DOI:
10.1039/C7QM00172J
(Research Article)
Mater. Chem. Front., 2017,
1, 1841-1846
Ultrasensitive water sensors based on fluorenone-tetraphenylethene AIE luminogens†
Received
19th April 2017
, Accepted 25th April 2017
First published on 27th April 2017
Abstract
Two solvatochromic fluorenone-tetraphenylethene luminogens, 2-(4-(1,2,2-triphenylvinyl)phenyl)-9H-fluoren-9-one (TPE-FO) and 2,7-bis(4-(1,2,2-triphenylvinyl)phenyl)-9H-fluoren-9-one (TPE-FO-TPE), have been designed and synthesized, in which tetraphenylethene offers dual functions, i.e., aggregation-induced emission (AIE) and electron donation. Both TPE-FO and TPE-FO-TPE exhibit pronounced solvatochromic effects and AIEE activity. The two luminogens exhibit ultra-high sensitivity to trace water in organic solvents such as THF or dioxane. The Stern–Volmer plots show good linear relationships with water fractions below 0.9%. The detection limit of TPE-FO for water in THF is calculated to be 11 ppm which is pretty close to that of Karl–Fischer titration.
Introduction
In 2001, Tang’s group reported a phenomenon of aggregation-induced emission (AIE), which is opposite to the notorious aggregation-caused quenching (ACQ) suffered by many traditional luminescent materials owing to the intense intermolecular π–π stacking interactions.1,2 Since then, AIE luminogens (AIEgens) as a kind of burgeoning materials have shown widespread applications in light-emitting diodes, luminescent sensors, biomedical imaging, photonic devices, etc.2 Among those AIEgens, tetraphenylethene (TPE) derivatives have attracted much interest owing to their excellent AIE performance and accessibility of structure modification. Additionally, TPE derivatives possess high fluorescence quantum yield and mechanochromism properties in solid state.3–5 They have also been used as building blocks to form various superstructures via self-assembly.6–11 Moreover, the TPE group possesses the magic ability of converting traditional ACQ luminogens, such as pyrene and perylenebisimide, to AIE-active molecules when TPE is incorporated into the structures of ACQ molecules.2 Besides AIE activity, TPE was found to be an electron donor, and has been utilized to construct luminogens exhibiting intramolecular charge transfer (ICT) behaviours.12–22 In previous work, we reported the first example of naphthalimide-TPE luminogens which exhibit both AIE and ICT behaviours without additional electron donating groups in the molecule structure.12 Generally, a TPE-based ICT system can be designed by connecting the TPE group with electron-donating ability to an electron accepting group. Fluorenone (FO), which is an inexpensive and commercially available starting material, possesses a relatively planar structure, accessibility of structure modification, and good electron-transport properties, which made it an interesting electron-deficient candidate for designing a D–A system. Furthermore, FO and its derivatives are very sensitive to both intramolecular and intermolecular interactions, such as hydrogen bonding, polarity and steric interactions.23 However, FO-based luminogens with AIE property are still quite rare.24–27 Therefore, it is important to utilize the TPE as an electron donor group and FO as an electron accepting group to build smart luminogens with AIE and solvatochromic effects, which might exhibit high sensitivity and large response to external stimuli.25
In recent decades, a great deal of attention has been paid to determination of water content in organic solvents on account of its crucial role in chemical reactions and industrial applications, e.g., food, textile, ceramics, electronic, pharmaceutical, petroleum, and environmental monitoring industries.28–30 The most broadly applied water determination technique is the Karl–Fischer titration, which was developed in the 1930s and can detect water down to about 1 ppm.31 Incontestably, this method features high sensitivity and wide applicability for a large variety of samples. However, there are also some limitations, including very time-consuming process, the use of toxic and expensive chemical reagents, difficulty in end point determination, interference by other common species, and so on.32 In order to overcome these drawbacks, many kinds of water sensors based on various sensing mechanisms have been developed. Among them, optical water sensors based on fluorescence spectroscopy are particularly eye-catching because of their high sensitive, short time-consuming, inexpensive, easy processing and non-destructive nature as well as the capability of remote and in situ monitoring of optical measurements.28,29 Most of the fluorescent sensors for trace water detection are based on fluorescent conjugated polymer or organic fluorescent small molecular dyes which can exhibit fluorescence responses to trace water in organic solvents.29 The sensing mechanisms of these fluorescent sensors are diverse, such as intramolecular charge transfer, proton transfer, photo-induced electron transfer, water-induced decomplexation of fluorophores with anions, water induced interpolymer π-stacking aggregation and solvatochromism.29 Among them, the solvatochromism and hydrogen bonding interactions play important roles in the determination of trace water using these fluorescent sensors. It shows great potential to develop ultrasensitive water sensors with limits of detection close to that of Karl–Fischer titration.33
Herein, two solvatochromic luminogens 2-(4-(1,2,2-triphenylvinyl)phenyl)-9H-fluoren-9-one (TPE-FO) and 2,7-bis(4-(1,2,2-triphenylvinyl)phenyl)-9H-fluoren-9-one (TPE-FO-TPE) were designed and synthesized. The synthetic routes to these TPE derivatives are shown in Scheme 1. The experiment details and characterization are described in the experimental section. Both luminogens exhibit pronounced solvatochromism and AIEE effect. Moreover, they show ultrasensitivity and evident fluorescence response to water in THF and dioxane, and are able to be used as detectors for trace water determination in organic solvents.
