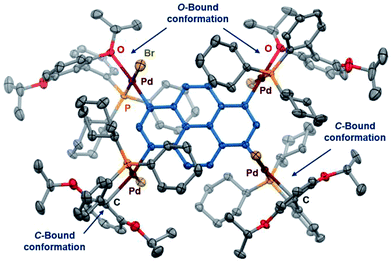 |
| Fig. 1 Molecular structure of 3v in the solid state (thermal ellipsoids at 50% probability; hydrogen atoms and CH2Cl2 are omitted for clarity). | |
Subsequently, we investigated the effect of the phosphine ligand on the mechanochemical palladium-mediated oxidative addition of 2f (Table 4). The reactions using 2-dicyclohexylphosphino-2′,6′-dimethoxybiphenyl (SPhos) and 2-dicyclohexylphosphino-2′,4′,6′-triisopropylbiphenyl (XPhos) furnished 3w and 3x in 44% and 32% yield, respectively.
Conditions: 1 (0.12 mmol), 2f (0.12 mmol), ligand (0.12 mmol), and THF (0.2 μL mg−1) in a stainless-steel ball-milling jar (1.5 mL) with a stainless-steel ball (3 mm).
|
|
These palladium-based oxidative addition complexes can also be prepared on the gram-scale in air using mechanochemistry (Scheme 3). For this purpose, the reaction of 2s (1 mmol) was carried out in a larger stainless-steel ball-milling jar (25 mL) with two stainless-steel balls (10 mm) in air, and the desired oxidative addition complex (3s) was obtained in 85% yield (1.26 g). This result clearly demonstrates the potential utility of the present mechanochemical protocol for large-scale preparation of synthetically useful palladium reagents without any special operational skills or precautions.
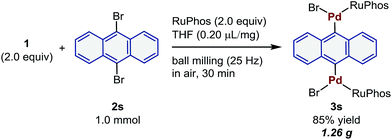 |
| Scheme 3 Gram-scale synthesis of 3s. Conditions: 1 (2.0 mmol), 2s (1.0 mmol), RuPhos (2.0 mmol), and THF (0.20 μL mg−1) in a stainless-steel ball-milling jar (25 mL) with two stainless-steel balls (10 mm). | |
In order to further explore the practical utility of this protocol, we compared the efficiency of the solution-based and the mechanochemical reactions (Scheme 4). The oxidative addition of 2o under the reported solution-based conditions using cyclohexane or THF as solvents3a,4 provided 3o in 61% and 87% yield, respectively. However, when the reactions were conducted in air, significantly lower yields were obtained (8% and 46%, respectively). In contrast, under the aforementioned mechanochemical conditions, the oxidative addition proceeded efficiently, even in air, providing 3o in 92% yield. These results suggest that gaseous oxygen does not efficiently diffuse through crystalline or amorphous solid-state reaction mixtures,25,26 which results in little to no impact on the air-sensitive palladium(0)-mediated oxidative addition reactions. Related to this finding, Mack and co-workers have described that the gaseous nature of water and oxygen does not significantly influence moisture-sensitive organic transformations under mechanochemical conditions.25
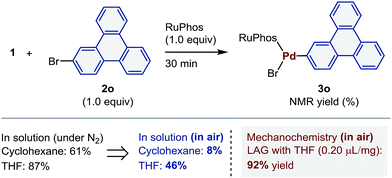 |
| Scheme 4 Comparison of the performance of solution-based and mechanochemical reactions. | |
Conclusions
Palladium-based oxidative addition complexes are widely used in mechanistic studies, as catalyst precursors and as stoichiometric aryl-group-transfer reagents. However, the preparation of these complexes generally requires glove-box or Schlenk-line techniques due to the high air-sensitivity of the palladium(0) species involved. We have demonstrated that mechanochemistry allows synthesizing a wide range of Buchwald-type-phosphine-ligated palladium(II)-based oxidative addition complexes in air. The low diffusion efficiency of gaseous oxygen in the solid-state reaction mixture was identified as the most likely reason for the low impact of atmospheric oxygen on these sensitive organometallic reactions. This study illustrates the outstanding potential of mechanochemistry as an operationally simple glove-box-and-Schlenk-line-free synthetic route to valuable organometallic compounds and other attractive synthetic targets, even when highly sensitive reagents or intermediates are involved.
Conflicts of interest
There are no conflicts to declare.
Acknowledgements
This work was financially supported by the Japan Society for the Promotion of Science (JSPS) via KAKENHI grants JP18H03907, JP17H06370 and JP19K15547, as well as by the Institute for Chemical Reaction Design and Discovery (ICReDD), which has been established by the World Premier International Research Center Initiative (WPI), MEXT, Japan. We would like to thank Dr Tomohiro Seki and Takaki Mashimo for their help in analysing the X-ray crystallography data.
