DOI:
10.1039/C6RA06303A
(Paper)
RSC Adv., 2016,
6, 57828-57834
Lanthanide metal–organic frameworks based on a 1,2,3-triazole-containing tricarboxylic acid ligand for luminescence sensing of metal ions and nitroaromatic compounds†
Received
9th March 2016
, Accepted 6th June 2016
First published on 8th June 2016
Abstract
A series of novel 3D lanthanide metal–organic frameworks based on unsymmetrical 1,2,3-triazole-containing tricarboxylic acid ligand 5-[4-(4-carboxy-phenyl)-[1,2,3]triazol-1-yl]-isophthalic (H3TAIP) has been synthesized under solvothermal conditions. The ligand was synthesized with diethyl-5-azidoisophthalate and 4-ethynyl-benzoic acid ethyl ester through the copper-catalyzed azide alkyne cycloaddition (CuAAC) reaction. Single-crystal X-ray diffraction analyses reveal that these metal–organic frameworks are isostructural. The structures are constructed by bi-nuclear lanthanide metal clusters and TAIP3− ligands and possess flu-3,6-C2/c net with point symbol (42.6)2(44.62.87.102). We explore the luminescence properties of compound 1, which shows the characteristic emission of Tb3+ and exhibits selective detection of 4-nitrophenol and Fe3+ ions in ethanol solution over other nitroaromatic compounds and metal ions.
Introduction
Metal–organic frameworks (MOFs) as a fantastic type of crystalline solid porous materials have attracted much attention in the past two decades due to their interesting architectures and their potential for a wide range of applications in gas storage, separation, heterogeneous catalysis, magnetism, drug delivery etc.1 Since the high surface areas, tailored pore size, structural flexibility and functionality of MOFs, it has became suitable candidates of chemical sensors.2 Rapid detection of high explosives, heavy metal ions and some hazardous chemicals is important for homeland security, civilian safety, and environmental protection.3 But the current detection methods of nitrocompounds and metal ions are limited by their equipment-demanding which are expensive and not easily accessible. Recently, a simple, sensitive and convenient method of fluorescence quenching based detection of metal ions and electron-deficient nitroaromatics using luminescent MOFs has attracted considerable interest.4 The delocalized π-electrons of the aromatic ligands could increase the electrostatic interaction between the framework and the electron deficient nitro-aromatics. Further, the photoinduced electron transfer from an excited state of ligands to the guest molecules results in fluorescence quenching of the luminescent MOF.5 On the other hand, the detection of metal ions also plays an important role in biological and environmental systems.6 Compared to other detection methods, fluorescence quenching based detection is highly promising due to its simplicity and high sensitivity.
Compared with the metal–organic frameworks based on transition metal ions, lanthanide metal–organic frameworks (Ln-MOFs) have been particularly valued on account of the fact that they possess unique optical properties,7 such as large Stokes' shifts, high color purity, and relatively long luminescence lifetimes, which originated from f–f transitions via an “antenna effect”.8 The pioneering work of Li et al., Ghosh et al., Liu et al., Chen et al. and others has focused on various Ln-MOFs materials for luminescent sensing and demonstrated that Ln-MOFs exhibit excellent luminescence properties and the potential value in explosives and metal ions detection.9 So we can foresee that Ln-MOFs are excellent candidate for the development of novel kinds of chemical sensors.
Certainly, the organic ligands play crucial roles in the control of the structures and functions of MOFs. One of the most important reasons is that by simple selection of different kinds of ligands, the chemical and physical properties of MOFs can be adjusted. Such an idea inspires chemists to design novel ligands in the construction of diverse MOFs. Embedding Lewis basic sites such as –NH2, –OH, pyridyl and polyazole groups into the organic ligands to construct MOFs can largely improve their ability to recognize and sense neutral and ionic species.9c,10 Also, it is supposed that unsymmetrical ligands with two or more distinct carboxylate linkers give facilities for constructing MOFs with novel topologies and interesting properties.11 In our previous work, great efforts have been paid in the design of novel rigid ligands, such as 5-((4-carboxyphenyl)ethynyl)isophthalic acid (H3CPEIP), p-terphenyl-3,4′′,5-tricarboxylic acid (H3TPT), 5,5′-(1H-1,2,3-triazole-1,4-diyl)diisophthalic acid (H4TADIP) etc.12 With these ligands we synthesized various of new luminescent MOFs and exhibits high efficiency detection for nitroaromatic compounds and metal ions by fluorescence quenching mechanism.12b,c,13 To continue our efforts in luminescence sensing, in this work, a novel 5-[4-(4-carboxy-phenyl)-[1,2,3]triazol-1-yl]-isophthalic acid (H3TAIP) ligand has been designed and synthesized to build Ln-MOFs. Three new 3D Ln-MOFs based on this ligand have been successfully synthesized under solvothermal conditions. In addition, we explored its application in fluorescence sensing of nitroaromatic compounds and metal ions.