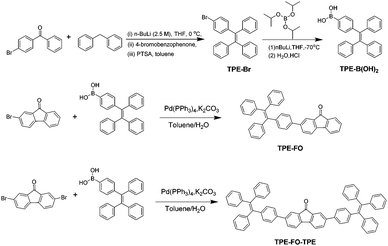 |
| Scheme 1 Synthesis route to compounds TPE-FO and TPE-FO-TPE. | |
Experimental
Reagents and materials
All commercially available starting materials, reagents and solvents were used as supplied, unless otherwise stated, and were purchased from Energy, Alfa Aesar, J&K and Zhengzhou Huawen Chemical Co. Ltd. Toluene, dioxane and tetrahydrofuran (THF) were dried using sodium pieces and benzophenone indicator. Tri(isopropyl)borate was distilled over sodium pieces. All reactions were carried out under a dry nitrogen atmosphere and the temperatures were measured externally. Reported yields are isolated yields. Purification of all final products was accomplished by gravity column chromatography, using silica gel. For qualitative purity tests of all intermediates and final products, a single spot (visualised using UV-light at 254 nm and 365 nm) was obtained.
Characterization
NMR spectra were recorded on a 600 M Bruker AV600 using CDCl3 as the solvent. Mass Spectrometry was performed on Agilent (1100 LC/MSD Trap). The UV-Vis absorption and photoluminescence emission of the compounds were recorded on the Shimadzu UV-VIS-NIR Spectrophotometer (UV-3600) and Edinburgh spectrometers (FLS 920), respectively. Fluorescence lifetimes were measured based on the time correlated single photon counting (TCSPC), and recorded on the Edinburgh FLS 920 spectrometers. The scanning electron microscopy pictures were collected on a FEI Nova NanoSEM 450. The relative fluorescence quantum yields were estimated using quinine sulfate in 0.1 M H2SO4 solution as a standard.
Synthesis
1-(4-Bromophenyl)-1,2,2-triphenylethylene (TPE-Br) and 4-(1,2,2-triphenylvinyl)phenylboronic acid (TPEB(OH)2).
TPE-Br and TPEB(OH)2 were synthesized by following the previously reported procedures.12–14
2-(4-(1,2,2-Triphenylvinyl)phenyl)-9H-fluoren-9-one (TPE-FO).