Notes and references
-
The Manipulations of Air-Sensitive Compounds, ed. D. F. Shriver and M. A. Drezdon, John Wiley and Sons, New York, 2nd edn, 1986 Search PubMed.
- For selected examples of mechanistic studies using palladium-based oxidative addition complexes, see:
(a) D. A. Watson, M. Su, G. Teverovskiy, Y. Zhang, J. García-Fortanet, T. Kinzel and S. L. Buchwald, Science, 2009, 325, 1661 CrossRef CAS PubMed;
(b) T. J. Maimone, R. J. Milner, T. Kinzel, Y. Zhang, M. K. Takase and S. L. Buchwald, J. Am. Chem. Soc., 2011, 133, 18106 CrossRef CAS PubMed;
(c) A. C. Sather, H. G. Lee, V. Y. De La Rosa, Y. Yang, P. Müller and S. L. Buchwald, J. Am. Chem. Soc., 2015, 137, 13433 CrossRef CAS PubMed;
(d) P. L. Arrechea and S. L. Buchwald, J. Am. Chem. Soc., 2016, 138, 12486 CrossRef CAS PubMed;
(e) J. M. Dennis, N. A. White, R. Y. Liu and S. L. Buchwald, J. Am. Chem. Soc., 2018, 140, 4721 CrossRef CAS PubMed;
(f) A. H. Roy and J. F. Hartwig, J. Am. Chem. Soc., 2001, 123, 1232 CrossRef CAS PubMed;
(g) A. H. Roy and J. F. Hartwig, J. Am. Chem. Soc., 2003, 125, 13944 CrossRef CAS PubMed;
(h) K. Fujita, M. Yamashita, F. Puschmann, M. M. Alvarez-Falcon, C. D. Incarvito and J. F. Hartwig, J. Am. Chem. Soc., 2006, 128, 9044 CrossRef CAS PubMed;
(i) M. Yamashita and J. F. Hartwig, J. Am. Chem. Soc., 2004, 126, 5344 CrossRef CAS PubMed;
(j) D. M. Peacock, Q. Jiang, P. S. Hanley, T. R. Cundari and J. F. Hartwig, J. Am. Chem. Soc., 2018, 140, 4893 CrossRef CAS PubMed;
(k) A. Maleckis and M. S. Sanford, Organometallics, 2014, 33, 2653 CrossRef CAS;
(l) A. Maleckis, J. W. Kampf and M. S. Sanford, J. Am. Chem. Soc., 2013, 135, 6618 CrossRef CAS PubMed;
(m) J. M. Racowski, N. D. Ball and M. S. Sanford, J. Am. Chem. Soc., 2011, 133, 18022 CrossRef CAS PubMed;
(n) J. F. Hartwig and F. Paul, J. Am. Chem. Soc., 1995, 117, 5373 CrossRef CAS;
(o) M. Portnoy and D. Milstein, Organometallics, 1993, 12, 1665 CrossRef CAS;
(p) C. Amatore and F. Pflüger, Organometallics, 1990, 9, 2276 CrossRef CAS;
(q) W. R. Moser, A. W. Wang and N. K. Kildahl, J. Am. Chem. Soc., 1988, 110, 2816 CrossRef CAS;
(r) T. L. Andersen, S. Kramer, J. Overgaard and T. Skrydstrup, Organometallics, 2017, 36, 2058 CrossRef CAS.
- For selected examples of the use of palladium-based oxidative addition complexes as catalyst precursors, see:
(a) B. T. Ingoglia and S. L. Buchwald, Org. Lett., 2017, 19, 2853 CrossRef CAS PubMed;
(b) J. Vicente, A. Arcas, F. Juliá-Hernández and D. Bautista, Angew. Chem., Int. Ed., 2011, 50, 6896 CrossRef CAS PubMed;
(c) A. Yokoyama, H. Suzuki, Y. Kubota, K. Ohuchi, H. Higashimura and T. Yokozawa, J. Am. Chem. Soc., 2007, 129, 7236 CrossRef CAS PubMed.