Experimental section
Materials and physical characterizations
Except for the H3TAIP ligand, all other chemicals were purchased commercially and used without further purification. The IR absorption spectra were recorded on a Nicolet Impact 410 FTIR spectrometer with KBr pellets in the range of 400–4000 cm−1. Powder X-ray diffraction (PXRD) patterns were collected on a Rigaku D-Max 2550 diffractometer using Cu-Kα radiation (λ = 0.15418 nm) in a 2θ range of 4–40° with a scan speed of 12° min−1 at room temperature. The elemental analyses (C, H and N) were performed using a Perkin-Elmer 2400 elemental analyzer. The thermogravimetric analyses (TGA) data were recorded on Perkin-Elmer TGA Q500 thermogravimetric analyzer from room temperature to 800 °C with a heating rate of 10 °C min−1 under a N2 atmosphere. All fluorescence measurements were carried out on a SHIMADZU RF-5301 PC fluorescence spectrophotometer. The point symbol and topological analysis were conducted using the TOPOS program package.14
Crystal structure determination
Single-crystal X-ray diffraction measurement was performed on a Bruker AXS SMART APEX-II diffractometer with graphite monochromated Mo Kα (λ = 0.71073 Å) radiation at 293 K. Data processing was completed with the SAINT processing program.15 The crystal structures were solved by means of the direct method and refined employing full-matrix least squares on F2 (SHELX-97 program).16 All non-hydrogen atoms were refined with anisotropic displacement parameters. Some restraints, like delu, isor, dfix were added to the terminal coordinated DMF molecules for a better geometry configuration. The hydrogen atoms on the aromatic rings were geometrically placed with isotropic thermal parameters 1.2 times that of the attached carbon atom. The SQUEEZE routine of PLATON was used to remove the diffraction contribution from guests to produce a set of solvent-free diffraction intensities.17 A summary of the crystallographic data and refinement parameters are given in Tables S1, S3 and S5.† The selected bond lengths and angles are given in Tables S2, S4 and S6.†
Synthesis of M1 and M2
Diethyl-5-azidoisophthalate (M1) was synthesized according to the literature procedure18 and used immediately without further purification. 4-Ethynyl-benzoic acid ethyl ester (M2) was synthesized according to the literature procedures.12a,19
Synthesis of 5-[4-(4-ethoxycarbonyl-phenyl)-[1,2,3]triazol-1-yl]-isophthalic acid diethyl ester (M3)
To a solution of M1 (2.630 g, 10.0 mmol) and M2 (1.828 g, 10.5 mmol) in tert-BuOH–H2O (1
:
1, 100 mL) were added sodium ascorbate (180 mg, 0.91 mmol) and CuSO4·5H2O (60 mg, 0.24 mmol). The mixture was stirred at ambient temperature overnight and then a precipitate was formed. The precipitate was filtered off and dissolved in ethyl acetate, washed with aqueous NH3 (5%) and brine for several times, and dried over MgSO4. Evaporation and recrystallization from ethyl acetate gave the product as a yellow solid. CHN elemental analysis (%) for M3, C23H23N3O6: C, 63.15; H, 5.30; N, 9.61. Found: C, 63.37; H, 5.49; N, 9.61. 1H NMR (300 MHz, CDCl3): δ = 8.76 (t, J = 1.5 Hz, 1H), 8.66 (d, J = 1.5 Hz, 2H), 8.43 (s, 1H), 8.15 (d, J = 8.4 Hz, 2H), 8.01 (d, J = 8.4 Hz, 2H), 4.48 (q, J = 7.8 Hz, 4H), 4.41 (q, J = 7.2 Hz, 2H), 1.46 (t, J = 6.9 Hz, 6H), 1.43 (t, J = 7.2 Hz, 3H). 13C NMR (75 MHz, CDCl3): δ = 166.0, 164.4, 147.9, 137.2, 133.9, 132.9, 130.5, 130.4, 120.2, 125.6, 124.9, 118.3, 61.9, 61.0, 14.22, 14.20. MS (ESI): 438.168 (M + H+). IR (cm−1): 2991, 1722, 1695, 1455, 1244, 1014.
Synthesis of 5-[4-(4-carboxy-phenyl)-[1,2,3]triazol-1-yl]-isophthalic acid (H3TAIP)
To a solution of M3 (4.809 g, 11 mmol) in H2O–THF (1
:
1, 120 mL) was added KOH (8.081 g, 144 mmol). The mixture was refluxed for about 12 h and THF was evaporated. The resulting solution was acidified to pH 4–5 with HCl (2 M). The precipitate was filtered and washed with water successively to give H3TAIP as a yellow solid (3.944 g, 9.93 mmol, 91.26% yield). CHN elemental analysis (%) for H3TAIP, C17H11N3O6: C, 57.80; H, 3.14; N, 11.89. Found: C, 56.92; H, 3.14; N, 11.15. 1H NMR (300 MHz, DMSO-d6): δ = 13.50 (bs, 2H), 9.77 (s, 1H), 8.73 (d, J = 1.5 Hz, 2H), 8.54 (t, J = 1.5 Hz, 1H), 8.15–8.05 (m, 4H). 13C NMR (75 MHz, DMSO-d6): δ = 166.8, 165.5, 146.6, 136.9, 134.0, 133.1, 130.3, 130.0, 129.4, 125.2, 123.8, 121.0. MS (ESI): 354.074 (M + H+). IR (cm−1): 3068, 1722, 1684, 1614, 1425, 1278, 1172.