A mixture of TPE-B(OH)2 (0.45 g, 1.2 mmol), 2-bromo-9H-fluoren-9-one (0.26 g, 1 mmol), Pd(PPh3)4 (0.06 g, 0.05 mmol), PTC ((C4H9)4NHSO4) (34 mg, 0.1 mmol), K2CO3 (0.21 g, 1.5 mmol) in toluene (20 mL) and water (10 mL) was heated to reflux and stirred under nitrogen overnight. After cooling down to room temperature, the organic layer was separated and the water layer was extracted with dichloromethane (DCM). The combined organic solution was dried with Na2SO4. After filtration, the resulting solution was concentrated on a rotary evaporator. The residue was purified by column chromatography on silica gel using DCM/PE (v/v: 5
:
5) as the eluent to obtain TPE-FO (85%, yield) as an orange powder. 1H NMR (600 MHz, CDCl3) 7.85 (s, 1H), 7.71–7.64 (m, 2H), 7.56–7.47 (m, 3H), 7.37 (d, J = 8.3 Hz, 2 H), 7.29 (t, J = 7.2 Hz, 1H), 7.17–7.01 (m, 17H). 13C NMR (150 MHz, CDCl3) 193.85, 144.33, 143.63, 143.60, 143.56, 143.53, 143.03, 141.83, 141.44, 140.28, 137.51, 134.82, 134.79, 134.48, 132.87, 131.94, 131.39, 131.31, 128.96, 127.80, 127.73, 127.64, 126.62, 126.54, 126.48, 125.90, 124.39, 122.77, 120.60, 120.35. MS (APCI) calcd for C39H26O: 510.20; found: [M + 1]+ 511.2.
2,7-bis(4-(1,2,2-Triphenylvinyl)phenyl)-9H-fluoren-9-one (TPE-FO-TPE).
A mixture of TPE-B(OH)2 (0.86 g, 2.3 mmol), 2,7-dibromo-9H-fluoren-9-one (0.34 g, 1 mmol), Pd(PPh3)4 (0.06 g, 0.05 mmol), PTC ((C4H9)4NHSO4) (34 mg, 0.1 mmol), K2CO3 (0.414 g, 3 mmol) in toluene (20 mL) and water (10 mL) was heated to reflux and stirred under nitrogen overnight. After cooling down to room temperature, the organic layer was separated and the water layer was extracted with dichloromethane (DCM). The combined organic solution was dried with Na2SO4. After filtration, the resulting solution was concentrated on a rotary evaporator. The residue was purified by column chromatography on silica gel using DCM/PE (v/v: 5
:
5) as the eluent to obtain TPE-FO-TPE (78%, yield) as a yellow powder. 1H NMR (600 MHz, CDCl3) δ 7.86 (s, 2H), 7.68 (dd, J = 7.8, 1.3 Hz, 2H), 7.54 (d, J = 7.8 Hz, 2H), 7.37 (d, J = 8.2 Hz, 4H), 7.16–7.01 (m, 34H). 13C NMR (150 MHz, CDCl3) δ 193.77, 143.63, 143.59, 143.55, 143.53, 142.91, 141.70, 141.45, 140.28, 137.49, 135.14, 132.96, 131.94, 131.39, 131.31, 127.80, 127.73, 127.64, 126.62, 126.54, 126.48, 125.88, 122.81, 120.65. MS (APCI) calcd for C65H44O: 841.07; found: [M + 1]+ 842.8.
Results and discussion
Solvatochromic effect
TPE-FO and TPE-FO-TPE possess typical D–A and D–A–D structures respectively, in which the fluorenone group plays the role of electron acceptor against the electron donating TPE. The formation of a D–A structure will strongly affect the photophysics properties of fluorenone-containing TPE in solution, which is strongly dependent on the solvent polarity. Fig. 1 shows the fluorescence spectra of TPE-FO and TPE-FO-TPE in solvents varying in polarity and the detailed optical data are summarized in Table S1 (ESI†). From non-polar solvents (i.e., cyclohexane) to polar solvents (i.e., DMSO), strong solvent-dependent emission of TPE-FO, ranging from 504 nm to 594 nm, is demonstrated, exhibiting a remarkable bathochromic effect (Fig. 1 and Table S1, ESI†). Also, the remarkable solvatochromic phenomenon can be observed from the fluorescence photos (Fig. 1). Meanwhile, the fluorescence quantum yield changes with the increase of solvent polarity (TPE-FO: 21% to 0%, from hexane to DMSO, Table S1, ESI†). In more polar solvents such as DMSO, the fluorescence of TPE-FO is nearly completely quenched and the emission peak shifts further to the red region. In contrast, the absorption spectra of TPE-FO displayed minor changes in different solvents (Fig. S1, ESI†). Similarly, with the increase of solvent polarity, TPE-FO-TPE with a D–A–D structure shows the maximum emission wavelength bathochromic shift from 530 nm to 592 nm, and fluorescence quantum yield changes from 36.4% to 1.7%. Compared with TPE-FO, the emission wavelength of TPE-FO-TPE red-shifts resulting from the difference in conjugated structure between TPF-FO and TPE-FO-TPE.