- For selected examples of the use of palladium-based oxidative addition complexes as stoichiometric aryl-group-transfer reagents for the diversification of biomolecules and pharmaceuticals, see:
(a) E. V. Vinogradova, C. Zhang, A. M. Spokoyny and B. L. Pentelute, Nature, 2015, 526, 687 CrossRef CAS PubMed;
(b) W. Zhao, H. G. Lee, S. L. Buchwald and J. M. Hooker, J. Am. Chem. Soc., 2017, 139, 7152 CrossRef CAS PubMed;
(c) A. J. Rojas, B. L. Pentelute and S. L. Buchwald, Org. Lett., 2017, 19, 4263 CrossRef CAS PubMed;
(d) H. G. Lee, G. Lautrette, B. L. Pentelute and S. L. Buchwald, Angew. Chem., Int. Ed., 2017, 56, 3177 CrossRef CAS PubMed;
(e) A. J. Rojas, C. Zhang, E. V. Vinogradova, N. Buchwald, J. Reilly, B. L. Pentelute and S. L. Buchwald, Chem. Sci., 2017, 8, 4257 RSC;
(f) K. Kubota, P. Dai, B. L. Pentelute and S. L. Buchwald, J. Am. Chem. Soc., 2018, 140, 3128 CrossRef CAS PubMed;
(g) M. R. Uehling, R. P. King, S. W. Krska, T. Cernak and S. L. Buchwald, Science, 2019, 363, 405 CrossRef CAS PubMed.
-
(a) Y. Pan and G. B. Young, J. Organomet. Chem., 1999, 577, 257 CrossRef CAS;
(b) J. R. McAtee, S. E. S. Martin, D. T. Ahneman, K. A. Johnson and D. A. Watson, Angew. Chem., Int. Ed., 2012, 51, 3663 CrossRef CAS PubMed.
- For recent reviews on organic syntheses using mechanochemistry, see:
(a) S. L. James, C. J. Adams, C. Bolm, D. Braga, P. Collier, T. Friščić, F. Grepioni, K. D. M. Harris, G. Hyett, W. Jones, A. Krebs, J. Mack, L. Maini, A. G. Orpen, I. P. Parkin, W. C. Shearouse, J. W. Steed and D. C. Waddell, Chem. Soc. Rev., 2012, 41, 413 RSC;
(b) G.-W. Wang, Chem. Soc. Rev., 2013, 42, 7668 RSC;
(c) T. Friščić, I. Halasz, V. Štrukil, M. Eckert-Maksić and R. E. Dinnebier, ACS Cent. Sci., 2017, 3, 13 CrossRef PubMed;
(d) J. G. Hernández and C. Bolm, J. Org. Chem., 2017, 82, 4007 CrossRef PubMed;
(e) J. G. Hernández, Chem.–Eur. J., 2017, 23, 17157 CrossRef;
(f) T.-X. Métro, J. Martinez and F. Lamaty, ACS Sustainable Chem. Eng., 2017, 5, 9599 CrossRef;
(g) T. K. Achar, A. Bose and P. Mal, Beilstein J. Org. Chem., 2017, 13, 1907 CrossRef CAS;
(h) J.-L. Do and T. Friščić, Synlett, 2017, 28, 2066 CrossRef CAS;
(i) D. Tan and T. Friščić, Eur. J. Org. Chem., 2018, 18 CrossRef CAS;
(j) O. Eguaogie, J. S. Vyle, P. F. Conlon, M. A. Gîlea and Y. Liang, Beilstein J. Org. Chem., 2018, 14, 955 CrossRef CAS PubMed;
(k) J. L. Howard, Q. Cao and D. L. Browne, Chem. Sci., 2018, 9, 3080 RSC;
(l) J. Andersen and J. Mack, Green Chem., 2018, 20, 1435 RSC;
(m) N. R. Rightmire and T. P. Hanusa, Dalton Trans., 2016, 45, 2352 RSC;
(n) M. Leonardi, M. Villacampa and J. C. Menéndez, Chem. Sci., 2018, 9, 2042 RSC.
- For the mechanochemical synthesis of gold complexes, see:
(a) A. Zhdanko, M. Ströbele and M. E. Maier, Chem.–Eur. J., 2012, 18, 14732 CrossRef CAS PubMed;
(b) J. D. Egbert, A. M. Z. Slawin and S. P. Nolan, Organometallics, 2013, 32, 2271 CrossRef CAS.
- For the mechanochemical synthesis of ferrocene, see: V. D. Makhaev, A. P. Borisov and L. A. Petrova, J. Organomet. Chem., 1999, 590, 222 CrossRef CAS.
- For the
mechanochemical synthesis of rhenium and manganese complexes, see: J. G. Hernández, I. S. Butler and T. Friščić, Chem. Sci., 2014, 5, 3576 RSC.
- For the mechanochemical oxidative addition of rhenium complexes, see: J. G. Hernández, N. A. J. Macdonald, C. Mottillo, I. S. Butler and T. Friščić, Green Chem., 2014, 16, 1087 RSC.
- For the mechanochemical synthesis of nickel and cobalt complexes, see: C. S. B. Gomes, P. T. Gomes and M. T. Duarte, J. Organomet. Chem., 2014, 760, 101 CrossRef CAS.
- For the related mechanochemical synthesis of palladium complexes, see:
(a) D. Cinčić, M. Juribašić, D. Babić, K. Molčanov, P. Šket and M. Ćurić, Chem. Commun., 2011, 47, 11543 RSC;
(b) M. Juribašić, K. Užarević, D. Gracin and M. Ćurić, Chem. Commun., 2014, 50, 10287 RSC.