Synthesis of [Tb(TAIP)(DMF)2] (1)
Tb(NO3)3·6H2O (25 mg, 0.0552 mmol) and H3TAIP (10 mg, 0.0282 mmol) were dissolved in DMF (1 mL), and then HNO3 (125 μL, 2.7 M in DMF) was added. The solution was sealed in a 20 mL vial and heated at 85 °C for 36 hours, 105 °C for 24 hours, then cooled to room temperature. Colourless block-shaped crystals were collected by filtration with a 74% yield based on H3TAIP. CHN elemental analysis (%) for 1, C23H22TbN5O8: C, 42.15; H, 3.38; N, 10.69. Found: C, 41.09; H, 3.77; N, 11.75. IR (cm−1): 3087(w), 2932(w), 1668(s), 1591(m), 1531(m), 1384(s), 1238(w), 1101(w), 1050(w), 852(m), 782(s), 706(s), 671(m), 508(w), 430(w).
Synthesis of [La(TAIP)(DMF)2]·(DMF)0.5 (2)
La(NO3)3·6H2O (25 mg, 0.0577 mmol) and H3TAIP (10 mg, 0.0282 mmol) were dissolved in DMF (1 mL), and then HNO3 (125 μL, 2.7 M in DMF) was added. The solution was sealed in a 20 mL vial and heated at 85 °C for 36 hours, 105 °C for 24 hours, then cooled to room temperature. Colourless block-shaped crystals were collected by filtration with a 67% yield based on H3TAIP. CHN elemental analysis (%) for 2, C49H51La2N11O17: C, 43.80; H, 3.83; N, 11.47. Found: C, 42.02; H, 4.20; N, 11.33. IR (cm−1): 1634(m), 1582(m), 1552(w), 1402(s), 1367(s), 1050(w), 852(w), 782(s), 749(m), 714(s), 422(w).
Synthesis of [Eu(TAIP)(DMF)2] (3)
EuCl3·6H2O (25 mg, 0.0682 mmol) and H3TAIP (10 mg, 0.0282 mmol) were dissolved in DMF (1 mL), and then HNO3 (125 μL, 2.7 M in DMF) was added. The solution was sealed in a 20 mL vial and heated at 85 °C for 36 hours, 105 °C for 24 hours, then cooled to room temperature. Colourless block-shaped crystals were collected by filtration with a 86% yield based on H3TAIP. CHN elemental analysis (%) for 3, C23H22EuN5O8: C, 42.60; H, 3.42; N, 10.80. Found: C, 40.77; H, 4.06; N, 11.18. IR (cm−1): 3087(w), 1651(s), 1582(s), 1539(m), 1384(s), 1247(w), 1101(m), 1050(m), 852(m), 782(s), 714(s), 671(m), 525(w), 430(w).
Synthesis of micrometer-sized phase 1′
Tb(NO3)3·6H2O (1250 mg, 2.76 mmol) and H3TAIP (500 mg, 1.41 mmol) were dissolved in a mixture of DMF (50 mL), and then HNO3 (6.25 mL, 2.7 M in DMF) was added. The mixture was sealed in a 100 mL flask and heated at 100 °C with stirring on an oil bath for 3 days. The powder product of micrometer-sized phase 1′ was obtained from the mother liquor by centrifugation and dried in air at room temperature. The product was obtained in 63% yield based on H3TAIP.
Micrometer-sized phase 2′ and 3′ were synthesized under similar reaction conditions using La(NO3)3·6H2O and EuCl3·6H2O, respectively.
Detection experiments for metal ions and nitroaromatic compounds
0.5 mg of as-synthesized micrometer-size phase 1′ above mentioned were well dispersed in a solution of 2.0 mL ethanol with stirring for about 10 minutes, then various amounts of analytes ([analytes] = 1 × 10−3 M) were added to a quartz cuvette containing 2 mL ethanol suspension of micrometer-size phase for luminescent detection experiment.
Results and discussion
Synthesis of ligand and MOFs
As depicted in Scheme 1, the ligand of H3TAIP was synthesized by the copper-catalyzed azide–alkyne cycloaddition (CuAAC) reaction of diethyl-5-azidoisophthalate and 4-ethynyl-benzoic acid ethyl ester followed by hydrolysis. Based on the ligand, three new MOFs were synthesized under solvothermal conditions. The ligand and lanthanide salts were dissolved in DMF, and then a certain amount of HNO3 was added. Colourless block-shaped crystals were collected after heated at 85 °C for 36 hours, and then 105 °C for 24 hours.
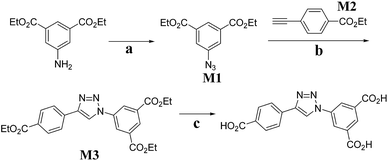 |
| Scheme 1 Reaction conditions: (a) NaNO2, HCl (6 M), NaN3, 0–5 °C; (b) CuSO4·5H2O, Na-ascorbate, H2O–tert-BuOH, room temperature; (c) H2O–THF, KOH, 80 °C, overnight. | |
Crystal structure descriptions
Single-crystal X-ray analyses reveal that compounds 1–3 are isostructural. The structure of compound 1 is described here representatively. Compound 1 crystallizes in the monoclinic space group C2/c. As shown in Fig. S1,† the asymmetric unit contains one crystallographically independent nine-coordinated Tb(III) ion, one TAIP ligand, two coordinated DMF molecules. Each Tb(III) center is coordinated to seven oxygen atoms (O1–O8, O7A) from five TAIP linkers, two oxygen atoms (O4, O5) from two terminal DMF molecules. The longest Tb–O bond in compound 1 is Tb(1)–O(7) with bond length of 2.767(4) Å. The remaining Tb–O bond distances ranging from 2.340(4) to 2.481(5) Å are close to the previously reported Tb–O lengths. The carboxylate groups TAIP ligand display three distinct coordination modes: bridging, chelating and chelate-bridging coordination (Fig. 1a). Four carboxylate groups from isophthalic moieties connect two Tb ions at equatorial positions, and other two carboxylate groups from benzoic acid occupy the axial position to link the same two Tb ions, forming a distorted [Tb2(COO)6] cluster (Fig. 1b). In the structure the three-connected TAIP ligands are linked together through six-connected terbium clusters to construct a 3D neutral framework (Fig. 1c).