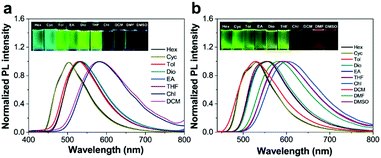 |
| Fig. 1 Normalized fluorescence spectra of TPE-FO (a) and TPE-FO-TPE (b) in different solvents. The insets show fluorescence photographs of (a) TPE-FO and (b) TPE-FO-TPE in organic solvents with different polarity. Hex, hexane; Cyc, cyclohexane; Tol, toluene; EA, ethyl acetate; Dio, dioxane; THF, tetrahydrofuran; Chl, chloroform; DCM, dichloromethane; DMF, dimethylformamide; DMSO, dimethylsulfoxide. | |
To gain a better understanding of the solvent effects of TPE-FO and TPE-FO-TPE, theoretical calculations were performed based on the density functional theory (DFT) using the B3LYP/6-31G(d) basis set. Fig. 2 shows the optimized molecular configurations, energy levels, and electron distributions of the highest occupied molecular orbital (HOMO) and the lowest unoccupied molecular orbital (LUMO) of TPE-FO and TPE-FO-TPE. The electronic cloud of LUMO is located mostly at the fluorenone core for both TPE-FO and TPE-FO-TPE. Notably, the electronic cloud of the HOMO of TPE-FO-TPE is delocalized over the conjugated backbone, while that of TPE-FO is located mostly at the TPE group and only a small part is on the fluorenone group. This indicates that TPE-FO has a more charge separated D–A structure. What’s more, the dihedral angles between the fluorenone and the linked phenyl rings of the TPE group are 36.2° and 26.1° for TPE-FO and TPE-FO-TPE, respectively, indicating a more conjugated structure of the latter. The energy gaps of TPE-FO and TPE-FO-TPE were calculated to be 3.07 eV and 2.98 eV, respectively (Table S3, ESI†).
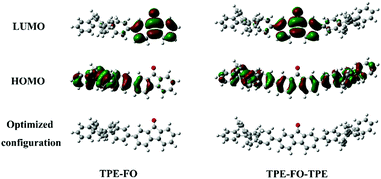 |
| Fig. 2 Optimized electronic structures of TPE-FO and TPE-FO-TPE. | |
Aggregation-induced enhanced emission (AIEE)
The AIEE properties of TPE-FO and TPE-FO-TPE were investigated by employing anhydrous DMSO as a good solvent and water as a poor solvent. It is clearly seen that TPE-FO and TPE-FO-TPE both show weak emission in the pure DMSO. The fluorescence intensity of TPE-FO and TPE-FO-TPE decreases lightly when the water fraction in DMSO is below 40% and 30% for TPE-FO and TPE-FO-TPE respectively, which could be attributed to an enhanced ICT caused by the increased solvent polarity (Fig. 3). However, as the water fraction continues to increase, the fluorescence intensity sharply increases. The maximum fluorescence intensity will be reached when the water fraction increases up to 60% where the aggregations of the luminogens are formed. The formation of aggregates can be verified by the appearance of a level-off tail in the visible region of their absorption spectra (Fig. S2, ESI†) and the scanning electron microscopy (SEM) images of samples in high water fraction solutions (Fig. S3, ESI†). Dynamic Light Scattering (DLS) analyses also indicate the formation of aggregates (Fig. S4, ESI†). The slight decrease of fluorescence intensity in the water fraction above 60% could be attributed to the agglomeration of nanoparticles. The AIEE phenomena, observed from DMSO–water solvent mixture measurements, are comprehensible. The TPE group plays an important role in the AIE effect of the two luminogens, owing to their twisted propeller-shaped molecular structures. When a large amount of water was added to DMSO, the luminogen molecules tended to aggregate and formed nanoparticles. Thus, the result is that the rotational and vibrational motions of the phenyl rings around the TPE double bond are restricted, which blocks nonradiative relaxation pathways and enables enhanced emission of the aggregates.2 What’s more, the anti-ICT process also contributed to the AIEE effect. The formation of aggregated nanoparticles forces the molecules of luminogens to escape from the polar environment and thus the polarity inside nanoparticles is lower than that outside.2,34 With the decrease of environmental polarity, the luminogens overcome the detrimental ICT effect and their fluorescence intensities increase along with a blue-shift in the wavelength.