- For the mechanochemical synthesis of zinc complexes, see: J. Lewiński, M. Dutkiewicz, M. Lesiuk, W. Śliwiński, K. Zelga, I. Justyniak and J. Lipkowski, Angew. Chem., Int. Ed., 2010, 49, 8266 CrossRef PubMed.
- Very recently, Browne and co-workers have reported the mechanochemical synthesis of organozinc species in air; see: Q. Cao, J. L. Howard, E. Wheatley and D. L. Browne, Angew. Chem., Int. Ed., 2018, 57, 11339 CrossRef CAS PubMed.
- For the mechanochemical synthesis of gold–silver honeycomb aggregates, see: M. C. Blanco, J. Cámara, M. C. Gimeno, A. Laguna, S. T. James, M. C. Lagunas and M. D. Villacampa, Angew. Chem., Int. Ed., 2012, 51, 9777 CrossRef CAS PubMed.
- For the mechanochemical synthesis of aluminium complexes, see: N. R. Rightmire, T. P. Hanusa and A. L. Rheingold, Organometallics, 2014, 33, 5952 CrossRef CAS.
- For the mechanochemical synthesis of Grignard reagents, see: J. M. Harrowfield, R. J. Hart and C. R. Whitaker, Aust. J. Chem., 2001, 54, 423 CrossRef CAS.
- For the mechanochemical synthesis of an iridacycle, see: G. N. Herman, P. Becker and C. Bolm, Angew. Chem., Int. Ed., 2016, 55, 3781 CrossRef PubMed.
- For the mechanochemical synthesis of a rhodacycle, see: G. N. Herman and C. Bolm, ACS Catal., 2017, 7, 4592 CrossRef.
- For selected recent examples of palladium-catalyzed cross-coupling reactions in air using mechanochemistry, see:
(a) Q. Cao, W. I. Nicholson, A. C. Jones and D. L. Browne, Org. Biomol. Chem., 2019, 17, 1722 RSC;
(b) Q.-L. Shao, Z.-J. Jiang and W.-K. Su, Tetrahedron Lett., 2018, 59, 2277 CrossRef CAS;
(c) K. Kubota, T. Seo, K. Koide, Y. Hasegawa and H. Ito, Nat. Commun., 2019, 10, 111 CrossRef PubMed;
(d) V. Declerck, E. Colacino, X. Bantreil, J. Martinez and F. Lamaty, Chem. Commun., 2012, 48, 11778 RSC;
(e) G. Cravotto, D. Garella, S. Tagliapietra, A. Stolle, S. Schüβler, S. E. S. Leonhardt and B. Ondruschka, New J. Chem., 2012, 36, 1304 RSC;
(f) Z.-J. Jiang, Z.-H. Li, J.-B. Yu and W.-K. Su, J. Org. Chem., 2016, 81, 10049 CrossRef CAS PubMed;
(g) H. Cheng, J. G. Hernández and C. Bolm, Org. Lett., 2017, 19, 6284 CrossRef CAS PubMed.
-
(a) D. W. Old, J. P. Wolfe and S. L. Buchwald, J. Am. Chem. Soc., 1998, 120, 9722 CrossRef CAS;
(b) R. Martin and S. L. Buchwald, Acc. Chem. Res., 2008, 41, 1461 CrossRef CAS.
- T. Friščić, S. L. Childs, S. A. A. Rizvi and W. Jones, CrystEngComm, 2009, 11, 418 RSC.
- F. Schneider and B. Ondruschka, ChemSusChem, 2008, 1, 622 CrossRef CAS PubMed.
-
(a) J. Vicente and M. Lyakhovych, Organometallics, 2001, 20, 4695 CrossRef CAS;
(b) J. Vicente, R. V. Shenoy and E. Martínez-Viviente, Organometallics, 2009, 28, 6101 CrossRef CAS;
(c) J. Vicente, R. V. Shenoy, E. Martínez-Viviente and P. G. Jones, Inorg. Chem., 2011, 50, 7189 CrossRef CAS PubMed.
- D. C. Waddell, T. D. Clark and J. Mack, Tetrahedron Lett., 2012, 53, 4510 CrossRef CAS.
- C. Bolm and J. D. Hernández, Angew. Chem., Int. Ed., 2019, 58, 3285 CrossRef CAS PubMed.
Footnote |
† Electronic supplementary information (ESI) available. CCDC 1908179. For ESI and crystallographic data in CIF or other electronic format see DOI: 10.1039/c9sc01711a |
|
This journal is © The Royal Society of Chemistry 2019 |
Click here to see how this site uses Cookies. View our privacy policy here.