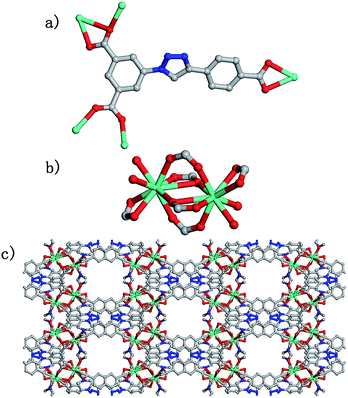 |
| Fig. 1 (a) Coordination modes of carboxyl groups of TAIP ligand. (b) The distorted binuclear [Tb2(COO)6] motif. (c) The 3D framework of 1 showing the channels along [001] direction. The coordinated DMF molecules are simplified to be coordinated oxygen are omitted for clarity (colour mode: Tb green, N blue, O red, C gray). | |
Topological approach can help us to better understand the 3D framework of 1. In compound 1, the ligand can be considered as an organic three-connected node (Fig. 2a) and each binuclear metal center is regarded as a six-connected node (Fig. 2b). The cross link of these two kinds of nodes results in the 3D (3,6)-connected framework (Fig. 2c). Topological analysis reveals that it possesses a flu-3,6-C2/c net with point symbol (42.6)2(44.62.87.102). Such a topological feature has been reported by other unsymmetrical tricarboxylate ligands, such as p-terphenyl-3,4′′,5-tricarboxylate acid, biphenyl-3,4′,5-tricarboxylate, 5-((4-carboxyphenyl)ethynyl)isophthalic acid and 3,4′,5-azobenzenetricarboxylic acid.12a,13a,20
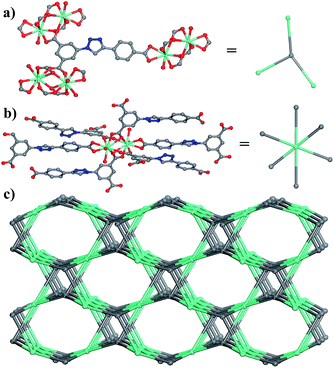 |
| Fig. 2 (a and b) Ball-and-stick and schematic representations of 3-connected (gray) and 6-connected nodes (green), respectively (the coordinated DMF molecules are simplified to be coordinated oxygen for clarity). (c) Schematic representation of 3,6-connected flu-3,6-C2/c topology of 1. | |
Powder XRD patterns, infrared spectra and thermal properties
The X-ray powder diffraction (PXRD) experiments of the three compounds have been performed to check the phase purity. As shown in Fig. S2 (ESI†), the peak positions of the patterns are mainly consistent, proving the high phase purity of the synthesized products. Infrared spectroscopy was used to characterize the functional group. As shown in Fig. S3 (ESI†), the three compounds have almost the same characteristic vibrations, further confirming that these compounds are isostructural. Thermogravimetric analyses (TGA) was used to characterize the thermal stability of the compounds. As shown in Fig. S4 (ESI†), the curves of the three compounds are similar. Compound 1 exhibits a total weight loss of 74.98% (calcd: 75.74%), which corresponds to the loss of DMF molecules and decomposition of organic ligand. Similarly, compounds 2 and 3 exhibit a total weight loss of 77.09% (calcd: 79.32%) and 75.93% (calcd: 76.44%), respectively.
Luminescent property and detection for metal ions
We investigated the luminescent properties of the three compounds in ethanol suspensions. The results show that compounds 1, 2 and 3 exhibit luminescent properties (Fig. S6, ESI†), but the intensities of compounds 2 and 3 are much lower than that of compound 1 (Fig. S7, ESI†). The outstanding luminescent property of 1 make us interest in its potential application in detection of common metal ions. In order to study the selectivity of sensing ability for various metal ions, the as-synthesized micrometer-size phase 1′ was immersed in ethanol solution, with stirring for about 10 minutes, then various amounts of different nitrate salts M(NO3)x (M = Fe3+, Pb2+, Cd2+, Cr3+, Zn2+, Sr2+, Cu2+, In3+, Ni2+, Co2+, Ba2+, Li+ and Mg2+) as well as FeCl2 were added for the sensing studies, and the concentration of the metal ions in ethanol is 95.02 μM. Interesting, we found that different metal ions exhibit different degrees of fluorescence enhancement or quenching when the concentration of metal ions added to 95.02 μM. The luminescent quench percentage (QP) was calculated with the formula of QP = [(Io − I)/Io] × 100%, where I0 and I are the luminescent intensities of 1′–ethanol suspensions before and after the addition of analytes, respectively. As shown in Fig. 3, the quenching efficiency in the following orders: Fe3+ > Fe2+ > Cr3+ > Cu2+ > Ba2+ > In3+ > Li+ > Pb2+ > Co2+ > Zn2+ > Ni2+ > Cd2+ > Mg2+ > Sr2+. These results indicate that about 46% quenching could be observed of Fe3+, which is significantly more than that of Fe2+ (17%), Cr3+ (10%) and Cu (10%).