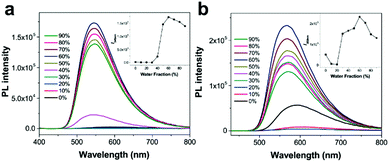 |
| Fig. 3 Fluorescence spectra of TPE-FO (a) and TPE-FO-TPE (b) in different DMSO–water mixture solvents. The insets show the plot of the fluorescence intensity versus water fraction at 546 and 568 nm for TPE-FO (a) and TPE-FO-TPE (b), respectively. The concentration is 10 nM. The excitation wavelength is 360 nm. | |
Water sensing
We have investigated the fluorescence behaviour of TPE-FO and TPE-FO-TPE in THF and dioxane by adding different amounts of water. Typically, Fig. 4 shows the fluorescence responses of TPE-FO and TPE-FO-TPE in THF when the water fraction varies from 0 to 10% (v/v) in THF solutions, respectively. There is a remarkable decrease of fluorescence intensity with the increase of water fraction along with bathochromic shifts from 530 nm to 564 nm and 566 nm to 572 nm, respectively. When the water fraction rises up to 1% (v/v), the fluorescence intensities of TPE-FO (at 530 nm) and TPE-FO-TPE (at 556 nm) in THF are quenched by 90% and 81%, respectively (Fig. S4, ESI†). Especially, the fluorescence quenching percentages of TPE-FO and TPE-FO-TPE in THF reach 97% and 99% respectively when the water fraction reaches 10% (v/v). Similar phenomena were also observed in the dioxane/H2O system but with relatively small fluorescence quenching percentages compared with the THF/H2O system under the same conditions. The Stern–Volmer equation was used to evaluate the sensitivity of dyes, with (I0 − I)/I = KSV[H2O], where KSV was the Stern–Volmer quenching constant.35 When the water fraction is below 0.9% (0.5 M), the Stern–Volmer plots are nearly linear, with a correlation coefficient (R2) of 0.9876, 0.9915, 0.9983 and 0.9985 for TPE-FO/THF, TPE-FO/dioxane, TPE-FO-TPE/THF and TPE-FO-TPE/dioxane systems, respectively (Fig. 5). The KSV values were 17.6012, 12.1983, 8.7652 and 8.8956 M−1 for TPE-FO/THF, TPE-FO/dioxane, TPE-FO-TPE/THF and TPE-FO-TPE/dioxane systems, respectively, indicating ultrasensitivity for water detection. Moreover, the detection limits were estimated based on the 3σ/k method. The calculated detection limits are 22 (0.0020%) and 17 ppm (0.0018%) for TPE-FO-TPE in THF and dioxane, respectively. Markedly, the calculated detection limits are as low as 11 (0.0010%) and 14 ppm (0.0014%) for TPE-FO in THF and dioxane, respectively, which very much approaches the several ppm of modern coulometric Karl–Fischer titration.35 To our knowledge, it is the highest sensitivity of fluorescent sensors for water detection in THF (Table S2, ESI†). What’s more, TPE-FO-TPE also shows considerable sensitivity for water detection in DMF and DMSO with the detection limits of 92 (0.0087%) and 218 ppm (0.0240%), respectively, and it has a wider detection range from 0% to 10% compared to the THF or dioxane system (Fig. S7, ESI†). The extremely low detection limits indicate that these luminogens are ultrasensitive to trace water in organic solvents, which is attributed to solvent effects and intermolecular hydrogen bonding interactions.33 According to the above discussion, the solvent effects are reasonable when considering that TPE-FO and TPE-FO-TPE are sensitive to polarity of the solvent system which changed dramatically when adding an amount of water to organic solvents. On the other hand, intermolecular hydrogen bonding interactions have been reported as important for the fluorescence quenching phenomena of fluorenone derivatives.23,36–38 On the basis of the theoretical computation, the partial atomic charges localized on the oxygen atom of the fluorenone group change from −0.465 to −0.567 and −0.464 to −0.582 for TPE-FO and TPF-FO-TPE, respectively, when excited from the ground state to the first excited state. The large electron cloud density on the oxygen atom in the excited state makes the oxygen atom get considerable electronegativity, which facilitates it to combine with electropositive hydrogen atoms. Therefore, the formation of the intermolecular hydrogen bond C
O⋯H–O can be promoted between the oxygen atom of the fluorenone group and water molecule. The nonradiative deactivation processes of the excited-state can be strongly enhanced by the intermolecular hydrogen bonding interactions.33 As a result, the fluorescence of TPE-FO and TPE-FO-TPE can be efficiently quenched by adding water into organic solvents. Although fluorescent sensors based on solvent effects and intermolecular hydrogen bonding interactions show fluorescence responses to other interferents (Fig. S8, ESI†), they are still promising water sensors with ultrasensitivity in the specific solvent systems containing trace water.