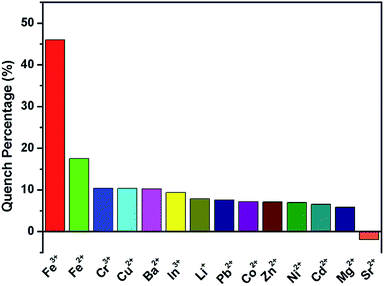 |
| Fig. 3 Reduction of luminescent intensity (plotted as quench percentage) of 1′–ethanol suspension in presence of different metal ions with the concentration of 95.02 μM (λex = 305 nm). | |
To further examine the sensing ability of 1′ for Fe3+ ions, another luminescent titration experiment has done with gradually increased Fe3+ ions concentration. As shown in Fig. 4a, the luminescent intensity of 1′ decreases gradually with the addition of Fe3+ ions, the quench percentage was about 87% when the Fe3+ concentration up to 333.33 μM. Within low concentrations, the quenching effect can be rationalized by the Stern–Volmer equation, Io/I = 1 + Ksv × [M], where Io and I are the luminescent intensities of 1′–ethanol suspensions before and after the addition of analytes, respectively; [M] is the molar concentration of analytes; Ksv is the quenching constant and was calculated by the experimental data fitting from Fe3+ ions concentration. As shown in Fig. 4b, the Stern–Volmer plot is almost linear for Fe3+ at the low concentrations of 0–70 μM. The Ksv value is 8.86 × 103 M−1, which is comparable those of reported MOF-based sensors.21
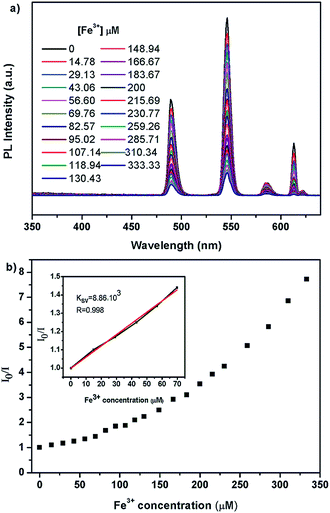 |
| Fig. 4 (a) Luminescent quenching of 1′ dispersed in ethanol by gradual addition of Fe3+–ethanol solution ([Fe3+] = 1 × 10−3 M). (b) Stern–Volmer plot of Io/I versus Fe3+ concentration in 1–ethanol suspension (insert: the selected area enlarged view). | |
In order to explore the underlying mechanism of the luminescence quenching, the X-ray powder diffraction experiments of micrometer-sized phase 1′ after test have been performed (Fig. S11†), the result shows that the crystal structures remain unchanged. In addition, the UV-Vis absorption spectra for M(NO3)x in ethanol solutions have been recorded. As shown in Fig. S12,† the absorption spectrum of Fe3+ in ethanol solution has a large overlap with the excitation spectrum of micrometer-sized phase 1′. We speculated that the luminescence quenching might be due to the competitive absorption of the light source energy and the electronic interaction between Fe3+ and TAIP moieties. The Fe3+ filter the light adsorbed by TAIP moieties, and then decrease subsequent energy transfer from TAIP to Tb3+, which causes the quenching phenomenon.22
Detection for nitroaromatic compounds
In addition, we studied the sensing ability of 1′ to various kinds of nitroaromatic compounds in the same method, such as 2,4,6-trinitrotoluene (TNT), 2,4-dinitrotoluene (2,4-DNT), 4-nitrotoluene (4-NT), 4-nitrobenzaldehyde (4-NBA), 4-chloronitrobenzene (4-Cl-NB), 2,4-dinitrophenol (2,4-DNP), 4-nitrophenol (4-NP) and picric acid (PA). As shown in Fig. 5, the addition of 56.60 μM of analytes gives rise to different degrees of fluorescent quenching, and in the following order: 4-NP > 2,4-DNP > PA > 4-Cl-NB > 4-NT > TNT > 2,4-DNT > 4-NBA. These results indicate that about 65% quenching could be observed of 4-NP, which is significantly more than other nitroaromatic compounds.
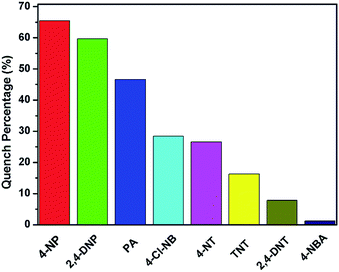 |
| Fig. 5 QP of luminescence of 1′–ethanol suspension in present of different nitroaromatic compounds with the concentration of 56.60 μM (λex = 305 nm). | |
The sensing ability of 1′ for 4-NP was also investigated in the same way through the gradual addition of 4-NP–ethanol solution to 1′–ethanol suspension. As shown in Fig. 6a, the luminescent intensity of 1′–ethanol decreases clearly with the addition of 4-NP. When the concentration of 4-NP in 1′–ethanol suspension reaches to 130.43 μM, the fluorescence is almost completely quenched with the quench percentage of 91%. In the same way, the quenching effect can be rationalized by the Stern–Volmer equation in the low concentration. As shown in Fig. 6b, the Stern–Volmer is almost linear for 4-NP at the low concentrations of 0–60 μM, indicating that the quenching mechanism can be explained by the combination of dynamic and static mechanisms. The static quenching mechanism may attribute to the hydrogen-bonding interactions between the hydroxyl groups of 4-NP and the carboxylate groups of TAIP ligand. And the dynamic quenching mechanism may attribute to the collisions encounters between 4-NP and the fluorene moieties. The quenching constant (Ksv) of 1′ for 4-NP at low concentration is found to be 3.35 × 104 M−1, which is comparable those of reported MOF-based sensors.23
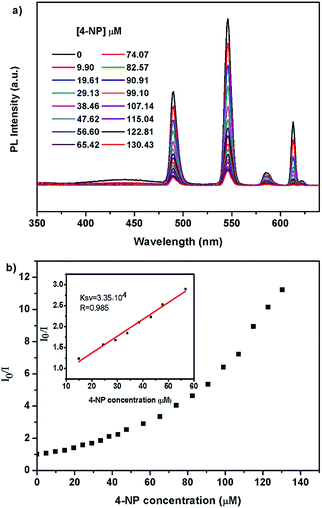 |
| Fig. 6 (a) Luminescent quenching of 1′ dispersed in ethanol by gradual addition of 4-NP–ethanol solution ([4-NP] = 1 × 10−3 M). (b) Stern–Volmer plot of Io/I versus the concentration of 4-NP in 1′–ethanol suspension (insert: the selected area enlarged view). | |
Conclusions
In conclusion, we have successfully synthesized three novel MOFs based on an unsymmetrical 1,2,3-triazole-containing tricarboxylic acid ligand H3TAIP with lanthanide salts under solvothermal conditions. Single-crystal X-ray diffraction analyses reveal that these metal–organic frameworks are isostructural and crystallize in the monoclinic space group C2/c. Moreover, we study the luminescent property of compound 1 (Tb-MOF) and its detection for metal ions and nitroaromatic compounds. The result indicate that both metal ions and nitroaromatic compounds can quench fluorescence in different degrees. This phenomenon can be attributed to the energy transfer effect between the skeleton of MOF to metal ions and nitroaromatic explosives. This work may provide helpful information to construct MOFs based on unsymmetrical ligand for luminescence sensing of metal ions and nitroaromatic compounds. The H3TAIP ligand can potentially be used in the construction of other metal-based MOFs, and this work is currently being performed in our laboratory.
Acknowledgements
We thank the National Natural Science Foundation of China (Grants: 21320102001 and 21471064) for support to this work.
Notes and references
-
(a) J. A. Mason, M. Veenstra and J. R. Long, Chem. Sci., 2014, 5, 32 RSC;
(b) H. H. Wu, Q. H. Gong, D. H. Olson and J. Li, Chem. Rev., 2012, 112, 836 CrossRef CAS PubMed;
(c) J.-R. Li, R. J. Kuppler and H.-C. Zhou, Chem. Soc. Rev., 2009, 38, 1477 RSC;
(d) S. S. Han, J. L. Mendoza-Cortes and W. A. Goddard III, Chem. Soc. Rev., 2009, 38, 1460 RSC;
(e) M. Kurmoo, Chem. Soc. Rev., 2009, 38, 1353 RSC;
(f) N. A. Vermeulen, O. Karagiaridi, A. A. Sarjeant, C. L. Stern, J. T. Hupp, O. K. Farha and J. F. Stoddart, J. Am. Chem. Soc., 2013, 135, 14916 CrossRef CAS PubMed;
(g) J. An, S. J. Geib and N. L. Rosi, J. Am. Chem. Soc., 2009, 131, 8376 CrossRef CAS PubMed;
(h) P. Horcajada, T. Chalati, C. Serre, B. Gillet, C. Sebrie, T. Baati, J. F. Eubank, D. Heurtaux, P. Clayette, C. Kreuz, J.-S. Chang, Y. K. Hwang, V. Marsaud, P.-N. Bories, L. Cynober, S. Gil, G. Ferey, P. Couvreur and R. Gref, Nat. Mater., 2010, 9, 172 CrossRef CAS PubMed;
(i) J. Lee, O. K. Farha, J. Roberts, K. A. Scheidt, S. T. Nguyen and J. T. Hupp, Chem. Soc. Rev., 2009, 38, 1450 RSC.
-
(a) L. E. Kreno, K. Leong, O. K. Farha, M. Allendorf, R. P. Van Duyne and J. T. Hupp, Chem. Rev., 2012, 112, 1105 CrossRef CAS PubMed;
(b) M. D. Allendorf, C. A. Bauer, R. K. Bhakta and R. J. T. Houk, Chem. Soc. Rev., 2009, 38, 1330 RSC;
(c) Y. Cui, Y. Yue, G. Qian and B. Chen, Chem. Rev., 2012, 112, 1126 CrossRef CAS PubMed;
(d) D. Banerjee, Z. C. Hu and J. Li, Dalton Trans., 2014, 43, 10668 RSC;
(e) Z. C. Hu, B. J. Deibert and J. Li, Chem. Soc. Rev., 2014, 43, 5815 RSC;
(f) X. S. Lian, D. Zhao, J. Y. Cui, Y. Yang and G. D. Qian, Chem. Commun., 2015, 51, 17676 RSC;
(g) Y. Yang, F. L. Jiang, L. Chen, J. D. Pang, M. Y. Wu, X. Y. Wan, J. Pan, J. J. Qian and M. C. Hong, J. Mater. Chem. A, 2015, 3, 13526 RSC;
(h) D. Zhao, Y. J. Cui, Y. Yang and G. D. Qian, CrystEngComm, 2016, 18, 3746 RSC.