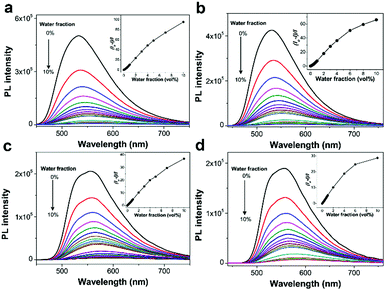 |
| Fig. 4 Fluorescence spectra of TPE-FO and TPE-FO-TPE change with increasing water fraction in THF or dioxane solution (concentration: 10 nM, excitation at 360 nm). (a) TPE-FO/THF, (b) TPE-FO/dioxane, (c) TPE-FO-TPE/THF and (d) TPE-FO-TPE/dioxane. Insets: the Stern–Volmer plot of (I0 − I)/I versus water fractions (0–10%, v/v), where I0 and I represent the peak intensity in the absence and presence of water, respectively. | |
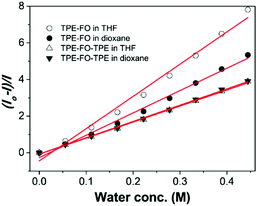 |
| Fig. 5 The Stern–Volmer plot of (I0 − I)/I versus water concentration (M), where I0 and I represent the peak intensity in the absence and presence of water, respectively. The red curves represent the linear fitted curves. | |
In order to demonstrate the practical application of these luminogens as water sensors, test papers of TPE-FO and TPE-FO-TPE were prepared by absorbing a certain amount of the luminogen.22,33 The obtained test papers were immersed in organic solvents with different water fractions. As shown in Fig. 6, the fluorescence emission of test papers decreased significantly and had a red-shift along with the increase of water fraction from 0 to 10% upon illumination of 365 nm UV light. TPE-FO shows more obvious decreases in the fluorescence brightness of both THF and dioxane with increasing water fraction, and the fluorescence difference of the samples between 0% and 1% could be easily picked out by eye. TPE-FO-TPE exhibits bigger changes in emission colors, from green to yellow, with increasing water fraction. Moreover, 10% fraction of water in THF and dioxane can almost completely quench the flourescence of the samples for both TPE-FO and TPE-FO-TPE. These results indicate that TPE-FO and TPE-FO-TPE are promisingly used as a kind of water detectors to detect low level water content in THF or dioxane qualitatively and roughly quantitatively.
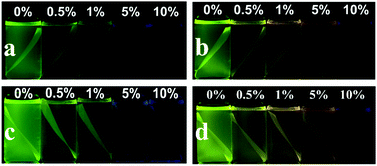 |
| Fig. 6 Fluorescence photographs of test papers having absorbed a certain amount of TPE-FO or TPE-FO-TPE in THF or dioxane solution containing different amounts of water. (a) TPE-FO/THF, (b) TPE-FO-TPE/THF, (c) TPE-FO/dioxane and (d) TPE-FO-TPE/dioxane. | |
Conclusions
In conclusion, using TPE as an electron donor and as an AIE activator, we have designed and synthesized two fluorenone-containing luminogens. The conjugation of the electron-donating TPE and the electron-accepting fluorenone group enables ICT processes in the molecules of TPE-FO and TPE-FO-TPE, which exhibit pronounced solvatochromic effects and AIE activity. Additionally, these two luminogens exhibit high fluorescence responses to trace water in THF or dioxane and the Stern–Volmer plots show good linear relationships with water fractions below 0.9%. The detection limits were estimated to be as low as 11 ppm, indicating an ultrasensitive water determination ability. These luminogens are expected to make ultrasensitive water detectors for organic solvents.