- Y. Salina, R. Martinez-Manez, M. D. Marcos, F. Sancenon, A. M. Castero, M. Parra and S. Gill, Chem. Soc. Rev., 2012, 41, 1261 RSC.
-
(a) Y.-W. Li, J.-R. Li, L.-F. Wang, B.-Y. Zhou, Q. Chen and X.-H. Bu, J. Mater. Chem. A, 2013, 1, 495 RSC;
(b) X. Zhou, H. Li, H. Xiao, L. Li, Q. Zhao, T. Yang, J. Zuo and W. Huang, Dalton Trans., 2013, 42, 5718 RSC;
(c) Y.-N. Gong, L. Jiang and T.-B. Lu, Chem. Commun., 2013, 49, 11113 RSC.
-
(a) S. Pramanik, C. Zheng, X. Zhang, T. J. Emge and J. Li, J. Am. Chem. Soc., 2011, 133, 4153 CrossRef CAS PubMed;
(b) P. Wu, J. Wang, C. He, X. Zhang, Y. Wang, T. Liu and C. Duan, Adv. Funct. Mater., 2012, 22, 1698 CrossRef CAS;
(c) D. Ma, B. Li, X. Zhou, Q. Zhou, K. Liu, G. Zeng, G. Li, Z. Shi and S. Feng, Chem. Commun., 2013, 49, 8964 RSC.
-
(a) G. Aragay, J. Pons and A. Merkoci, Chem. Rev., 2011, 111, 3433 CrossRef CAS PubMed;
(b) D. T. Quang and J. S. Kim, Chem. Rev., 2010, 110, 6280 CrossRef CAS PubMed.
-
(a) J. Rocha, L. D. Carlos, F. A. A. Paz and D. Ananias, Chem. Soc. Rev., 2011, 40, 926 RSC;
(b) Y. J. Cui, G. D. Qian and B. L. Chen, J. Am. Chem. Soc., 2012, 134, 3979 CrossRef CAS PubMed;
(c) J. J. Qian, Q. P. Li, L. F. Liang, Y. Yang, Z. Cao, P. P. Yu, S. M. Huang and M. C. Hong, Chem. Commun., 2016 10.1039/c5cc10359b.
-
(a) S. I. Weissman, J. Chem. Phys., 1942, 10, 214 CrossRef CAS;
(b) F. J. Steemers, W. Verboom, D. N. Reinhoudt, E. B. van der Tol and J. W. Verhoeven, J. Am. Chem. Soc., 1995, 117, 9408 CrossRef CAS.
-
(a) A.-J. Lan, K.-H. Li, H.-H. Wu, D. H. Olson, T. J. Emge, W. Ki, M.-C. Hong and J. Li, Angew. Chem., Int. Ed., 2009, 48, 2334 CrossRef CAS PubMed;
(b) W. S. Liu, T. Q. Jiao, Y. Z. Li, Q. Z. Liu, M. Y. Tan, H. Wang and L. F. Wang, J. Am. Chem. Soc., 2004, 126, 2280 CrossRef CAS PubMed;
(c) B. L. Chen, L. B. Wang, Y. Q. Xiao, F. R. Fronczek, M. Xue, Y. J. Cui and G. D. Qian, Angew. Chem., Int. Ed., 2009, 48, 500 CrossRef CAS PubMed;
(d) B. L. Chen, Y. Yang, F. Zapata, G. N. Lin, G. D. Qian and E. B. Lobkovsky, Adv. Mater., 2007, 19, 1693 CrossRef CAS;
(e) A. K. Chaudhari, S. S. Nagarkar, B. Joarder and S. K. Ghosh, Cryst. Growth Des., 2013, 13, 3716 CrossRef CAS;
(f) S. N. Zhao, L. J. Li, X. Z. Song, M. Zhu, Z. M. Hao, X. Meng, L. L. Wu, J. Feng, S. Y. Song, C. Wang and H. J. Zhang, Adv. Funct. Mater., 2015, 25, 1463 CrossRef CAS;
(g) X. Z. Song, S. Y. Song, S. N. Zhao, Z. M. Hao, M. Zhu, X. Meng, L. L. Wu and H. J. Zhang, Adv. Funct. Mater., 2014, 24, 4034 CrossRef CAS.
-
(a) Z. H. Xiang, C. Q. Fang, S. H. Leng and D. P. Cao, J. Mater. Chem. A, 2014, 2, 7662 RSC;
(b) I. Spanopoulos, P. Xydias, C. D. Malliakas and P. N. Trikalitis, Inorg. Chem., 2013, 52, 855 CrossRef CAS PubMed;
(c) K. L. Wong, G. L. Law, Y. Y. Yang and W. T. Wong, Adv. Mater., 2006, 18, 1051 CrossRef CAS;
(d) Z. M. Hao, X. Z. Song, M. Zhu, X. Meng, S. N. Zhao, S. Q. Su, W. T. Yang, S. Y. Song and H. J. Zhang, J. Mater. Chem. A, 2013, 1, 11043 RSC;
(e) S. Seth, G. Savitha and J. N. Moorthy, Inorg. Chem., 2015, 54, 6829 CrossRef CAS PubMed;
(f) A. Burgun, C. J. Doonan and C. J. Sumby, Aust. J. Chem., 2013, 66, 409 CrossRef CAS.