Acknowledgements
This work was supported by NSFC (51673077, 21474034, 51603078), the National Basic Research Program of China (Grant No. 2013CB922104, 2015CB755602), the Fundamental Research Funds for the Central Universities (HUST: 2016YXMS029), and Director Fund of WNLO. We also thank the Analytical and Testing Center of Huazhong University of Science and Technology, the Center of Micro-Fabrication and Characterization (CMFC) and the Center for Nanoscale Characterization & Devices (CNCD) of WNLO for use of their facilities.
Notes and references
- J. Luo, Z. Xie, J. W. Y. Lam, L. Cheng, H. Chen, C. Qiu, H. S. Kwok, X. Zhan, Y. Liu, D. Zhu and B. Z. Tang, Chem. Commun., 2001, 1740 RSC.
- J. Mei, N. L. Leung, R. T. Kwok, J. W. Lam and B. Z. Tang, Chem. Rev., 2015, 115, 11718–11940 CrossRef CAS PubMed.
- N. Zhao, Z. Yang, J. W. Y. Lam, H. H. Y. Sung, N. Xie, S. Chen, H. Su, M. Gao, I. D. Williams, K. S. Wong and B. Z. Tang, Chem. Commun., 2012, 48, 8637–8639 RSC.
- A. Rananaware, D. Duc La and S. V. Bhosale, RSC Adv., 2015, 5, 56270–56273 RSC.
- A. Rananaware, A. N. Abraham, D. D. La, V. Mistry, R. Shukla and S. V. Bhosale, Aust. J. Chem, 2016 DOI:10.1071/CH16459.
- W. Z. Yuan, F. Mahtab, Y. Gong, Z.-Q. Yu, P. Lu, Y. Tang, J. W. Y. Lam, C. Zhu and B. Z. Tang, J. Mater. Chem., 2012, 22, 10472–10479 RSC.
- Anuradha, D. D. La, M. Al Kobaisi and S. V. Bhosale, Sci. Rep., 2015, 5, 15652 CrossRef CAS PubMed.
- A. Rananaware, R. S. Bhosale, K. Ohkubo, H. Patil, L. A. Jones, S. L. Jackson, S. Fukuzumi, S. V. Bhosale and S. V. Bhosale, J. Org. Chem., 2015, 80, 3832–3840 CrossRef CAS PubMed.
- A. Rananaware, D. Duc La and S. V. Bhosale, RSC Adv., 2015, 5, 56270–56273 RSC.
- H. Li, X. Zheng, H. Su, J. W. Y. Lam, K. Sing Wong, S. Xue, X. Huang, X. Huang, B. S. Li and B. Z. Tang, Sci. Rep., 2016, 6, 19277 CrossRef PubMed.
- M. Salimimarand, D. D. La, M. A. Kobaisi and S. V. Bhosale, Sci. Rep., 2017, 7, 42898 CrossRef CAS PubMed.
- G. F. Zhang, M. P. Aldred, W. L. Gong, C. Li and M. Q. Zhu, Chem. Commun., 2012, 48, 7711–7713 RSC.
- M. P. Aldred, G. F. Zhang, C. Li, G. Chen, T. Chen and M. Q. Zhu, J. Mater. Chem. C, 2013, 1, 6709–6718 RSC.
- N. H. Xie, C. Li, J. X. Liu, W. L. Gong, B. Z. Tang, G. Li and M. Q. Zhu, Chem. Commun., 2016, 52, 5808–5811 RSC.
- R. Hu, C. F. A. Gomez-Duran, J. W. Y. Lam, J. L. Belmonte-Vazquez, C. Deng, S. Chen, R. Ye, E. Pena-Cabrera, Y. Zhong, K. S. Wong and B. Z. Tang, Chem. Commun., 2012, 48, 10099–10101 RSC.
- X. Y. Shen, Y. J. Wang, E. Zhao, W. Z. Yuan, Y. Liu, P. Lu, A. Qin, Y. Ma, J. Z. Sun and B. Z. Tang, J. Phys. Chem. C, 2013, 117, 7334–7347 CAS.