-
(a) A. G. Wong-Foy, O. Lebel and A. J. Matzger, J. Am. Chem. Soc., 2007, 129, 15740 CrossRef CAS PubMed;
(b) L. Feng, Z. X. Chen, T. B. Liao, P. Li, Y. Jia, X. F. Liu, Y. T. Yang and Y. M. Zhou, Cryst. Growth Des., 2009, 9, 1505 CrossRef CAS;
(c) Z.-J. Lin, B. Xu, T.-F. Liu, M.-N. Cao, J. Lu and R. Cao, Eur. J. Inorg. Chem., 2010, 24, 3842 CrossRef.
-
(a) J. Xu, L. B. Sun, H. Z. Xing, Z. Q. Liang, J. H. Yu and R. R. Xu, Inorg. Chem. Commun., 2011, 14, 978 CrossRef CAS;
(b) C. Q. Zhang, L. B. Sun, Y. Yan, J. Y. Li, X. W. Song, Y. L. Liu and Z. Q. Liang, Dalton Trans., 2015, 44, 230 RSC;
(c) L. B. Sun, Y. Li, Z. Q. Liang, J. H. Yu and R. R. Xu, Dalton Trans., 2012, 41, 12790 RSC.
-
(a) C. Q. Zhang, Y. Yan, Q. H. Pan, L. B. Sun, H. M. He, Y. L. Liu, Z. Q. Liang and J. Y. Li, Dalton Trans., 2015, 44, 13340 RSC;
(b) L. B. Sun, H. Z. Xing, J. Xu, Z. Q. Liang, J. H. Yu and R. R. Xu, Dalton Trans., 2013, 42, 5508 RSC.
-
(a) E. V. Alexandrov, V. A. Blatov, A. V. Kochetkov and D. M. Proserpio, CrystEngComm, 2011, 13, 3947 RSC;
(b) V. A. Blatov, Struct. Chem., 2012, 23, 955 CrossRef CAS.
- M. E. C. P. Bruker, SMART APEX User's Manual., AXS Inc., WI 53711-5373, USA, 2000 Search PubMed.
- G. M. Sheldrick, Acta Crystallogr., Sect. A: Found. Crystallogr., 2008, 64, 112 CrossRef CAS PubMed.
-
(a) A. L. Spek, J. Appl. Crystallogr., 2003, 36, 7 CrossRef CAS;
(b) A. L. Spek, Acta Crystallogr., Sect. D: Biol. Crystallogr., 2009, 65, 148 CrossRef CAS PubMed.
- Z. P. Demko and K. B. Sharpless, Angew. Chem., Int. Ed., 2002, 41, 2110 CrossRef CAS PubMed.
- I. Aujard, J.-P. Baltaze, J.-B. Baudin, E. Cogné, F. Ferrage, L. Jullien, É. Perez, V. Prévost, L. M. Qian and O. Ruel, J. Am. Chem. Soc., 2001, 123, 8177 CrossRef CAS PubMed.
-
(a) X.-Y. Li, Z.-J. Lin, Y.-Y. Yang and R. Cao, CrystEngComm, 2014, 16, 6425 RSC;
(b) L. L. Zhang, C. Y. Lu, S. P. Chen, F. S. Yu, X. Li, J. T. Tan and X. W. Yang, Inorg. Chem. Commun., 2011, 14, 143 CrossRef CAS.
-
(a) Y. Zhou, H. H. Chen and B. Yan, J. Mater. Chem. A, 2014, 2, 13691 RSC;
(b) Q. R. Wu, J. J. Wang, H. M. Hu, Y. Q. Shangguan, F. Fu, M. L. Yang, F. X. Dong and G. L. Xue, Inorg. Chem. Commun., 2011, 14, 484 CrossRef CAS.
-
(a) R. M. Wen, S. D. Han, G. J. Ren, Z. Chang, Y. W. Li and X. H. Bu, Dalton Trans., 2015, 44, 10914 RSC;
(b) Y. T. Liang, G. P. Yang, B. Liu, Y. T. Yan, Z. P. Xi and Y. Y. Wang, Dalton Trans., 2015, 44, 13325 RSC;
(c) X. L. Zhao, D. Tian, Q. Gao, H. W. Sun, J. Xu and X. H. Bu, Dalton Trans., 2016, 45, 1040 RSC.
-
(a) S. S. Nagarkar, A. V. Desai and S. K. Ghosh, Chem. Commun., 2014, 50, 8915 RSC;
(b) S. S. Nagarkar, B. Joarder, A. K. Chaudhari, S. Mukherjee and S. K. Ghosh, Angew. Chem., Int. Ed., 2013, 125, 2953 CrossRef;
(c) J.-D. Xiao, L.-G. Qiu, F. Ke, Y.-P. Yuan, G.-S. Xu, Y.-M. Wang and X. Jiang, J. Mater. Chem. A, 2013, 1, 8745 RSC.
Footnote |
† Electronic supplementary information (ESI) available: X-ray crystallographic data for 1–3 in CIF format. The crystal data and structure refinement, the selected bond lengths and angles for 1–3. The asymmetric unit, PXRD patterns, TGA, and IR spectra of 1–3. Luminescent titrations of different volume of NAC of 1′ in ethanol suspensions. CCDC 1438473–1438475 for 3, 2 and 1, respectively. For ESI and crystallographic data in CIF or other electronic format see DOI: 10.1039/c6ra06303a |
|
This journal is © The Royal Society of Chemistry 2016 |
Click here to see how this site uses Cookies. View our privacy policy here.