- Y. Li, Z. Li, Y. Wang, A. Compaan, T. Ren and W.-J. Dong, Energy Environ. Sci., 2013, 6, 2907–2911 CAS.
- Q. Qi, J. Qian, X. Tan, J. Zhang, L. Wang, B. Xu, B. Zou and W. Tian, Adv. Funct. Mater., 2015, 25, 4005–4010 CrossRef CAS.
- A. Rananaware, D. D. La and S. V. Bhosale, RSC Adv., 2015, 5, 63130–63134 RSC.
- H.-R. Xu, K. Li, S.-Y. Jiao, S.-L. Pan, J.-R. Zeng and X.-Q. Yu, Analyst, 2015, 140, 4182–4188 RSC.
- H. Shi, R. Liu, S. Zhu, Q. Gong, H. Shi, X. Zhu and H. Zhu, J. Fluoresc., 2016, 26, 2005–2013 CrossRef CAS PubMed.
- Y. Zhang, D. Li, Y. Li and J. Yu, Chem. Sci., 2014, 5, 2710 RSC.
- G. J. Zhao and K. L. Han, J. Phys. Chem. A, 2009, 113, 14329–14335 CrossRef CAS PubMed.
- Y. Liu, X. Tao, F. Wang, J. Shi, J. Sun, W. Yu, Y. Ren, D. Zou and M. Jiang, J. Phys. Chem. C, 2007, 111, 6544–6549 CAS.
- M. S. Yuan, D. E. Wang, P. Xue, W. Wang, J. C. Wang, Q. Tu, Z. Liu, Y. Liu, Y. Zhang and J. Wang, Chem. Mater., 2014, 26, 2467–2477 CrossRef CAS.
- M. S. Yuan, X. Du, F. Xu, D.-E. Wang, W. J. Wang, T. B. Li, Q. Tu, Y. Zhang, Z. Du and J. Wang, Dyes Pigm., 2015, 123, 355–362 CrossRef CAS.
- F. Xu, H. Wang, X. Du, W. Wang, D.-E. Wang, S. Chen, X. Han, N. Li, M. S. Yuan and J. Wang, Dyes Pigm., 2016, 129, 121–128 CrossRef CAS.
- G. Men, G. Zhang, C. Liang, H. Liu, B. Yang, Y. Pan, Z. Wang and S. Jiang, Analyst, 2013, 138, 2847–2857 RSC.
- H. S. Jung, P. Verwilst, W. Y. Kim and J. S. Kim, Chem. Soc. Rev., 2016, 45, 1242–1256 RSC.
- R. Nussbaum, D. Lischke, H. Paxmann and B. Wolf, Chromatographia, 2000, 51, 119 CAS.
- K. Fischer, Angew. Chem., 1935, 48, 394 CrossRef CAS.
- S. Pal, M. Mukherjee, B. Sen, S. Lohar and P. Chattopadhyay, RSC Adv., 2014, 4, 21608 RSC.
- L. Ding, Z. Zhang, X. Li and J. Su, Chem. Commun., 2013, 49, 7319–7321 RSC.
- Y. Hong, J. W. Y. Lam and B. Z. Tang, Chem. Commun., 2009, 4332 RSC.
- D. Citterio, K. Minamihashi, Y. Kuniyoshi, H. Hisamoto, S. i. Sasaki and K. Suzuki, Anal. Chem., 2001, 73, 5339 CrossRef CAS PubMed.
- A. Morimoito, T. Yatsuhashi, T. Shimada, L. Biczók, D. A. Tryk and H. Inoue, J. Phys. Chem. A, 2001, 105, 10488–10496 CrossRef CAS.
- Y. Matsushita, T. Ichimura and T. Hikida, Chem. Phys. Lett., 2002, 360, 65–71 CrossRef CAS.
- Y. H. Liu, G. J. Zhao, G. Y. Li and K. L. Han, J. Photochem. Photobiol., A, 2010, 209, 181–185 CrossRef CAS.
Footnote |
† Electronic supplementary information (ESI) available: Characterization, optical spectra, and SEM images, NMR data and relevant data for TPE-FO and TPE-FO-TPE. See DOI: 10.1039/c7qm00172j |
|
This journal is © the Partner Organisations 2017 |
Click here to see how this site uses Cookies. View our privacy policy here.